Female-biased upregulation of insulin pathway activity mediates the sex difference in Drosophila body size plasticity
Abstract
Nutrient-dependent body size plasticity differs between the sexes in most species, including mammals. Previous work in Drosophila showed that body size plasticity was higher in females, yet the mechanisms underlying increased female body size plasticity remain unclear. Here, we discover that a protein-rich diet augments body size in females and not males because of a female-biased increase in activity of the conserved insulin/insulin-like growth factor signaling pathway (IIS). This sex-biased upregulation of IIS activity was triggered by a diet-induced increase in stunted mRNA in females, and required Drosophila insulin-like peptide 2, illuminating new sex-specific roles for these genes. Importantly, we show that sex determination gene transformer promotes the diet-induced increase in stunted mRNA via transcriptional coactivator Spargel to regulate the male-female difference in body size plasticity. Together, these findings provide vital insight into conserved mechanisms underlying the sex difference in nutrient-dependent body size plasticity.
Introduction
In insects, the rate of growth during development is influenced by environmental factors such as nutrient availability (Boulan et al., 2015; Edgar, 2006; Hietakangas and Cohen, 2009; Nijhout, 2003; Nijhout et al., 2014). When nutrients are abundant, the growth rate is high and body size is large (Beadle et al., 1938; Edgar, 2006; Mirth and Shingleton, 2012; Nijhout, 2003; Robertson, 1963). When nutrients are scarce, the growth rate is lower and body size is smaller (Beadle et al., 1938; Edgar, 2006; Mirth and Riddiford, 2007; Mirth and Shingleton, 2012; Nijhout, 2003; Robertson, 1963). This ability of an organism or genotype to adjust its body size in line with nutrient availability is a form of phenotypic plasticity (Agrawal, 2001; Garland, 2006). While the capacity of individuals to display nutrient-dependent changes to body size depends on many factors, one important factor that affects phenotypic plasticity is whether an animal is male or female (Stillwell et al., 2010; Teder and Tammaru, 2005). For example, in Drosophila the magnitude of changes to wing cell size and cell number in a nutrient-poor diet were larger in females compared with males (Alpatov, 1930). Similarly, the magnitude of protein- and carbohydrate-induced changes to several morphological traits was larger in female flies (Shingleton et al., 2017). While these studies clearly establish a sex difference in nutrient-dependent phenotypic plasticity, the genetic and molecular mechanisms underlying this increased trait size plasticity in females remain unclear.
Clues into potential mechanisms underlying the increased nutrient-dependent phenotypic plasticity in female flies have emerged from over 20 years of studies on nutrient-dependent growth in Drosophila (Andersen et al., 2013; Boulan et al., 2015; Edgar, 2006; Koyama and Mirth, 2018; Mirth and Piper, 2017). In particular, these studies have identified the conserved insulin/insulin-like growth factor signaling pathway (IIS) as a key regulator of nutrient-dependent growth in Drosophila (Böhni et al., 1999; Britton et al., 2002; Chen et al., 1996; Fernandez et al., 1995; Grewal, 2009; Teleman, 2010). In nutrient-rich conditions, insulin-producing cells (IPCs) in the larval brain release Drosophila insulin-like peptides (Dilps) into the circulation (Brogiolo et al., 2001; Géminard et al., 2009; Ikeya et al., 2002; Rulifson et al., 2002). These Dilps bind the Insulin-like Receptor (InR; FBgn0283499) on target cells to induce receptor autophosphorylation and recruitment of adapter proteins (Almudi et al., 2013; Böhni et al., 1999; Chen et al., 1996; Poltilove et al., 2000; Werz et al., 2009). These adapter proteins enable the recruitment of the regulatory and catalytic subunits of the Drosophila homolog of phosphatidylinositol 3-kinase (Pi3K21B; FBgn0020622 and Pi3K92E; FBgn0015279, respectively), which catalyze the production of phosphatidylinositol (3,4,5)-trisphosphate (PIP3) from phosphatidylinositol (4,5)-bisphosphate (PIP2) (Leevers et al., 1996). Increased plasma membrane PIP3 recruits and activates signaling proteins such as phosphoinositide-dependent kinase 1 (Pdk1; FBgn0020386) and Akt (Akt; FBgn0010379), which influence diverse cellular processes to enhance cell, tissue, and organismal size (Cho et al., 2001; Grewal, 2009; Rintelen et al., 2001; Verdu et al., 1999).
In contrast, when nutrients are scarce, Dilp release from the IPCs is reduced (Géminard et al., 2009), and plasma membrane Pi3K recruitment, PIP3 levels, and Pdk1- and Akt-dependent signaling are all reduced (Britton et al., 2002; Nowak et al., 2013). Together, these changes diminish cell, tissue, and organismal size (Arquier et al., 2008; Britton et al., 2002; Géminard et al., 2009; Honegger et al., 2008; Okamoto et al., 2013; Rulifson et al., 2002; Zhang et al., 2009). Indeed, the potent growth-promoting ability of IIS activation is shown by the fact that increased IIS activity augments body size (Arquier et al., 2008; Goberdhan et al., 1999; Honegger et al., 2008; Ikeya et al., 2002; Nowak et al., 2013; Okamoto et al., 2013; Oldham et al., 2002), whereas reduced IIS activity limits cell, organ, and body size (Böhni et al., 1999; Brogiolo et al., 2001; Chen et al., 1996; Colombani et al., 2003; Gao et al., 2000; Grönke et al., 2010; Leevers et al., 1996; Murillo-Maldonado et al., 2011; Rulifson et al., 2002; Weinkove et al., 1999; Zhang et al., 2009). Because increased IIS activity bypasses the reduction in cell size in low-nutrient conditions (Britton et al., 2002; Géminard et al., 2009; Nowak et al., 2013), and mutations that blunt IIS pathway activity reduce size in nutrient-rich contexts (Böhni et al., 1999; Brogiolo et al., 2001; Chen et al., 1996; Leevers et al., 1996), Drosophila studies have established IIS as one key pathway that promotes organismal growth downstream of nutrient input. While this highlights the impact of Drosophila on our knowledge of how IIS couples nutrient input with growth, it is important to note that most studies used a mixed-sex population of larvae. Given that cell and body size differ significantly between male and female flies (Alpatov, 1930; Brown and King, 1961; Okamoto et al., 2013; Partridge et al., 1994; Rideout et al., 2015; Sawala and Gould, 2017; Testa et al., 2013), more knowledge is needed of nutrient-dependent changes to body size and IIS activity in each sex.
Recent studies have begun to make progress in this area by studying IIS regulation and function in both sexes in a single dietary context (reviewed in Millington and Rideout, 2018). For example, in late third instar larvae, there are sex differences in dilp mRNA levels, IIS activity, and Drosophila insulin-like peptide 2 (Dilp2; FBgn0036046) secretion from the IPCs (Rideout et al., 2015; McDonald et al., 2020). Similarly, transcriptomic studies have detected male-female differences in mRNA levels of genes associated with IIS function (Mathews et al., 2017; Rideout et al., 2015), and revealed links between IIS and the sex determination hierarchy gene regulatory network (Castellanos et al., 2013; Chang et al., 2011; Clough et al., 2014; Fear et al., 2015; Garner et al., 2018; Goldman and Arbeitman, 2007). As increasing evidence of sex-specific IIS regulation accumulates, several reports reveal sex-limited and sex-biased phenotypic effects caused by changes to IIS function. Changes to IIS activity in larvae show sex-biased effects on growth and final body size (Grönke et al., 2010; Rideout et al., 2015; Shingleton et al., 2005; Testa et al., 2013; Millington et al., 2021), and there are widespread sex-specific and sex-biased changes to gene expression in adult flies with altered diet and IIS activity (Camus et al., 2019; Graze et al., 2018). Further, sex differences exist in how changes to diet and IIS activity affect life span (Bjedov et al., 2010; Clancy, 2001; Giannakou et al., 2004; Grönke et al., 2010; Regan et al., 2016; Tatar et al., 2001; Woodling et al., 2020; Wu et al., 2020). Together, these studies illuminate the utility of Drosophila in revealing sex-specific IIS regulation and describing the physiological impact of this regulation. Yet, more studies are needed to discover the molecular mechanisms underlying sex-specific IIS regulation, and to extend these studies beyond a single nutritional context.
Additional insights into male-female differences in the regulation of cell, tissue, and body size arise from studies on sex determination genes. In Drosophila, sex is determined by the number of X chromosomes. In XX females, a functional splicing factor called Sex-lethal (Sxl; FBgn0264270) is produced (Bell et al., 1988; Bridges, 1921; Cline, 1978; Salz and Erickson, 2010). Sxl-dependent splicing of transformer (tra; FBgn0003741) pre-mRNA allows a functional Tra protein to be produced in females (Belote et al., 1989; Boggs et al., 1987; Inoue et al., 1990; Sosnowski et al., 1989). In XY males, the lack of a functional Sxl protein causes the default splicing of tra pre-mRNA, and no functional Tra protein is produced in males (Cline and Meyer, 1996; Salz and Erickson, 2010; Belote et al., 1989; Boggs et al., 1987; Inoue et al., 1990; Sosnowski et al., 1989). The presence of functional Sxl and Tra proteins in females account for most aspects of female sexual development, behavior, and physiology (Anand et al., 2001; Billeter et al., 2006; Brown and King, 1961; Camara et al., 2008; Christiansen et al., 2002; Clough et al., 2014; Dauwalder, 2011; Demir and Dickson, 2005; Goodwin et al., 2000; Hoshijima et al., 1991; Hudry et al., 2016; Hudry et al., 2019; Ito et al., 1996; Millington and Rideout, 2018; Neville et al., 2014; Nojima et al., 2014; Pavlou et al., 2016; Pomatto et al., 2017; Regan et al., 2016; Rezával et al., 2014; Rezával et al., 2016; Rideout et al., 2010; Ryner et al., 1996; Sturtevant, 1945; von Philipsborn et al., 2014). Recently, new roles for Sxl and Tra in regulating body size were also described. While female flies are normally larger than males, females lacking neuronal Sxl were smaller than control females, and not different in size from males (Sawala and Gould, 2017). Similarly, females lacking a functional Tra protein were smaller than control females; however, these tra mutant females were still larger than males (Brown and King, 1961; Mathews et al., 2017; Rideout et al., 2015). Together, these studies indicate that Tra and Sxl are required to promote a larger body size in females; however, much remains to be discovered about the mechanisms by which Sxl and Tra impact body size. Moreover, which sex determination genes contribute to the male-female difference in diet-induced trait size plasticity remains unknown, as studies on sex determination genes used a single diet.
In the present study, we aimed to improve knowledge of the genetic and molecular mechanisms that contribute to male-female differences in nutrient-dependent phenotypic plasticity in Drosophila. Our detailed examination of body size revealed increased phenotypic plasticity in females in response to a protein-rich diet, in line with studies on plasticity in other traits (Shingleton et al., 2017). We discovered that a female-biased upregulation of IIS activity was responsible for the larger body size of females raised on a protein-rich diet. Mechanistically, we show that the nutrient-dependent upregulation of stunted (sun; FBgn0014391) mRNA levels by transcriptional coactivator Spargel (Srl; FBgn0037248) in females triggers the diet-induced increase in IIS activity, as females with reduced sun do not augment IIS activity or body size in a protein-rich diet. Importantly, we show that sex determination gene tra is required for the nutrient-dependent increase in sun mRNA, IIS activity, and phenotypic plasticity in females, and that Srl represents a key link between Tra and regulation of sun mRNA levels. In males, ectopic Tra expression confers nutrient-dependent body size plasticity via Srl-mediated regulation of sun mRNA levels and IIS activity. Together, these results provide new insight into the molecular mechanisms that govern male-female differences in body size plasticity, and identify a previously unrecognized role for sex determination gene tra in regulating nutrient-dependent phenotypic plasticity.
Results
High levels of dietary protein are required for increased nutrient-dependent body size plasticity in females
Previous studies identified a sex difference in nutrient-dependent plasticity in several morphological traits (Shingleton et al., 2017; Stillwell et al., 2010; Teder and Tammaru, 2005). To determine whether sex differences in nutrient-dependent body size plasticity exist in Drosophila, we measured pupal volume, an established readout for Drosophila body size (Delanoue et al., 2010), in white1118 (w; FBgn0003996) males and females reared on diets of varying nutrient quantity. We found that pupal volume in w1118 female larvae raised on the two-acid diet (1X) (Lewis, 1960) was significantly larger than genotype-matched females raised on a diet with half the nutrient quantity (0.5X) (Figure 1—figure supplement 1A). In w1118 males, pupal volume was also significantly larger in larvae raised on the 1X diet compared with the 0.5X diet (Figure 1—figure supplement 1A). No significant sex-by-diet interaction was detected using a two-way analysis of variance (ANOVA) (sex:diet interaction p=0.7048; Supplementary file 1), suggesting that nutrient-dependent body size plasticity was not different between the sexes in this context. We next compared pupal volume in w1118 males and females raised on the 1X diet with larvae cultured on a diet with twice the nutrient content (2X). Pupal volume in w1118 females was significantly larger in larvae raised on the 2X diet compared with larvae cultured on the 1X diet (Figure 1—figure supplement 1A). In w1118 males, the magnitude of the nutrient-dependent increase in pupal volume was smaller compared with female larvae (Figure 1—figure supplement 1A; sex:diet interaction p<0.0001; Supplementary file 1). This suggests that in nutrient-rich conditions, there is a sex difference in phenotypic plasticity, where nutrient-dependent body size plasticity is higher in females. To represent the normal body size responses of each sex to nutrient quantity, we plotted reaction norms for pupal volume in w1118 males and females raised on different diets (Figure 1—figure supplement 1B). The body size response to increased nutrient quantity between 0.5X and 1X was not different between the sexes (Figure 1—figure supplement 1B); however, the body size response to increased nutrient quantity between 1X and 2X was larger in females than in males (Figure 1—figure supplement 1B). Importantly, these findings were not specific to pupal volume, as we reproduced our findings using adult weight as an additional readout for body size (Figure 1A,B). Thus, our findings demonstrate that while phenotypic plasticity is similar between the sexes in some nutritional contexts, body size plasticity is higher in females than in males in a nutrient-rich environment.
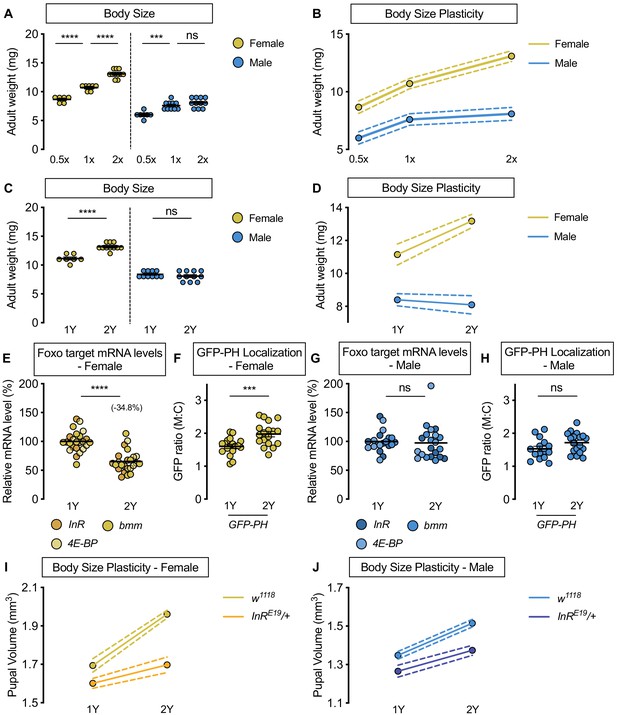
Upregulation of IIS activity is required for increased nutrient-dependent body size plasticity in females in a protein-rich diet.
(A) Adult weight was significantly higher in w1118 males and females cultured on 1X compared with flies raised on 0.5X (p<0.0001 for both sexes; two-way ANOVA followed by Tukey HSD test). The magnitude of this increase in adult weight was the same in both sexes (sex:diet interaction p=0.3197; two-way ANOVA followed by Tukey HSD test). Adult weight was significantly higher in w1118 females raised on 2X compared to flies cultured on 1X; however, male adult weight was not significantly increased (p<0.0001 and p=0.4015, respectively; two-way ANOVA followed by Tukey HSD test), where the diet-dependent increase in adult weight was higher in females (sex:diet interaction p=0.0003; two-way ANOVA followed by Tukey HSD test). (B) Reaction norms for adult weight in response to changes in nutrient quantity in w1118 females and males, plotted using the data presented in panel A. n = 6–11 groups of 10 flies. (C) Adult weight was significantly higher in females cultured on 2Y compared with flies raised on 1Y; however, male adult weight was not significantly higher in flies raised on 2Y compared with males cultured on 1Y (p<0.0001 and p=0.7199, respectively; two-way ANOVA followed by Tukey HSD test, sex:diet interaction p<0.0001). (D) Reaction norms for adult weight in w1118 females and males reared on either 1Y or 2Y, plotted using data from panel C. n = 7–11 groups of 10 flies. (E) In females, mRNA levels of Foxo targets (insulin receptor (InR), brummer (bmm), and eukaryotic initiation factor 4E-binding protein (4E-BP)), were significantly lower in larvae raised on a protein-rich diet (2Y) compared with larvae raised on a diet containing half the protein content (1Y) (p<0.0001; Student’s t test). n = 8 biological replicates. (F) Quantification of the ratio between cell surface membrane-associated green fluorescent protein (GFP) and cytoplasmic GFP (GFP ratio [M:C]) in a dissected fat body of female larvae from the GFP-PH strain. The ratio was significantly higher in female larvae cultured on 2Y compared with larvae raised on 1Y (p=0.001; Student’s t test). n = 18 biological replicates. (G) In males, there was no significant difference in mRNA levels of Foxo targets between larvae raised on 2Y compared with larvae cultured on 1Y (p=0.7323; Student’s t test). n = 6–7 biological replicates. (H) In males, the M:C ratio for GFP-PH was not significantly different between males cultured on 2Y compared with larvae raised on 1Y (p=0.0892; Student’s t test). n = 15–18 biological replicates. (I) Pupal volume was significantly higher in both w1118 females and InRE19/+ females reared on 2Y compared with genotype-matched females cultured on 1Y (p<0.0001 for both genotypes; two-way ANOVA followed by Tukey HSD test); however, the magnitude of the nutrient-dependent increase in pupal volume was lower in InRE19/+ females (genotype:diet interaction p<0.0001; two-way ANOVA followed by Tukey HSD test). n = 58–77 pupae. (J) Pupal volume was significantly higher in both w1118 males and InRE19/+ males reared on 2Y compared with genotype-matched males cultured on 1Y (p<0.0001 for both genotypes; two-way ANOVA followed by Tukey HSD test). While we observed a sex:diet interaction in the w1118 control genotype, there was no sex:diet interaction in the InRE19/+ genotype (p<0.0001 and p=0.7104, respectively; two-way ANOVA followed by Tukey HSD test). n = 47–76 pupae. For body size plasticity graphs, filled circles indicate mean body size, and dashed lines indicate 95% confidence interval. *** indicates p<0.001, **** indicates p<0.0001; ns indicates not significant; error bars indicate SEM.
To narrow down macronutrients that account for the increased body size plasticity in females, we changed individual food ingredients and measured body size in w1118 males and females. We first altered dietary yeast, as previous studies show that yeast is a key source of protein and an important determinant of larval growth (Britton et al., 2002; Géminard et al., 2009; Robertson, 1963). In w1118 females raised on a diet with yeast content that corresponds to the amount in the 2X diet (2Y diet), pupal volume was significantly larger than in females raised on a diet containing half the yeast content (1Y) (Figure 1—figure supplement 1C). It is important to note that the yeast and calorie content of the 1Y diet was within the range of standard diets used in many larval growth studies (22.65 g/L vs. 21–46 g/L and 586 calories/L vs 459–760 calories/L, respectively) (Ghosh et al., 2014; Koyama and Mirth, 2016; Marshall et al., 2012; Sawala and Gould, 2017), and therefore does not represent a nutrient-restricted diet. In w1118 males, the magnitude of the nutrient-dependent increase in pupal volume was smaller than in females (Figure 1—figure supplement 1C; sex:diet interaction p=0.0001; Supplementary file 1), suggesting that nutrient-dependent body size plasticity was higher in females in a yeast-rich context. Indeed, when we plotted reaction norms for pupal volume in both sexes, the magnitude of the yeast-dependent change in pupal volume (Figure 1—figure supplement 1D) and adult weight (Figure 1C,D) was larger in females than in males. This sex difference in phenotypic plasticity in a yeast-rich context was reproduced in Canton-S (CS), a wild-type strain (Figure 1—figure supplement 2A,B), and using wing length as an additional measure of size (Figure 1—figure supplement 3A). Thus, our findings indicate that the male-female difference in nutrient-dependent body size plasticity persists across multiple genetic backgrounds, and confirms that body size is a robust trait to monitor nutrient-dependent phenotypic plasticity.
Given the sex difference in body size plasticity in response to altered yeast content, we hypothesized that yeast may trigger increased nutrient-dependent body size plasticity in females. To test this, we raised larvae on diets with altered sugar (Figure 1—figure supplement 4A) or calorie content (Figure 1—figure supplement 4B). Because we observed no sex:diet interaction for either manipulation (sex:diet interaction p=0.6536 and p=0.3698, respectively; Supplementary file 1), this suggests dietary yeast mediates the sex difference in nutrient-dependent body size plasticity. To test whether protein is the macronutrient in yeast that enables sex-specific phenotypic plasticity, we pharmacologically limited protein breakdown by culturing larvae on the 2Y diet supplemented with either a broad-spectrum protease inhibitor (protease inhibitor cocktail; PIC) or a serine protease-specific inhibitor (4-(2-aminoethyl)benzenesulfonyl fluoride hydrochloride; AEBSF). Previous studies suggest that these inhibitors are specific, as the growth-inhibitory effect of these protease inhibitors was buffered by feeding larvae with bacteria that enhance intestinal protease mRNA levels and gut proteolytic activity (Erkosar et al., 2015). While we found a significant body size reduction in both sexes treated with protease inhibitors (Figure 1—figure supplement 5A,B), in line with previous studies (Erkosar et al., 2015), the magnitude of the inhibitor-induced decrease in pupal volume was larger in female larvae than in males (sex:treatment interaction p=0.0029 [PIC] and p<0.0001 [AEBSF]; Supplementary file 1). This indicates that yeast-derived dietary protein is the macronutrient that augments nutrient-dependent body size plasticity in females. While two potential explanations for the male-female difference in body size plasticity are a sex difference in food intake or length of the growth period, we found no differences in either phenotype between w1118 male and female larvae cultured on 1Y or 2Y (Figure 1—figure supplement 6A–C). Moreover, the larger body size of female larvae does not explain their increased nutrient-dependent body size plasticity, as a genetic manipulation that augments male body size did not enhance phenotypic plasticity (Figure 1—figure supplement 7A,B). Taken together, our data reveals female larvae have enhanced body size plasticity in a nutrient-rich context, and identifies abundant dietary protein as a prerequisite for females to maximize body size.
The nutrient-dependent upregulation of IIS activity in females is required to achieve a larger body size in a protein-rich context
In a mixed-sex population of Drosophila larvae, IIS activity is positively regulated by nutrient availability to promote growth (Böhni et al., 1999; Britton et al., 2002; Chen et al., 1996; Fernandez et al., 1995; Grewal, 2009; Teleman, 2010). We therefore examined nutrient-dependent changes to IIS activity in larvae raised on 1Y and 2Y (Figure 1E–H). Previous studies show that high levels of IIS activity repress mRNA levels of several genes via transcription factor Forkhead box, sub-group O (Foxo; FBgn0038197) (Alic et al., 2011; Jünger et al., 2003; Kang et al., 2017; Puig and Tjian, 2005; Zinke et al., 2002). We therefore assessed mRNA levels of known Foxo target genes InR, brummer (bmm, FBgn0036449), and eukaryotic initiation factor 4E-binding protein (4E-BP, FBgn0261560) together to quantify IIS activity in each sex and dietary context, an established approach to analyze coregulated genes (Blaschke et al., 2013; Hudry et al., 2019). In w1118 females, mRNA levels of Foxo target genes were significantly lower in larvae reared on 2Y than in larvae raised on 1Y (Figure 1E). This suggests IIS activity is significantly higher in females raised on 2Y than in females cultured on 1Y. To confirm this, we used the localization of a ubiquitously-expressed green fluorescent protein (GFP) fused to a pleckstrin homology (PH) domain (GFP-PH) as an additional readout of IIS activity. Because high levels of IIS activity raise plasma membrane PIP3, and PH domains bind specifically to PIP3, larvae with elevated IIS activity show increased membrane localization of GFP-PH (Britton et al., 2002). We observed a significantly higher membrane localization of GFP-PH in females cultured on 2Y than in female larvae raised on 1Y (Figure 1F). Together with increased Foxo target gene repression in 2Y, this GFP-PH data indicates that females reared on 2Y have higher IIS activity than females cultured on 1Y. In males, the magnitude of the nutrient-dependent change in Foxo target genes was smaller than in females (Figure 1G), as we detected a significant sex:diet interaction for Foxo target genes (p=0.0007; Supplementary file 1). Indeed, there was no significant increase in GFP-PH membrane localization between males raised on 2Y and males reared on 1Y (Figure 1H). Taken together, these results reveal a previously unrecognized female-biased upregulation of IIS activity in a protein-rich context.
To determine whether increased IIS activity is required in females for the ability to maximize body size on a protein-rich diet, we measured pupal volume in larvae heterozygous for a hypomorphic mutation in the InR gene (InRE19/+) that were raised in either 1Y or 2Y. Previous studies have shown that while overall growth is largely normal in InRE19/+ heterozygous animals, growth that requires high levels of IIS activity is blunted (Chen et al., 1996; Rideout et al., 2012; Rideout et al., 2015). In w1118 control females, larvae cultured on 2Y were significantly larger than larvae raised on 1Y (Figure 1I); however, the magnitude of this protein-dependent increase in pupal volume was smaller in InRE19/+ females (Figure 1I; genotype:diet interaction p<0.0001; Supplementary file 1). This suggests that nutrient-dependent body size plasticity was reduced in InRE19/+ females. Indeed, while we observed a sex difference in phenotypic plasticity in the w1118 control genotype (sex:diet interaction p<0.0001 Supplementary file 1), the sex difference in nutrient-dependent body size plasticity was abolished in the InRE19/+ genotype (Figure 1I,J; sex:diet interaction p=0.7104; Supplementary file 1). Together, these results indicate that the nutrient-dependent upregulation of IIS activity in females is required for them to achieve a larger body size in a protein-rich context, and that the sex difference in body size plasticity arises from the female-biased upregulation of IIS activity in a protein-rich context.
dilp2 is required for the nutrient-dependent upregulation of IIS activity and a larger body size in females raised on a protein-rich diet.
Previous studies have identified changes to the production and release of Dilps as important mechanisms underlying nutrient-dependent changes to IIS activity and body size (Colombani et al., 2003; Géminard et al., 2009; Zhang et al., 2009). For example, mRNA levels of Drosophila insulin-like peptide 3 (dilp3; FBgn0044050) and Drosophila insulin-like peptide 5 (dilp5; FBgn0044048), but not dilp2, decrease in response to nutrient withdrawal (Colombani et al., 2003; Géminard et al., 2009; Ikeya et al., 2002), and the release of Dilps 2, 3, and 5 from the IPCs is altered by changes in nutrient availability (Géminard et al., 2009; Kim and Neufeld, 2015). Levels of Dilp2 also fluctuate during larval development (Slaidina et al., 2009). Interestingly, a recent study suggests that late third-instar female larvae have increased Dilp2 secretion compared with age-matched males when the larvae were raised in a diet equivalent to 2Y (Rideout et al., 2015). Given that Dilp2 is an important growth-promoting Dilp (Grönke et al., 2010; Ikeya et al., 2002), we tested whether dilp2 was required in females for the nutrient-dependent upregulation of IIS activity. In control w1118 females, mRNA levels of Foxo target genes were significantly lower in larvae raised on 2Y than in larvae reared on 1Y (Figure 2A), suggesting a nutrient-dependent increase in IIS activity. In contrast, mRNA levels of Foxo target genes were not significantly lower in dilp2 mutant female larvae raised on 2Y compared with genotype-matched females cultured on 1Y (Figure 2A), suggesting that loss of dilp2 in females eliminated the nutrient-dependent increase in IIS activity. The magnitude of the nutrient-dependent decrease in Foxo target gene expression was smaller in w1118 males compared with w1118 females (Figure 2B, sex:diet interaction p=0.0511; Supplementary file 1), but not in dilp2 mutant males compared with genotype-matched females (sex:diet interaction p=0.6754; Supplementary file 1). This indicates that dilp2 loss blocks the female-biased upregulation of IIS activity in a protein-rich diet.
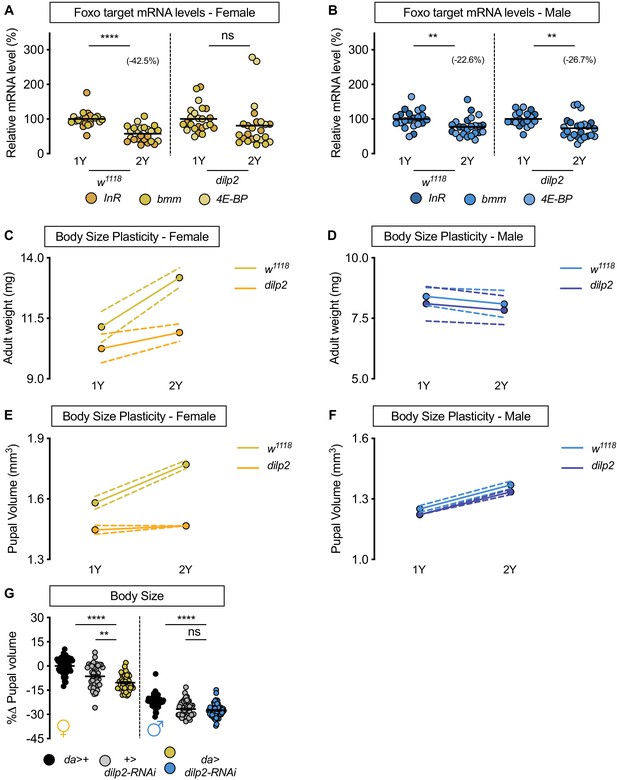
Drosophila insulin-like peptide 2 is required for the nutrient-dependent upregulation of insulin pathway activity and increased female body size plasticity.
(A) In control w1118 females, mRNA levels of Foxo targets (insulin receptor (InR), brummer (bmm), and eukaryotic initiation factor 4E-binding protein (4E-BP)), were significantly lower in larvae cultured on a protein-rich diet (2Y) compared with larvae raised on a diet containing half the protein (1Y) (p<0.0001; Student’s t test). In dilp2 mutant females, there was no significant difference in mRNA levels of Foxo targets in larvae cultured on 2Y compared with larvae raised on 1Y (p=0.2231 Student’s t test). n = 8 biological replicates. (B) In control w1118 and dilp2 mutant males, mRNA levels of Foxo targets were significantly lower in larvae cultured on 2Y compared with larvae raised on 1Y (p=0.0066 and p=0.0023 respectively; Student’s t test). n = 7–8 biological replicates; however, the magnitude of the reduction in Foxo target gene expression in w1118 males was smaller than in genotype-matched females. (C) Adult weight was significantly higher in w1118 females raised on 2Y compared with flies cultured on 1Y (p<0.0001; two-way ANOVA followed by Tukey HSD test); however, adult weight was not significantly different between dilp2 mutant females reared on 2Y versus 1Y (p=0.1263; two-way ANOVA followed by Tukey HSD test). n = 7–11 groups of 10 flies. (D) Adult weight in control w1118 and dilp2 mutant males was not significantly higher in flies reared on 2Y compared with males raised on 1Y (p=0.8366 and p=0.8817, respectively; two-way ANOVA followed by Tukey HSD test). There was a significant sex:diet interaction in the control w1118 genotype (p<0.0001), but not in the dilp2 mutant genotype (p=0.0827; two-way ANOVA followed by Tukey HSD test). n = 10–12 groups of 10 flies. (E) Pupal volume was significantly higher in w1118 females but not in dilp2 mutant females reared on 2Y compared with genotype-matched females cultured on 1Y (p<0.0001 and p=0.6486 respectively; two-way ANOVA followed by Tukey HSD test). The magnitude of the nutrient-dependent increase in pupal volume was higher in w1118 females (genotype:diet interaction p<0.0001; two-way ANOVA followed by Tukey HSD test). n = 74–171 pupae. (F) Pupal volume was significantly higher in w1118 males and dilp2 mutant males reared on 2Y compared with genotype-matched males cultured on 1Y (p<0.0001 for both genotypes; two-way ANOVA followed by Tukey HSD test). The magnitude of the nutrient-dependent increase in pupal volume was not different between genotypes (genotype:diet interaction p=0.6891; two-way ANOVA followed by Tukey HSD test). n = 110–135 pupae. (G) Pupal volume was significantly reduced in females upon RNAi-mediated knockdown of dilp2 in 2Y when compared to both control genotypes (p<0.0001 [da>+], and p=0.002 [+>UAS-dilp2-RNAi], respectively; two-way ANOVA followed by Tukey HSD test), but not in males in 2Y (p<0.0001 [da>+], and 0.9634 [+>UAS-dilp2-RNAi], respectively; two-way ANOVA followed by Tukey HSD test). The magnitude of the effect of RNAi-mediated knockdown of dilp2 on pupal volume was higher in females (sex:genotype interaction p=0.003; two-way ANOVA followed by Tukey HSD test). n = 44–59 pupae. For all body size plasticity graphs, filled circles indicate mean body size, and dashed lines indicate 95% confidence interval. ** indicates p<0.01, **** indicates p<0.0001; ns indicates not significant; error bars indicate SEM.
To determine whether the inability to augment IIS activity on 2Y affects the nutrient-dependent increase in female body size, we measured body size in w1118 and dilp2 mutant larvae cultured on either 1Y or 2Y. In w1118 control females, adult weight was significantly higher in flies cultured on 2Y compared with flies raised on 1Y (Figure 2C); however, this nutrient-dependent increase in adult weight was not observed in dilp2 mutant females (Figure 2C; genotype:diet interaction p=0.0024; Supplementary file 1). In w1118 control males and dilp2 mutant males, there was no significant increase in adult weight in flies raised on 2Y compared with genotype-matched flies cultured on 1Y (Figure 2D; genotype:diet interaction p=0.935; Supplementary file 1). Indeed, in contrast to the sex difference in nutrient-dependent body size plasticity in the w1118 genotype (sex:diet interaction p<0.0001; Supplementary file 1), the sex difference in phenotypic plasticity was abolished in the dilp2 mutant genotype (sex:diet interaction p=0.0827; Supplementary file 1). Importantly, we replicated all these findings using pupal volume (Figure 2E,F), reproduced the female-specific effects of dilp2 loss by globally overexpressing a UAS-dilp2-RNAi transgene (Figure 2G), and show that dilp2 loss does not alter feeding behavior (Figure 2—figure supplement 1A). While we did not determine a sex difference in circulating Dilp2 levels in larvae with an endogenously tagged dilp2 allele due to body size plasticity defects in this strain (Park et al., 2014; Figure 2—figure supplement 2A,B), an experiment that will be important to repeat in future using alternative ways of measuring circulating Dilp2, we show that changes to dilp mRNA levels in males and females lacking dilp2 (Figure 2—figure supplement 3A,B), and nutrient-dependent changes to dilp mRNA levels (Figure 2—figure supplement 4A,B), were similar in both sexes. Together, our data reveals a previously unrecognized female-specific requirement for dilp2 in triggering a nutrient-dependent increase in IIS activity and body size in a protein-rich context.
A nutrient-dependent increase in stunted mRNA levels is required for enhanced IIS activity and a larger body size in females cultured in a protein-rich diet
Nutrient-dependent changes in Dilp secretion from the IPCs, and consequently IIS activity, are mediated by humoral factors that are regulated by dietary nutrients (Britton and Edgar, 1998; Delanoue et al., 2016; Koyama and Mirth, 2016; Rajan and Perrimon, 2012; Rodenfels et al., 2014; Sano et al., 2015). For example, in a mixed-sex population of larvae, dietary protein augments mRNA levels of Growth-blocking peptides 1 and 2 (Gbp1, FBgn0034199; Gbp2, FBgn0034200), CCHamide-2 (CCHa2; FBgn0038147), unpaired 2 (upd2; FBgn0030904), and sun (Delanoue et al., 2016; Koyama and Mirth, 2016; Rajan and Perrimon, 2012; Sano et al., 2015). Increased levels of these humoral factors promote the secretion of IPC-produced Dilps to enhance IIS activity and growth (Delanoue et al., 2016; Koyama and Mirth, 2016; Meschi et al., 2019; Rajan and Perrimon, 2012; Sano et al., 2015). To determine whether any humoral factors contribute to the sex-biased increase in IIS activity in a protein-rich diet, we examined mRNA levels of each factor in larvae of both sexes raised on either 1Y or 2Y. In w1118 females, sun mRNA levels in larvae reared on 2Y were significantly higher than in larvae cultured on 1Y (Figure 3A). In contrast, mRNA levels of Gbp1, Gbp2, CCHa2, and upd2 were not significantly higher in female larvae reared on 2Y compared with 1Y (Figure 3B). Thus, while previous studies have shown that mRNA levels of all humoral factors were severely reduced by a nutrient-restricted diet or nutrient withdrawal (Delanoue et al., 2016; Koyama and Mirth, 2016; Rajan and Perrimon, 2012; Sano et al., 2015), our study suggests that for most factors, augmenting dietary protein beyond a widely used level does not further enhance mRNA levels. In males, there was no significant increase in sun mRNA levels (Figure 3C), or any other humoral factors (Figure 3D), in larvae reared on 2Y compared with 1Y. Thus, there is a previously unrecognized sex difference in the regulation of sun mRNA levels in a protein-rich context, which we confirm leads to a sex difference in circulating Sun levels (Figure 3—figure supplement 1A).
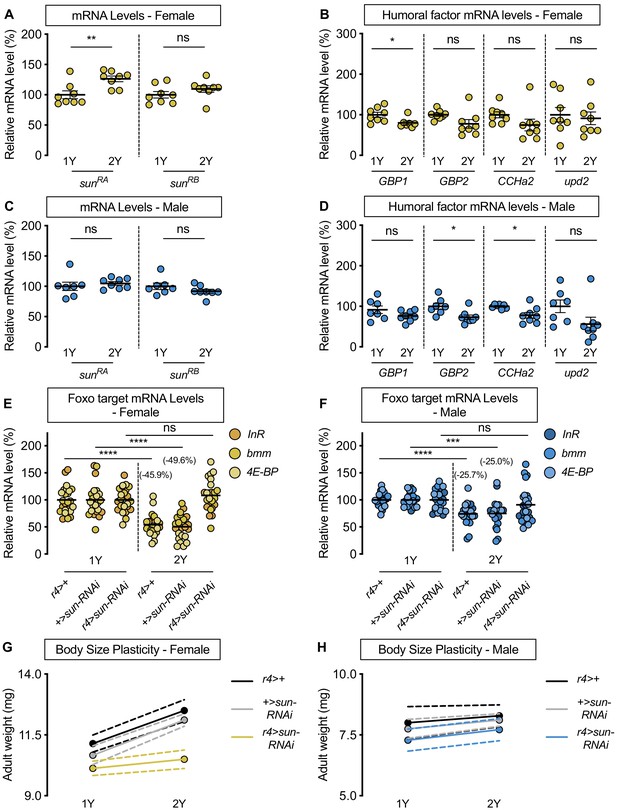
stunted is required for the nutrient-dependent upregulation of insulin pathway activity and increased female body size plasticity.
(A) In females, mRNA levels of stunted (sun)RA, but not sunRB, were significantly higher in larvae cultured on a protein-rich diet (2Y) compared with larvae raised on a diet containing half the protein (1Y) (p=0.0055 and p=0.2327, respectively; Student’s t test). n = 8 biological replicates. (B) mRNA levels of Growth-blocking peptide 1 (Gbp1) were significantly different in females cultured on 2Y compared with females raised in 1Y (p=0.0245; Student’s t test); however, mRNA levels of Growth-blocking peptide 2 (Gbp2), CCHamide-2 (CCHa2), and unpaired 2 (upd2) were not significantly different between female larvae raised on 1Y and 2Y (p=0.0662, 0.1416, and 0.7171, respectively; Student’s t test). n = 7–8 biological replicates. (C) In males, mRNA levels of sunRA and sunRB were not significantly different in larvae raised on 2Y compared with larvae raised on 1Y (p=0.5832 and p=0.2017, respectively; Student’s t test). n = 7–8 biological replicates. (D) Levels of Gbp1 and upd2 were not significantly different between male larvae raised on 2Y compared with larvae reared on 1Y (p=0.1487, and p=0.1686, respectively; Student’s t test); whereas levels of Gbp2 and CCHa2 were significantly different between males raised in 2Y and 1Y (p=0.0214, and p=0.0272, respectively; Student’s t test). n = 7–8 biological replicates. (E) In control r4>+, and +>sun-RNAi females, mRNA levels of Foxo targets (insulin receptor (InR), brummer (bmm), and eukaryotic initiation factor 4E-binding protein (4E-BP)), were significantly lower in larvae cultured on 2Y compared with larvae raised on 1Y (p<0.0001, for both comparisons; Student’s t test). However, in r4>sun-RNAi females, there was no significant difference in Foxo target mRNA levels (p=0.2792; Student’s t test). n = 8 biological replicates. (F) In control r4>+, and +>sun-RNAi males, mRNA levels of Foxo targets were significantly lower in larvae cultured on 2Y compared with larvae raised on 1Y (p<0.0001 and p=0.0001, respectively; Student’s t test). While r4>sun-RNAi males showed no significant difference in Foxo target mRNA levels (p=0.2469; Student’s t test), there was no genotype:diet interaction among males (p=0.1068), suggesting that genotype had no impact on Foxo target genes. Importantly, there was a significant sex:diet interaction for Foxo target mRNA levels in both the r4>+ control (p=0.0166; two-way ANOVA followed by Tukey HSD test) and +>sun-RNAi control (p=0.0119; two-way ANOVA followed by Tukey HSD test), but not in r4>sun-RNAi larvae (p=0.1121; two-way ANOVA followed by Tukey HSD test). n = 7–8 biological replicates. (G) Adult weight was significantly higher in female flies raised in 2Y compared with females raised in 1Y in r4>+ and +>UAS-sun-RNAi controls (p<0.0001 for both genotypes; two-way ANOVA followed by Tukey HSD test); however, adult weight was not significantly different between r4>UAS-sun-RNAi females reared on 2Y compared with genotype-matched females raised on 1Y (p=0.5035; two-way ANOVA followed by Tukey HSD test). n = 7–10 groups of 10 flies. (H) Adult weight was not significantly higher in male flies reared in 2Y compared with males cultured in 1Y for r4>+ and +>UAS-sun-RNAi controls or r4>UAS-sun-RNAi males (p=0.8883, 0.6317, and 0.554, respectively; two-way ANOVA followed by Tukey HSD test). There was a significant sex:diet interaction in the r4>+ and +>UAS-sun-RNAi control genotypes (p=0.011 and p=0.0005, respectively; two-way ANOVA followed by Tukey HSD test), but no sex:diet interaction in the r4>UAS-sun-RNAi genotype (p=0.8749; two-way ANOVA followed by Tukey HSD test). n = 6–9 groups of 10 flies. For all body size plasticity graphs, filled circles indicate mean body size, and dashed lines indicate 95% confidence interval. * indicates p<0.05, ** indicates p<0.01, *** indicates p<0.001 **** indicates p<0.0001; ns indicates not significant; error bars indicate SEM.
Given that a comprehensive series of genetic, molecular, and organ co-culture experiments have established that Sun promotes IIS activity by enhancing Dilp2 secretion (Delanoue et al., 2016), we hypothesized that the female-specific increase in sun mRNA levels in 2Y triggers the nutrient-dependent upregulation of IIS activity in females. To test this, we overexpressed UAS-sun-RNAi in the larval fat body using r4-GAL4, and cultured the animals on either 1Y or 2Y. Importantly, overexpression of the UAS-sun-RNAi transgene significantly decreased sun mRNA levels in both sexes (Figure 3—figure supplement 2A,B), where GAL4 expression was similar between the sexes in 1Y and 2Y (Figure 3—figure supplement 2C). In control r4>+ and +>UAS-sun-RNAi females, we observed a significant decrease in Foxo target gene expression in larvae cultured on 2Y compared with genotype-matched larvae reared on 1Y (Figure 3E). In contrast, the nutrient-dependent decrease in Foxo target gene expression was absent in r4>UAS-sun-RNAi females (Figure 3E; diet:genotype interaction p<0.0001; Supplementary file 1), suggesting sun is required in females for the nutrient-dependent increase in IIS activity. In males, the magnitude of the nutrient-dependent decrease in Foxo target gene expression was smaller than in genotype-matched females for the r4>+ and +>UAS-sun-RNAi control strains (p=0.0166 [r4>+]; p=0.0119 [+>UAS-sun-RNAi]; Supplementary file 1), but not in the r4>UAS-sun-RNAi strain (Figure 3F) (sex:diet interaction p=0.1121 [r4>UAS-sun-RNAi]; Supplementary file 1). Importantly, the lack of a diet:genotype interaction among males indicates that there was no effect of genotype on Foxo target gene expression (p=0.1068; Supplementary file 1). Together, this data suggests that in females a protein-rich diet stimulates a nutrient-dependent increase in sun mRNA that promotes IIS activity. In males, the 2Y diet did not augment sun mRNA levels, suggesting one reason for the female-biased increase in IIS activity in a protein-rich diet.
We next asked whether the female-specific increase in sun mRNA and its impact on IIS activity contribute to the nutrient-dependent increase in female body size in a protein-rich context. In r4>+ and +>UAS-sun-RNAi control females, adult weight was significantly higher in flies cultured on 2Y compared with genotype-matched flies raised on 1Y (Figure 3G). In contrast, the nutrient-dependent increase in adult weight was abolished in r4>UAS-sun-RNAi females (Figure 3G; genotype:diet interaction p=0.0014; Supplementary file 1). This indicates r4>UAS-sun-RNAi females have reduced nutrient-dependent body size plasticity, a finding that cannot be explained by changes to feeding behavior (Figure 3—figure supplement 3A). In r4>+, +>UAS-sun-RNAi, and r4>UAS-sun-RNAi male flies raised on 2Y, adult weight was not significantly higher than in genotype-matched males raised on 1Y (Figure 3H; genotype:diet interaction p=0.9278; Supplementary file 1). Importantly, in contrast to the sex difference in nutrient-dependent body size plasticity we observed in the r4>+ and +>UAS-sun-RNAi control genotypes (sex:diet interaction p=0.011 and p=0.0005, respectively; Supplementary file 1), the sex difference in phenotypic plasticity was abolished in the r4>UAS-sun-RNAi genotype (sex:diet interaction p=0.8749; Supplementary file 1), findings we reproduced using pupal volume (Figure 3—figure supplement 4A,B). While we observed no phenotypic plasticity effects in larvae with whole-body, pan-neuronal, or IPC loss of Sun receptor methuselah (mth; Fbgn0023000; Delanoue et al., 2016; Figure 3—figure supplement 5A–F), likely due to use of different dilp2-GAL4 lines, minor variation in rearing conditions, and sex-specific plasticity defects in the dilp2-GAL4 strain, we reproduced the female-specific effects of sun knockdown on body size using an additional fat body GAL4 line (Figure 3—figure supplement 6A). Further, we show that this role for sun in mediating the nutrient-dependent increase in female body size in a protein-rich context is unique to sun, as no other humoral factors caused sex-specific effects on body size (Figure 3—figure supplement 6B,C).
Our data suggests a model in which the nutrient-dependent increase in sun mRNA levels is one important reason that females raised in a protein-rich context have a larger body size. To determine whether increased sun mRNA levels could augment body size, we overexpressed sun specifically in the fat body in larvae of each sex reared on 1Y and 2Y. We found that fat body sun overexpression was sufficient to increase body size in both sexes, in both the 1Y and 2Y diets (Figure 3—figure supplement 7A,B). This demonstrates that increased sun mRNA levels are sufficient to enhance body size in these contexts. While this finding contrasts with data from a previous study using a different diet and a mixed-sex experimental group (Delanoue et al., 2016), when we replicated their experimental conditions we found a significant increase in body size that was obscured by pooling data from males and females (Figure 3—figure supplement 8A,B). Together, this data supports a model in which increased fat body sun mRNA levels enhance body size in multiple nutritional contexts, an effect that was previously overlooked due to minor variation between lab diets and use of a mixed-sex experimental group. It is important to note, however, that despite the larger body size of sun-overexpressing males and females, phenotypic plasticity was not increased in the sun-overexpressing larvae (Figure 3—figure supplement 7A,B; diet:genotype interaction p=0.4959; and p=0.0895, respectively; Supplementary file 1). This is likely due to the fact that the nutrient-dependent increase in sun mRNA levels was still absent in the context of sun overexpression in males (Figure 3—figure supplement 8C), as our model suggests it is the ability to upregulate sun mRNA in response to dietary protein, rather than absolute sun mRNA levels, that allows females raised on a protein-rich diet to achieve a larger body size.
Sex determination gene transformer promotes nutrient-dependent body size plasticity in females
To gain a more complete understanding of the sex difference in phenotypic plasticity, we wanted to identify genetic factors in females that confer the ability to upregulate sun mRNA levels in response to dietary protein. One candidate was sex determination gene tra, as tra was previously found to impact IIS activity and body size in a diet equivalent to 2Y (Rideout et al., 2015; Mathews et al., 2017). Thus, we performed loss- and gain-of-function studies with tra and monitored changes to IIS activity, sun mRNA, and body size in both the 1Y and 2Y diets. In control w1118 females, Foxo target gene expression was significantly lower in larvae raised on 2Y compared with larvae cultured on 1Y (Figure 4A); however, this nutrient-dependent decrease in Foxo target gene expression was abolished in tra mutant females (tra1/Df(3L)st-j7) (Figure 4A; diet:genotype interaction p=0.0081; Supplementary file 1). Similarly, while sun mRNA levels in w1118 control females were significantly higher in larvae raised on 2Y compared with 1Y (Figure 4B), this nutrient-dependent increase in sun mRNA levels was absent in tra mutant females (Figure 4B). This indicates that tra is required in females for the nutrient-dependent increase in sun mRNA levels and IIS activity in a protein-rich context.
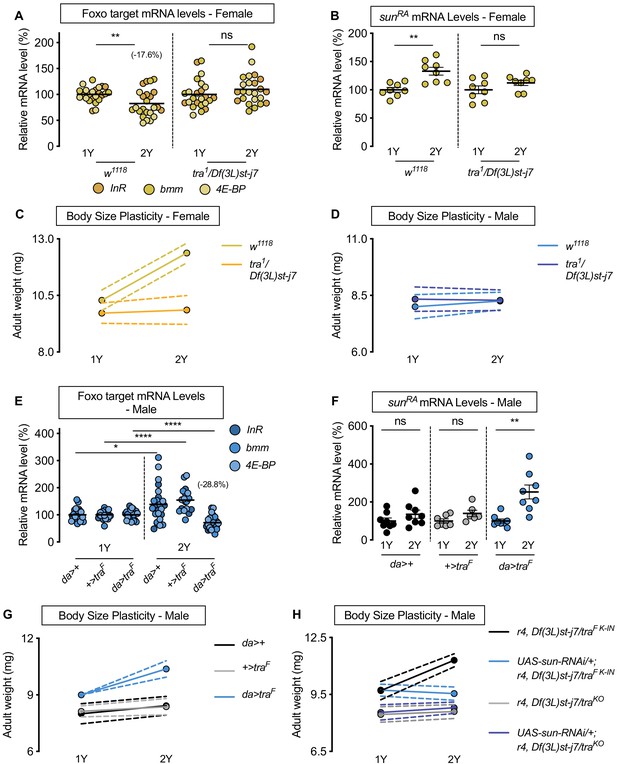
Sex determination gene transformer (tra) regulates increased nutrient-dependent body size plasticity in females.
(A) In control w1118 females, mRNA levels of Foxo targets (insulin receptor (InR), brummer (bmm), and eukaryotic initiation factor 4E-binding protein (4E-BP)), were significantly lower in larvae cultured on a protein-rich diet (2Y) compared with larvae raised on a diet containing half the protein (1Y) (p=0.0057; Student’s t test). In tra mutant (tra1/Df(3L)st-j7) females, there was no significant difference in mRNA levels of Foxo targets in larvae cultured on 2Y compared with larvae raised on 1Y (p=0.2291 Student’s t test). n = 8 biological replicates. (B) In control females, mRNA levels of sunRA were significantly higher in larvae cultured on 2Y compared with larvae raised on 1Y (p=0.0011; Student’s t test); however, in tra1/Df(3L)st-j7 females there was no significant difference in sunRA mRNA levels between larvae cultured on 2Y compared with larvae raised on 1Y (p=0.1644; Student’s t test). n = 8 biological replicates. (C) Adult weight was significantly higher in w1118 females raised on 2Y compared with females reared on 1Y (p<0.0001; two-way ANOVA followed by Tukey HSD test); however, there was no significant difference in adult weight between tra1/Df(3L)st-j7 females cultured on 2Y compared with genotype-matched females raised on 1Y (p=0.9617; two-way ANOVA followed by Tukey HSD test). n = 7–8 groups of 10 flies. (D) Adult weight was not significantly higher in either w1118 control or tra1/Df(3L)st-j7 mutant males in flies raised on 2Y compared with males reared on 1Y (p=0.7808 and p=0.9983, respectively; two-way ANOVA followed by Tukey HSD test). There was a significant sex:diet interaction in the w1118 control genotype (p<0.0001; two-way ANOVA followed by Tukey HSD test); however, there was no sex:diet interaction in the tra1/Df(3L)st-j7 genotype (p=0.6598; two-way ANOVA followed by Tukey HSD test). n = 6–8 groups of 10 flies. (E) In control da>+, and +>traF males, mRNA levels of Foxo targets were significantly higher in larvae cultured on 2Y compared with larvae raised on 1Y a diet containing half the protein content (1Y) (p=0.0108 and p<0.0001, respectively; Student’s t test). However, in da>traF males, there was a significant decrease in Foxo target mRNA levels (p<0.0001; Student’s t test). n = 8 biological replicates. Importantly, there was a significant sex:diet interaction for Foxo target mRNA levels in both the da>+ control (p=0.0004; two-way ANOVA followed by Tukey HSD test) and +>traF control (p<0.0001; two-way ANOVA followed by Tukey HSD test), but not in da>traF larvae (p=0.3095; two-way ANOVA followed by Tukey HSD test). n = 7–8 biological replicates. (F) In control da>+ and +>UAS-traF males, mRNA levels of sunRA were not significantly different between larvae cultured on 2Y compared with larvae raised on 1Y (p=0.2064 and p=0.0711, respectively; Student’s t test). In contrast, da>UAS-traF males showed a significant increase in mRNA levels of sunRA in larvae cultured on 2Y compared with males raised on 1Y (p=0.0013; Student’s t test). n = 6–8 biological replicates. (G) Adult weight was not significantly higher in da>+ and +>UAS-traF control males reared on 2Y compared with genotype-matched males flies cultured on 1Y (p=0.5186 and p=0.8858, respectively; two-way ANOVA followed by Tukey HSD test); however, there was a significant increase in adult weight between da>UAS-traF males cultured on 2Y compared with genotype-matched flies raised on 1Y (p<0.0001; two-way ANOVA followed by Tukey HSD test). n = 7–8 groups of 10 flies. (H) Adult weight was significantly higher in r4-GAL4 control males with traF K-IN, which express physiological levels of a functional Tra protein, when reared on 2Y compared with 1Y ((p<0.0001 [r4,Df(3L)st-j7/traF K-IN]); two-way ANOVA followed by Tukey HSD test). In contrast, the nutrient-dependent increase in adult weight was abolished upon fat body knockdown of sun in a traF K-IN male ((p=0.9915 [UAS-sun-RNAi/+;r4,Df(3L)st-j7/traF K-IN]); two-way ANOVA followed by Tukey HSD test). Adult weight was not different in tra mutant r4-GAL4 males (r4,Df(3L)st-j7/traKO) reared on 2Y compared with genotype-matched males cultured on 1Y (p=0.9980; two-way ANOVA followed by Tukey HSD test). Adult weight was not reduced in 1Y with fat body knockdown of sun in a tra mutant male ((UAS-sun-RNAi/+;r4,Df(3L)st-j7/traKO) (p=0.9998 [UAS-sun-RNAi/+;r4,Df(3L)st-j7/traKO v r4,Df(3L)st-j7/traKO]); two-way ANOVA followed by Tukey HSD test). n = 9–11 groups of 10 flies. For all body size plasticity graphs, filled circles indicate mean body size, and dashed lines indicate 95% confidence interval. * indicates p<0.05, ** indicates p<0.01, **** indicates p<0.0001; ns indicates not significant; error bars indicate SEM.
To determine whether lack of tra also impacts nutrient-dependent body size plasticity, we measured body size in w1118 controls and tra mutants raised in 1Y and 2Y. In control w1118 females, adult weight was significantly higher in flies raised on 2Y compared with flies cultured on 1Y (Figure 4C); however, this nutrient-dependent increase in adult weight was blocked in tra mutant females (Figure 4C; genotype:diet interaction p<0.0001; Supplementary file 1), a finding we reproduced using pupal volume (Figure 4—figure supplement 1A). Given that we confirmed this result using an additional tra mutant allele (traKO) (Hudry et al., 2016; Figure 4—figure supplement 1B), and that this finding cannot be explained by changes to food intake (Figure 4—figure supplement 1C), this indicates that tra mutant females have reduced nutrient-dependent body size plasticity compared with control females (genotype:diet interaction p<0.0001 [tra1/Df(3L)st-j7]; p<0.0001 [traKO]; Supplementary file 1). In control w1118 and tra mutant males, adult weight was not significantly higher in flies raised on 2Y compared with genotype-matched flies reared on 1Y (Figure 4D; genotype:diet interaction p=0.4507, Supplementary file 1). Given that we observed a sex difference in nutrient-dependent body size plasticity in the w1118 genotype (sex:diet interaction p<0.0001; Supplementary file 1), but not in the tra mutant strains (sex:diet interaction p=0.6598 [tra1/Df(3L)st-j7]; p=0.5068 [traKO]; Supplementary file 1), findings we replicated with pupal volume (Figure 4—figure supplement 1D,E), our data reveals a previously unrecognized requirement for tra in regulating the sex difference in nutrient-dependent phenotypic plasticity. To determine whether tra affects phenotypic plasticity via regulation of sun, we overexpressed sun in the fat body of tra mutant females. We found that the reduced body size of tra mutant females in 2Y was rescued by fat body sun overexpression (Figure 4—figure supplement 2A). This supports a model in which the smaller body size of tra mutant females reared in 2Y was due at least in part to lower sun mRNA levels.
To determine whether lack of a functional Tra protein in males explains their reduced nutrient-dependent body size plasticity, we overexpressed UAS-traF in all tissues using daughterless (da)-GAL4. We first asked whether Tra overexpression impacted the nutrient-dependent regulation of sun mRNA and IIS activity. In control da>+ and +>UAS-traF males, there was no significant decrease in Foxo target gene expression in larvae reared in 2Y compared with larvae raised in 1Y (Figure 4E). In da>UAS-traF males, however, there was a significant nutrient-dependent decrease in mRNA levels of Foxo target genes (Figure 4E). Because we observed a significant diet:genotype interaction (p<0.0001; Supplementary file 1), the magnitude of the nutrient-dependent increase in IIS activity in the da>UAS-traF genotype was larger than in control males. Similarly, while sun mRNA levels in control da>+ and +>UAS-traF males were not significantly different in larvae raised on 2Y compared with larvae reared on 1Y (Figure 4F), there was a nutrient-dependent increase in sun mRNA levels in da>UAS-traF males (Figure 4F). In da>+, +>UAS-traF, and da>UAS-traF females, we observed a significant decrease in Foxo target gene expression, and a significant increase in sun mRNA levels (Figure 4—figure supplement 3A,B). Thus, the presence of a functional Tra protein in males confers the ability to upregulate sun mRNA levels and IIS activity, revealing that the lack of Tra in normal males accounts for the lack of a nutrient-dependent increase in sun mRNA and IIS activity.
We next tested whether the presence of a functional Tra protein in males would augment nutrient-dependent body size plasticity. We observed a significant increase in adult weight between da>UAS-traF males reared on 2Y compared with genotype-matched males raised on 1Y (Figure 4G; genotype:diet interaction p=0.0038; Supplementary file 1). This nutrient-dependent increase was not present in either control da>+ or +>UAS-traF males (Figure 4G), a finding we reproduced using pupal volume (Figure 4—figure supplement 3C). Because one study suggested high levels of Tra expression may cause lethality (Siera and Cline, 2008), we repeated the experiment using males from a recently published strain of flies in which flies carry a cDNA encoding the female-specific Tra protein knocked into the tra locus (traF K-IN). These males express Tra at a physiological level (Hudry et al., 2019). As with da>UAS-traF males, we found traF K-IN males had increased nutrient-dependent body size plasticity compared with control w1118 males and traKO males (Figure 4—figure supplement 3D; genotype:diet interaction p<0.0001; Supplementary file 1). Thus, males expressing a functional Tra protein have increased phenotypic plasticity compared with control males, revealing a new role for tra in conferring the ability to adjust body size in response to a protein-rich diet. In females, we observed a significant increase in both adult weight and pupal volume in da>+, +>UAS-traF, and da>UAS-traF flies raised on the 2Y diet compared with genotype-matched females cultured on the 1Y diet (Figure 4—figure supplement 3E,F); however, lack of a significant genotype:diet interaction indicates that phenotypic plasticity in da>UAS-traF females was not different from controls (p=0.5912; Supplementary file 1), findings we reproduced with the traF K-IN allele (Figure 4—figure supplement 3G; genotype:diet interaction p<0.0001 Supplementary file 1). Importantly, the sex difference in nutrient-dependent body size plasticity that we observed in the w1118 control genotype (sex:diet interaction p<0.0001; Supplementary file 1) was abolished between traF K-IN males and their genotype-matched females (p=0.3168; Supplementary file 1). To determine whether the nutrient-dependent upregulation of sun mRNA was required for Tra to enhance male body size in a protein-rich context, we overexpressed the UAS-sun-RNAi transgene in the fat body of traF K-IN males. We found that the nutrient-dependent body size increase in traF K-IN males was blocked in males with fat body sun loss (Figure 4H), a finding we reproduced in traF K-IN females (Figure 4—figure supplement 3H). This indicates that the nutrient-dependent upregulation of sun mRNA in larvae with a functional Tra protein is required for phenotypic plasticity. Together, these data demonstrate a new role for Tra in regulating the sex difference in nutrient-dependent body size plasticity, and identify fat body sun as one downstream factor that mediates Tra’s effects on phenotypic plasticity.
Transcriptional coactivator spargel represents one link between transformer and regulation of sun mRNA levels
While sex determination gene tra impacts sexual differentiation via regulation of confirmed target genes doublesex (dsx; FBgn0000504) and fruitless (fru; FBgn0004652), neither dsx nor fru affect body size (Rideout et al., 2015). Given the key role of sun in mediating the nutrient-dependent increase in body size downstream of Tra, we wanted to identify the link between Tra and regulation of sun mRNA levels. Previous studies show that transcriptional coactivator spargel (srl, FBgn0037248), the Drosophila homolog of peroxisome proliferator-activated receptor gamma coactivator 1-alpha (PGC-1α) (Tiefenböck et al., 2010), coordinates sun mRNA levels with dietary protein (Delanoue et al., 2016). To test whether Srl mediates the sex difference in nutrient-dependent upregulation of sun mRNA levels, we examined mRNA levels of sun in female larvae heterozygous for a strong hypomorphic allele of srl (srl1/+) (Tiefenböck et al., 2010). In females, we found that the nutrient-dependent upregulation of sun mRNA levels in w1118 control larvae was blunted in srl1/+ larvae (Figure 5A; diet:genotype interaction p<0.0001; Supplementary file 1). To determine whether a smaller nutrient-dependent increase in sun mRNA levels affects the ability of srl1/+ larvae to achieve a larger body size in a protein-rich context, we raised srl1/+ larvae on 1Y and 2Y. While we confirmed that srl1/+ larvae have no generalized developmental defects, as there was no decrease in body size in srl1/+ female or male larvae reared on 1Y (Figure 5B,C), we showed that the nutrient-dependent increase in body size in srl1/+ females was eliminated (Figure 5B). Given that adult weight was significantly higher in control w1118 females raised on 2Y compared with genotype-matched females cultured on 1Y (Figure 5B), this indicates that srl1/+ females have reduced nutrient-dependent body size plasticity (genotype:diet interaction p<0.0001; Supplementary file 1). In control w1118 and srl1/+ males, adult weight was not significantly higher in flies raised on 2Y compared with genotype-matched flies reared on 1Y (Figure 5C; genotype:diet interaction p=0.8323). This result suggests that Srl mediates the nutrient-dependent upregulation of sun mRNA levels and increased nutrient-dependent body size plasticity in female larvae in a protein-rich context, where future studies will need to determine whether Srl also impacts the sex difference in circulating Sun. Indeed, while Sun is also regulated at the level of secretion by fat body Target-of-Rapamycin (TOR) signaling (Delanoue et al., 2016), we found no sex difference in fat body TOR activity in either 1Y or 2Y (Figure 5—figure supplement 1A–D). Given that TOR activity does not affect sun mRNA levels (Delanoue et al., 2016), which we confirm (Figure 5—figure supplement 1E), our data indicates that the sex difference in nutrient-dependent upregulation of sun mRNA levels is due to Srl, and not TOR. This aligns with our previous finding that treating larvae with TOR inhibitor Rapamycin did not cause sex-biased effects on larval growth (Rideout et al., 2015).
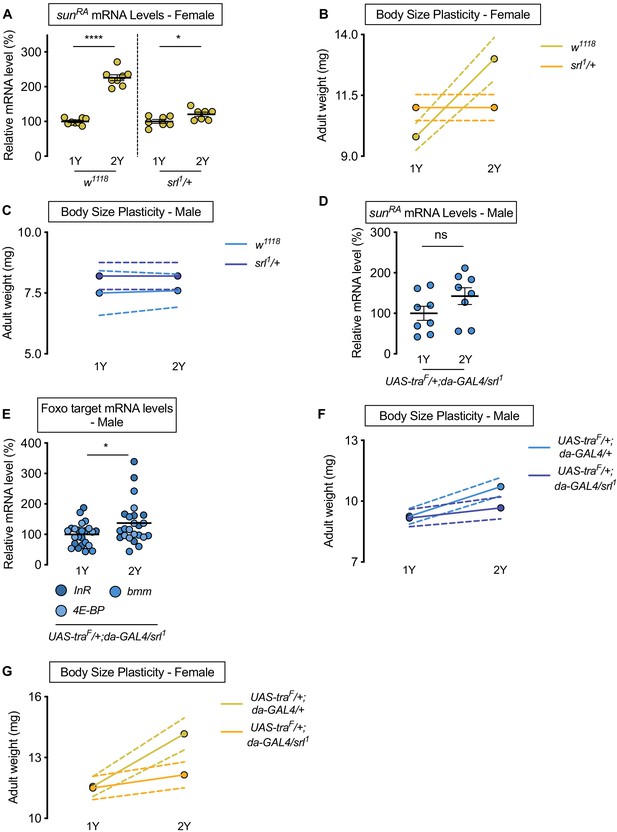
Sex determination gene transformer (tra) requires transcriptional coactivator spargel (srl) for increased nutrient-dependent body size plasticity in females.
(A) In control w1118 females and females with heterozygous loss of srl (srl1/+), mRNA levels of sunRA were significantly higher in larvae cultured on a protein-rich diet (2Y) compared with larvae raised on a diet with half the protein (1Y) (p<0.0001 and p=0.0301; Student’s t test); however, there was a significant genotype:diet interaction indicating that the protein-dependent upregulation of sunRA was blunted in srl1/+ females (p<0.0001; two-way ANOVA followed by Tukey HSD test). n = 7–8 biological replicates. (B) Adult weight was significantly higher in w1118 females raised on 2Y compared with females reared on 1Y (p<0.0001; two-way ANOVA followed by Tukey HSD test); however, there was no significant difference in adult weight between srl1/+ females cultured on 2Y compared with genotype-matched females raised on 1Y (p>0.9999; two-way ANOVA followed by Tukey HSD test). n = 5–7 groups of 10 flies. (C) Adult weight was not significantly higher in either w1118 control or srl1/+ mutant males in flies raised on 2Y compared with males reared on 1Y (p=0.9906 and p>0.9999, respectively; two-way ANOVA followed by Tukey HSD test). n = 4–5 groups of 10 flies. (D) mRNA levels of sunRA were not significantly different in da>traF males with heterozygous loss of srl (UAS-traF/+;da-GAL4/srl1) cultured on 1Y compared to genotype matched males cultured on 2Y (p=0.1405; Student’s t test). n = 8 biological replicates. (E) In control da>traF males with heterozygous loss of srl, mRNA levels of Foxo targets (insulin receptor (InR), brummer (bmm), and eukaryotic initiation factor 4E-binding protein (4E-BP)), were significantly higher in larvae cultured on 2Y compared with larvae raised on 1Y (p=0.0266; Student’s t test). n = 8 biological replicates. (F) Adult weight was higher in da>UAS-traF males raised on 2Y compared with da>UAS-traF males reared on 1Y (p<0.0001; two-way ANOVA followed by Tukey HSD test). In contrast, the nutrient-dependent increase in adult weight was abolished in da>UAS-traF males heterozygous for srl1 (p=0.2811; two-way ANOVA followed by Tukey HSD test). n = 6–8 groups of 10 flies. (G) Adult weight was higher in da>UAS-traF females raised on 2Y compared with da>UAS-traF females reared on 1Y (p<0.0001; two-way ANOVA followed by Tukey HSD test). In contrast, the nutrient-dependent increase in adult weight was absent in da>UAS-traF females heterozygous for srl1 (p=0.2927; two-way ANOVA followed by Tukey HSD test). n = 6–7 groups of 10 flies. For all body size plasticity graphs, filled circles indicate mean body size, and dashed lines indicate 95% confidence interval. * indicates p<0.05, **** indicates p<0.0001; ns indicates not significant; error bars indicate SEM.
To determine whether Srl mediates the Tra-dependent regulation of sun mRNA levels, we measured sun mRNA levels in males with ectopic Tra expression (da>UAS-traF). While da>UAS-traF males show a significant nutrient-dependent upregulation of sun mRNA levels compared with da>+ and +>UAS-traF control males (Figure 4F), we found that sun mRNA levels were no longer higher in da>UAS-traF males heterozygous for the srl1 allele raised on 2Y compared with genotype-matched males reared on 1Y (Figure 5D). Similarly, we observed no decrease in Foxo target genes between da>UAS-traF males heterozygous for the srl1 allele raised on 2Y compared with genotype-matched males reared on 1Y (Figure 5E), indicating the nutrient-dependent upregulation of IIS activity in da>UAS-traF males was abolished in the context of reduced Srl function. Given that we observed no Tra-dependent changes to TOR activity (Figure 5—figure supplement 1F,G), when taken together our data indicates that Srl function is required for the Tra-dependent increase in sun mRNA levels in a protein-rich context. Srl therefore represents an additional link between sex determination gene tra and the regulation of gene expression. Moreover, we show that the Srl-dependent regulation of sun downstream of Tra is significant for phenotypic plasticity, as the nutrient-dependent increase in body size was blocked in da>UAS-traF females and males heterozygous for the srl1 allele (Figure 5F,G; genotype:diet interaction p=0.0146 and p=0.0008, respectively). While we find that Srl targets other than sun were also regulated in a sex-specific manner by nutrients and Tra function (Figure 5—figure supplement 2A–D), other functionally similar Srl targets did not reproduce sex-specific changes to nutrient-dependent body size plasticity that we observed upon loss of fat body sun (Figure 5—figure supplement 2E–H). Although we cannot rule out all Srl targets, our data indicates a key role for sun among Srl targets in mediating the effects of Tra on nutrient-dependent body size plasticity. This reveals a previously unrecognized role for Srl in mediating sex-specific changes to gene expression, and identifies Srl as a new link between Tra and nutrient-dependent changes to gene expression.
Increased nutrient-dependent body size plasticity in females promotes fecundity in a protein-rich context
Previous studies have shown that plentiful nutrients during development maximize body size to promote fertility in Drosophila females (Bergland et al., 2008; Green and Extavour, 2014; Grönke et al., 2010; Hodin and Riddiford, 2000; Klepsatel et al., 2020; Mendes and Mirth, 2016; Robertson, 1957a; Robertson, 1957b; Sarikaya et al., 2012; Tu and Tatar, 2003), and that high levels of IIS activity are required for normal egg development, ovariole number, and fecundity (Green and Extavour, 2014; Grönke et al., 2010; Mendes and Mirth, 2016; Richard et al., 2005). In line with these findings, w1118 female flies reared on 2Y produced significantly more eggs compared with genotype-matched females cultured on 1Y (Figure 6A). This aligns with findings from many studies showing that increased nutrients promote fertility (Green and Extavour, 2014; Grönke et al., 2010; Mendes and Mirth, 2016; Richard et al., 2005) and suggests that the ability to augment IIS activity and body size in response to a protein-rich diet allows females to maximize fecundity in conditions where nutrients are plentiful. To test this, we measured the number of eggs produced by InRE19/+ females and w1118 controls raised in either 1Y or 2Y. In contrast to w1118 females, the nutrient-dependent increase in egg production was absent in InRE19/+ females (Figure 6A). Similarly, there was no diet-induced increase in egg production in dilp2 mutant females (Figure 6B). These findings suggest that the nutrient-dependent increase in IIS activity and body size are important to promote fecundity in a protein-rich context. This result aligns with findings from a previous study showing that lifetime fecundity was significantly lower in dilp2 mutants raised in a yeast-rich diet (Grönke et al., 2010). To extend our findings beyond dilp genes, we next examined fecundity in females with an RNAi-mediated reduction in sun. We found that the nutrient-dependent increase in egg production in r4>UAS-sun-RNAi females was eliminated, in contrast to the robust diet-induced increase in fecundity in r4>+ and +>UAS-sun-RNAi control females (Figure 6C). Together, this data suggests that dilp2 and fat body-derived sun play a role in maximizing IIS activity and body size to promote egg production in a protein-rich context. Future studies will need to determine which aspect of ovary development is affected by these genetic manipulations (Green and Extavour, 2014; Grönke et al., 2010; Mendes and Mirth, 2016; Richard et al., 2005), whether this phenotype is specific to dilp2, and whether the effects require InR function in the ovary or in other tissues.
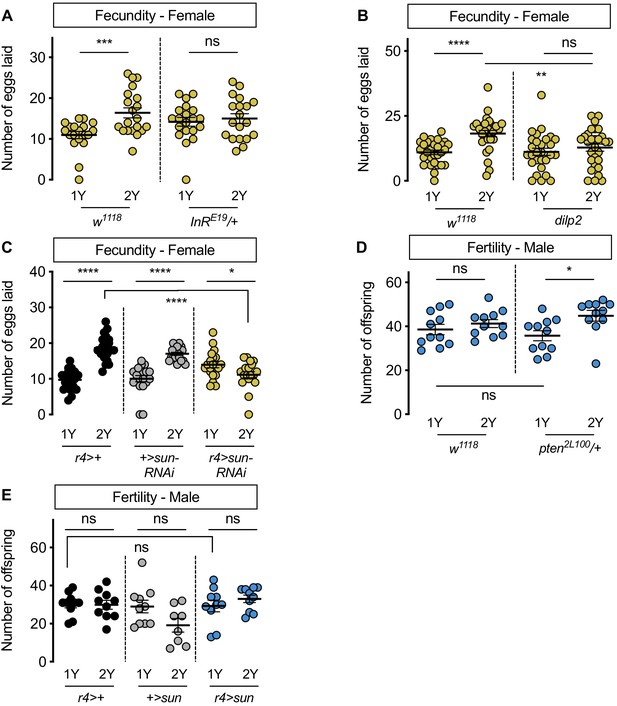
Increased nutrient-dependent body size plasticity in females promotes fertility.
(A) In control w1118 females, there was a significant increase in the number of eggs laid by females raised on a protein-rich diet (2Y) compared with females reared on a diet with half the protein (1Y) (p=0.0009; Student’s t test); however, there was no significant difference in the number of eggs laid between InRE19/+ females cultured on 2Y compared with genotype-matched females raised on 1Y (p=0.617; Student’s t test). n = 19–20 biological replicates. (B) In control w1118 females, there was a significant increase in the number of eggs laid by females raised on 2Y compared with females cultured on 1Y (p<0.0001; Student’s t test); however, there was no significant difference in the number of eggs laid between dilp2 mutant females cultured on 2Y compared with females raised on 1Y (p=0.4105; Student’s t test). n = 28–30 biological replicates. (C) In control r4>+ and +>UAS-sun-RNAi females, there was a significant increase in the number of eggs laid by females raised on 2Y compared with control females cultured on 1Y (p<0.0001 for both genotypes; Student’s t test). In r4>UAS-sun-RNAi females, the number of eggs laid by females cultured on 2Y was lower than females raised on 1Y (p=0.0243; Student’s t test). n = 20 biological replicates. (D) In control w1118 males, there was no significant difference in the number of offspring produced between a 1Y and 2Y diet (p=0.3662; Student’s t test). There was also no significant difference in the number of offspring produced between control w1118 males and males heterozygous for a loss-of-function allele of phosphatase and tensin homolog (pten; genotype pten2L100/+) raised on 1Y (p=0.4003; Student’s t test). Unlike control males, pten2L100/+ males reared on 2Y produced significantly more offspring than genotype-matched males raised on 1Y (p=0.0137; Student’s t test). n = 11 biological replicates. (E) In control r4>+ and +>UAS-sun and r4>UAS-sun males, there was no significant effect on the number of offspring produced between a 1Y and 2Y diet (p=0.9222, 0.0595, and 0.32 respectively; Student’s t test). There was also no significant difference in the number of offspring produced between control r4>+, +>UAS-sun males and r4>UAS-sun males raised on 1Y (p=0.9723 and p=0.9969 respectively; one-way ANOVA followed by Tukey HSD test). n = 8–10 groups of 10 flies. * indicates p<0.05, ** indicates p<0.01, *** indicates p<0.001, **** indicates p<0.0001; ns indicates not significant; error bars indicate SEM.
In males, which have a reduced ability to augment body size in response to a protein-rich diet, we also investigated the relationship between nutrient content, body size, and fertility. When we compared fertility in w1118 males reared on 1Y compared with males raised on 2Y, we found no significant difference in the number of offspring produced (Figure 6D). Thus, neither male body size nor fertility were enhanced by rearing flies in a protein-rich environment. Given that previous studies suggest that a larger body size in males promotes reproductive success (Ewing, 1961; Partridge et al., 1987; Partridge and Farquhar, 1983), we next asked whether genetic manipulations that augment male body size also increased fertility. One way to augment male body size in 1Y is heterozygous loss of phosphatase and tensin homolog (pten, FBgn0026379; pten2L100/+) (Figure 1—figure supplement 7B). Interestingly, fertility was not significantly higher in pten2L100/+ males compared with w1118 controls raised in 1Y (Figure 6D), suggesting that a larger body size does not always augment fertility in males. Similarly, when we measured fertility in r4>UAS-sun males, which are larger than control males (Figure 3—figure supplement 7B), fertility was not significantly different from r4>+ and +>UAS-sun control males (Figure 6E). Interestingly, when we examined fertility in pten2L100/+ and r4>UAS-sun males in 2Y, fertility was significantly increased in pten2L100/+ males compared with genotype-matched controls cultured in 1Y (Figure 6D), an observation we did not repeat in r4>UAS-sun males (Figure 6E). Ultimately, this less robust and more complex relationship between body size and fertility in males suggests a possible explanation for their decreased nutrient-dependent body size plasticity compared with females.
Discussion
In many animals, body size plasticity in response to environmental factors such as nutrition differs between the sexes (Fairbairn, 1997). While past studies have identified mechanisms underlying nutrient-dependent growth in a mixed-sex population, and revealed factors that promote sex-specific growth in a single nutritional context, the mechanisms underlying the sex difference in nutrient-dependent body size plasticity remain unknown. In this study, we showed that females have higher phenotypic plasticity compared with males when reared on a protein-rich diet, and elucidated the molecular mechanisms underlying the sex difference in nutrient-dependent body size plasticity in this context. Our data suggests a model in which high levels of dietary protein augment female body size by stimulating an increase in IIS activity, where we identified a requirement for dilp2 and sun in promoting this nutrient-dependent increase in IIS activity. Importantly, we discovered tra as the factor responsible for stimulating sun mRNA levels and IIS activity in a protein-rich context, revealing a novel role for sex determination gene tra in regulating phenotypic plasticity. Mechanistically, tra enhanced sun mRNA levels and body size in protein-rich conditions via transcriptional coactivator Srl, identifying Srl as one link between tra and the nutrient-dependent regulation of gene expression. Together, these findings provide new insight into how Drosophila females achieve increased nutrient-dependent body size plasticity compared with males.
One key feature of this increased phenotypic plasticity in females was a female-biased increase in IIS activity in a protein-rich context. This reveals a previously unrecognized sex difference in the coupling between IIS activity and dietary protein. In females, there was tight coupling between increased nutrient input and enhanced IIS activity across a wide protein concentration range in all control genotypes. In males, this close coordination between dietary protein and IIS activity was weaker in a protein-rich context. Our data shows that sex-biased nutrient-dependent change to IIS activity during development is physiologically significant, as it supports an increased rate of growth and consequently larger body size in females but not in males raised on a protein-rich diet. In future studies, it will be important to determine whether the sex difference in coupling between nutrients and IIS activity exists in other contexts. For example, previous studies on the extension of life span by dietary restriction have shown that male and female flies differ in the concentration of nutrients that produces the maximum life span extension, and in the magnitude of life span extension produced by dietary restriction (Magwere et al., 2004; Regan et al., 2016). Similar sex-specific effects of dietary restriction and reduced IIS on life span have also been observed in mice (Holzenberger et al., 2003; Kane et al., 2018; reviewed in Regan and Partridge, 2013; Selman et al., 2008) and humans (Van Heemst et al., 2005). Future studies will be needed to determine whether a male-female difference in coupling between nutrients and IIS activity account for these sex-specific life span responses to dietary restriction. Indeed, given that sex differences have been reported in the risk of developing diseases associated with overnutrition and dysregulation of IIS activity such as obesity and type 2 diabetes (Kautzky-Willer et al., 2016; Mauvais-Jarvis, 2018; Tramunt et al., 2020), more detailed knowledge of the male-female difference in coupling between nutrients and IIS activity in other models may provide insights into this sex-biased risk of disease.
In addition to revealing a sex difference in the nutrient-dependent upregulation of IIS activity, our data identified a female-specific requirement for dilp2 and sun in mediating the diet-induced increase in IIS activity in a protein-rich context. While previous studies have shown that both dilp2 and sun positively regulate body size (Ikeya et al., 2002; Grönke et al., 2010; Delanoue et al., 2016), we describe new sex-specific roles for dilp2 and sun in nutrient-dependent phenotypic plasticity. Elegant studies have shown that sun is a secreted factor that stimulates Dilp2 release from the IPCs (Delanoue et al., 2016). Together with our data, this suggests a model in which females are able to achieve a larger body size in a protein-rich diet because they have the ability to upregulate sun mRNA levels, whereas males do not. Indeed, we show that higher sun mRNA levels are sufficient to augment body size. This model aligns well with findings from two previous studies on Dilp2 secretion in male and female larvae. The first study, which raised larvae on a protein-rich diet equivalent to the 2Y diet, found increased Dilp2 secretion in females compared to males (Rideout et al., 2015). The second study, which raised larvae on a diet equivalent to the 1Y diet, found no sex difference in Dilp2 secretion and no effects of dilp2 loss on body size (Sawala and Gould, 2017). Thus, while these previous studies differed in their initial findings on a sex difference in Dilp2 secretion, our data reconcile these minor differences by identifying context-dependent effects of dilp2 on body size. It is important to note that absolute confirmation of a sex difference in hemolymph Dilp2 levels will be needed in future studies because the body size plasticity defects in the dilp2-HF strain precluded its use as a tool to quantify circulating Dilp2 levels in our study. Future studies will also need to determine whether these sex-specific and context-dependent effects of dilp2 are observed in other phenotypes regulated by dilp2 and other dilp genes. For example, flies carrying mutations in dilp genes show changes to aging, metabolism, sleep, and immunity, among other phenotypes (Bai et al., 2012; Brown et al., 2020; Cong et al., 2015; Grönke et al., 2010; Liu et al., 2016; Nässel and Vanden Broeck, 2016; Okamoto et al., 2009; Okamoto and Nishimura, 2015; Post et al., 2018; Post et al., 2019; Slaidina et al., 2009; Stafford et al., 2012; Zhang et al., 2009; Brogiolo et al., 2001; Cognigni et al., 2011; Linneweber et al., 2014; Semaniuk et al., 2018; Suzawa et al., 2019; Ugrankar et al., 2018). Further, it will be interesting to determine whether the sex-specific regulation of sun is observed in any other contexts, and whether it will influence sex differences in phenotypes associated with altered IIS activity, such as life span.
While our findings on sun and dilp2 provide mechanistic insight into the molecular basis for the larger body size of females reared on a protein-rich diet, a key finding from our study was the identification of sex determination gene tra as the factor that confers plasticity to females. Normally, nutrient-dependent body size plasticity is higher in females than in males in a protein-rich context. In females lacking a functional Tra protein, however, this increased nutrient-dependent body size plasticity was abolished. In males, which normally lack a functional Tra protein, ectopic Tra expression conferred increased nutrient-dependent body size plasticity. While a previous study showed that on the 2Y diet Tra promotes Dilp2 secretion (Rideout et al., 2015), our current study extends this finding in two ways: by identifying sun as one link between Tra, Dilp2, and changes to IIS activity; and by showing that Tra regulates sun mRNA via conserved transcriptional coactivator Srl. While previous studies discovered Srl as the factor that promotes sun mRNA levels in response to dietary protein in a mixed-sex larval population (Delanoue et al., 2016), our findings reveal a previously unrecognized sex-specific role for Srl in regulating transcription. Because loss of Tra reduces Srl transcriptional activity, this new link between Tra and Srl suggests an additional way in which Tra may impact gene expression beyond its canonical downstream targets dsx and fru. While this builds on recent studies that reveal a number of additional Tra-regulated genes (Clough et al., 2014; Hudry et al., 2016; Hudry et al., 2019), it will be important to determine whether these additional Tra-regulated genes including sun represent direct targets of Tra/Srl. Future studies will also be needed to elucidate how Tra impacts Srl transcriptional activity in a context-dependent manner. However, uncovering a connection between a sex determination gene and a key regulator of genes involved in mitochondrial function suggests an additional mechanism that may contribute to sex differences in phenotypes affected by mitochondrial function (e.g. lifespan) (Tiefenböck et al., 2010; Cho et al., 2011; Tower, 2015; Tower, 2017). In addition, it will be critical to explore how the presence of Tra allows an individual to couple dietary protein with body size. Because the tra locus is regulated both by alternative splicing and transcription (Belote et al., 1989; Boggs et al., 1987; Grmai et al., 2018; Inoue et al., 1990; Sosnowski et al., 1989), and Tra protein is regulated by phosphorylation (Du et al., 1998), our study highlights the importance of additional studies on the regulation of the tra genomic locus and Tra protein throughout development to gain mechanistic insight into its effects on nutrient-dependent body size plasticity.
While the main outcome of our work was to reveal the molecular mechanisms that regulate the sex difference in nutrient-dependent body size plasticity, we also provide some insight into how genes that contribute to nutrient-dependent body size plasticity affect female fecundity and male fertility. Our findings align well with previous studies demonstrating that increased nutrient availability during development and a larger female body size confers increased ovariole number and fertility (Green and Extavour, 2014; Klepsatel et al., 2020; Mendes and Mirth, 2016; Robertson, 1957a; Robertson, 1957b), as females lacking either dilp2 or fat body-derived sun were unable to augment egg production in a protein-rich context. Given that previous studies demonstrate IIS activity influences germline stem cells in the ovary in adult flies (Hsu et al., 2008; Hsu and Drummond-Barbosa, 2009; Kao et al., 2015; LaFever and Drummond-Barbosa, 2005; Lin and Hsu, 2020; Su et al., 2018), there is a clear reproductive benefit that arises from the tight coupling between nutrient availability, IIS activity, and body size in females. In males, however, the relationship between fertility and body size remains less clear. While larger males are more reproductively successful both in the wild and in laboratory conditions (Ewing, 1961; Partridge and Farquhar, 1983), other studies revealed that medium-sized males were more fertile than both larger and smaller males (Lefranc and Bundgaard, 2000). Given that our study revealed no significant increase in the number of progeny produced by larger males, the fertility benefits that accompany a larger body size in males may be context-dependent. For example, a larger body size increases the ability of males to outcompete smaller males (Flatt, 2020; Partridge et al., 1987; Partridge and Farquhar, 1983). Thus, in crowded situations, a bigger body may provide significant fertility gains. On the other hand, in conditions where nutrients are limiting, an imbalance in the allocation of energy from food to growth rather than to reproduction may decrease fertility (Bass et al., 2007; Camus et al., 2017; Jensen et al., 2015; Wood et al., 2018). Future studies will need to resolve the relationship between body size and fertility in males, as this will suggest the ultimate reason(s) for the sex difference in nutrient-dependent body size plasticity.
Materials and methods
Reagent type (species) or resource | Designation | Source or reference | Identifiers | Additional information |
---|---|---|---|---|
Genetic reagent (Drosophila melanogaster) | Canton-S | Bloomington Drosophila stock center | BDSC: 64349 | |
Genetic reagent (Drosophila melanogaster) | w1118 | Bloomington Drosophila stock center | BDSC: 3605 | |
Genetic reagent (Drosophila melanogaster) | tra1 | Bloomington Drosophila stock center | BDSC: 675 | |
Genetic reagent (Drosophila melanogaster) | Df(3L)st-j7 | Bloomington Drosophila stock center | BDSC: 5416 | |
Genetic reagent (Drosophila melanogaster) | srl1 | Bloomington Drosophila stock center | BDSC: 14965 | |
Genetic reagent (Drosophila melanogaster) | InRE19 | Bloomington Drosophila stock center | BDSC: 9646 | |
Genetic reagent (Drosophila melanogaster) | TRiP Control | Bloomington Drosophila stock center | BDSC: 36303 | |
Genetic reagent (Drosophila melanogaster) | UAS-dilp2-RNAi | Bloomington Drosophila stock center | BDSC: 32475 | |
Genetic reagent (Drosophila melanogaster) | UAS-upd2-RNAi | Bloomington Drosophila stock center | BDSC: 33949 | |
Genetic reagent (Drosophila melanogaster) | UAS-traF | Bloomington Drosophila stock center | BDSC: 4590 | |
Genetic reagent (Drosophila melanogaster) | UAS-rheb | Bloomington Drosophila stock center | BDSC: 9688 | |
Genetic reagent (Drosophila melanogaster) | UAS-cyt-c-p-RNAi | Bloomington Drosophila stock center | BDSC: 64898 | |
Genetic reagent (Drosophila melanogaster) | UAS-Idh-RNAi | Bloomington Drosophila stock center | BDSC: 41708 | |
Genetic reagent (Drosophila melanogaster) | mth1 | Bloomington Drosophila stock center | BDSC: 27896 | |
Genetic reagent (Drosophila melanogaster) | y1,w1 | Bloomington Drosophila stock center | BDSC: 1495 | |
Genetic reagent (Drosophila melanogaster) | UAS-sun-RNAi | Vienna Drosophila resource center | VDRC: GD23685 | |
Genetic reagent (Drosophila melanogaster) | UAS-Gbp1-RNAi | Vienna Drosophila resource center | VDRC: KK108755 | |
Genetic reagent (Drosophila melanogaster) | UAS-Gbp2-RNAi | Vienna Drosophila resource center | VDRC: GD16696 | |
Genetic reagent (Drosophila melanogaster) | UAS-CCHa2-RNAi | Vienna Drosophila resource center | VDRC: KK102257 | |
Genetic reagent (Drosophila melanogaster) | UAS-mth-RNAi | Vienna Drosophila resource center | VDRC: KK106399 | |
Genetic reagent (Drosophila melanogaster) | dilp2 | Grönke et al., 2010 | ||
Genetic reagent (Drosophila melanogaster) | pten2L100 | Oldham et al., 2002 | ||
Genetic reagent (Drosophila melanogaster) | UAS-sun | Delanoue et al., 2016 | ||
Genetic reagent (Drosophila melanogaster) | traKO | Hudry et al., 2016 | ||
Genetic reagent (Drosophila melanogaster) | traF K-IN | Hudry et al., 2019 | ||
Genetic reagent (Drosophila melanogaster) | y,w;;ilp2HF | Park et al., 2014 | ||
Genetic reagent (Drosophila melanogaster) | tGPH (GFP-PH) | Britton et al., 2002 | ||
Genetic reagent (Drosophila melanogaster) | da-GAL4 | Bloomington Drosophila stock center | BDSC: 55849 | Note. Discontinued stock, equivalent stocks available |
Genetic reagent (Drosophila melanogaster) | r4-GAL4 | Bloomington Drosophila stock center | BDSC: 33832 | |
Genetic reagent (Drosophila melanogaster) | cg-GAL4 | Bloomington Drosophila stock center | BDSC: 7011 | |
Genetic reagent (Drosophila melanogaster) | elav-GAL4 | Bloomington Drosophila stock center | BDSC: 458 | |
Genetic reagent (Drosophila melanogaster) | dilp2-GAL4 | Rulifson et al., 2002 | ||
Antibody | Anti-sun guinea pig polyclonal | Delanoue et al., 2016 | (1:50) | |
Antibody | Anti-Cv-d guinea pig polyclonal | Palm et al., 2012 | (1:1000) | |
Antibody | Anti-pS6k rabbit polyclonal | Cell Signaling: 9209 | (1:1000) | |
Antibody | Anti-Actin mouse monoclonal | Santa Cruz: 8432 | (1:1000) |
Fly husbandry
Request a detailed protocolLarvae were raised at a density of 50 animals per 10 ml food at 25°C on Drosophila growth medium consisting of: 0.5x: 5.125 g/L sucrose, 17.725 g/L D-glucose, 12.125 g/L cornmeal, 11.325 g/L yeast, 4.55 g/L agar, 0.5 g CaCl2•2H2O, 0.5 g MgSO4•7H2O, 11.77 mL acid mix (propionic acid/phosphoric acid). 1x: 10.25 g/L sucrose, 25.45 g/L D-glucose, 24.25 g/L cornmeal, 22.65 g/L yeast, 4.55 g/L agar, 0.5 g CaCl2•2H2O, 0.5 g MgSO4•7H2O, 11.77 mL acid mix (propionic acid/phosphoric acid). 2x: 20.5 g/L sucrose, 70.9 g/L D-glucose, 48.5 g/L cornmeal, 45.3 g/L yeast, 4.55 g/L agar, 0.5 g CaCl2•2H2O, 0.5 g MgSO4•7H2O, 11.77 mL acid mix (propionic acid/phosphoric acid). 1Y: 20.5 g/L sucrose, 70.9 g/L D-glucose, 48.5 g/L cornmeal, 22.65 g/L yeast, 4.55 g/L agar, 0.5 g CaCl2•2H2O, 0.5 g MgSO4•7H2O, 11.77 mL acid mix (propionic acid/phosphoric acid). 2Y: 20.5 g/L sucrose, 70.9 g/L D-glucose, 48.5 g/L cornmeal, 45.3 g/L yeast, 4.55 g/L agar, 0.5 g CaCl2•2H2O, 0.5 g MgSO4•7H2O, 11.77 mL acid mix (propionic acid/phosphoric acid). Details for diets manipulating dietary sugar (1S) and calorie content (2Y calories) are found in Supplementary file 3. Our diets were also deposited in the Drosophila Dietary Composition Calculator (DDCC) (Lesperance and Broderick, 2020). Animals were collected as indicated in figure legends, and sexed by gonad size. When gonad size could not be used to determine sex (e.g. tra mutants, da-GAL4>UAS-traF), chromosomal females were identified by the presence of an X-linked GFP. Adult flies were maintained at a density of 20 flies per vial in single-sex groups.
Fly strains
Request a detailed protocolThe following fly strains from the Bloomington Drosophila Stock Center were used: Canton-S (#64349), w1118 (#3605), tra1 (#675), Df(3L)st-j7 (#5416), srl1 (#14965), InRE19 (#9646), TRiP control (#36303), UAS-ilp2-RNAi (#32475), UAS-upd2-RNAi (#33949), UAS-traF (#4590), y,w (#1495), da-GAL4 (ubiquitous), r4-GAL4 (fat body), cg-GAL4 (fat body), dilp2-GAL4 (IPCs), elav-GAL4 (post-mitotic neurons), UAS-rheb (#9688), UAS-cyt-c-p-RNAi (#64898), UAS-Idh-RNAi (#41708), mth1 (#27896). The following fly strains from the Vienna Drosophila Resource Center were used in this study: UAS-sun-RNAi (GD23685), UAS-Gbp1-RNAi (KK108755) UAS-Gbp2-RNAi (GD16696), UAS-CCHa2-RNAi (KK102257), UAS-mth-RNAi (KK106399). Additional fly strains include: dilp2 (Grönke et al., 2010), pten2L100, UAS-sun, tGPH (GFP-PH), traKO (Hudry et al., 2016), traF K-IN(Hudry et al., 2019), y,w;;ilp2HF (Park et al., 2014). All genotypes used in the manuscript are listed in Supplementary file 4.
Body size
Request a detailed protocolPupal volume was measured in male and female pupae as previously described (Delanoue et al., 2010; Marshall et al., 2012; Rideout et al., 2012; Rideout et al., 2015). For adult weight, 5-day-old virgin male and female flies were weighed in groups of 10 in 1.5 ml microcentrifuge tubes on an analytical balance. Wing length was measured as previously described (Garelli et al., 2012).
Developmental timing
Request a detailed protocolLarvae were placed into the experimental diet ±2 hr post-hatching. Percent pupation was calculated by comparing the number of pupae at 12 hr intervals to the total pupae in the vial after all animals pupated.
Feeding behavior
Request a detailed protocolFeeding behavior was quantified in sexed larvae by counting mouth hook contractions for 30 s.
Protease feeding experiments
Request a detailed protocolWe treated larvae with a broad-spectrum protease inhibitor (PIC; Sigma-Aldrich #P2714) or a serine protease-specific inhibitor (AEBSF; Sigma-Aldrich #A8456) by adding the inhibitors to the food at final concentrations of 100 ml of 1x PIC per L, and 4 mM AEBSF as previously described (Erkosar et al., 2015).
RNA extraction and cDNA synthesis
Request a detailed protocolOne biological replicate represents ten larvae frozen on dry ice and stored at −80°C. Each experiment contained three to four biological replicates per sex, per genotype, and per diet, and each experiment was repeated twice. RNA was extracted using Trizol (Thermo Fisher Scientific; 15596018) according to manufacturer’s instructions, as previously described (Marshall et al., 2012; Rideout et al., 2012; Rideout et al., 2015; Wat et al., 2020). cDNA synthesis was performed using the QuantiTect Reverse Transcription Kit according to manufacturer’s instructions (Qiagen; 205314).
Quantitative real-time PCR (qPCR)
Request a detailed protocolqPCR was performed as previously described (Rideout et al., 2012; Rideout et al., 2015; Wat et al., 2020). To determine changes in Foxo target gene expression, we plotted and analyzed the fold change in mRNA levels for each of three known Foxo target genes (InR, bmm, and 4E-BP) together to quantify IIS activity in each sex and dietary context, an established approach to analyze co-regulated genes (Blaschke et al., 2013; Hudry et al., 2019). A complete primer list is available in Supplementary file 5.
Preparation of protein extract
Request a detailed protocolDissected fat bodies were prepared for SDS-PAGE by homogenizing sets of ten larval fat bodies 108 hr after egg laying in an appropriate volume of lysis buffer (20 mM Hepes (pH 7.8), 450 mM NaCl, 25% glycerol, 50 mM NaF, 0.2 mM EDTA, 1 mM DTT, 1× Protease Inhibitor Cocktail (Roche, 04693124001), 1x Phosphatase Inhibitor Cocktail (Roche, 4906845001) using the Omni Bead Ruptor (VWR). Cellular fragments were pelleted, and supernatant collected by centrifugation for 5 min at 10,000 rpm at 4°C (Thermo Scientific, Heraeus Pico 21 centrifuge). Protein concentration was determined by Bradford assay (Bio-Rad #550–0205) prior to SDS-PAGE).
SDS-PAGE and Western blotting
Request a detailed protocolA total of 20 μL of sample with 20 μg protein was loaded into each well. Proteins were separated using a 12% gel SDS-PAGE gel in SDS running buffer, and transferred to a nitrocellulose membrane (Bio-Rad) for 2 hr at 40 V on ice. Membranes were incubated for 1 hr in blocking buffer (5% milk or 5% BSA in TBST 0.1%) then incubated with primary antibodies overnight at 4°C. Membranes were washed (3 × 2 min) in TBST 0.1% then probed with secondary antibodies in blocking buffer for 1 hr at room temperature. After washes (3 × 2 min, 2 × 15 min, 1 × 5 min) in TBST 0.1%, membranes were treated with Pierce ECL (Thermo Scientific #32134) or Immobilon Forte (Millipore #WBLUF0100). Images were quantified using Image Studio (LI-COR). Primary antibodies: Anti-pS6K (#9209; Cell Signalling), and anti–actin (#8432; Santa Cruz), were used at 1:1000. HRP-conjugated secondary antibodies were used at 1:5000 for pS6k (anti-rabbit #65–6120; Invitrogen) and 1:3000 for actin (anti-mouse #7076; Cell Signalling).
Hemolymph Western blotting
Request a detailed protocolHemolymph Western blotting was performed as previously described (Delanoue et al., 2016). Briefly, hemolymph from 40 larvae was collected in 40 μL of PBS with protease and phosphatase inhibitors (Roche 04693124001, Roche 4906845001), and hemocytes were removed by centrifugation according to the published protocol (Delanoue et al., 2016). Antibody concentrations used to detect hemolymph proteins were 1:50 for anti-Sun and 1:1000 for anti-Cv-d. Anti-guinea pig HRP-conjugated secondary was used at 1:2000.
Fecundity and fertility
Request a detailed protocolFor female fecundity, single 6-day-old virgin female flies raised as indicated were crossed to three age-matched CS virgin males for a 24 hr mating period. Flies were transferred to fresh food vials with blue 2Y food to lay eggs. The number of eggs laid over 24 hr was quantified. For male fertility, single 6-day-old virgin males were paired with three 6-day-old virgin CS females to mate, and females were allowed to lay eggs for 24 hr. The number of progeny was quantified by counting viable pupae.
Microscopy
Request a detailed protocolGFP-PH larvae were picked into 1Y or 2Y food. Larvae were dissected 108 hr after egg laying (AEL) and inverted carcasses were fixed for 30 min in 4% paraformaldehyde in phosphate buffered saline (PBS) at room temperature. Carcasses were rinsed twice with PBS, once in 0.1% Triton-X in PBS (PBST) for 5 min, then incubated with Hoechst (5 μg/mL, Life Technologies H3570), , and phalloidin fluor 647 (1:1000, Abcam ab176759) in PBST for 40 min. The stained carcasses were washed with PBS and mounted in SlowFade Diamond (Thermo Fisher Scientific S36972). Images were acquired with a Leica SP5 (20X). Mean GFP intensity was quantified at the cell membrane (marked by phalloidin) and in the cytoplasm using Fiji (Schindelin et al., 2012). Three cells per fat body were measured, and at least five fat bodies per sex and per diet were measured.
Statistics and data presentation
Request a detailed protocolStatistical analyses and data presentation were carried out using Prism GraphPad 6 (GraphPad Prism version 8.4.3 for Mac OS X). Statistical tests are indicated in figure legends and all p-values are listed in Supplementary file 1.
Data availability
All data generated in this study are provided in Supplementary file 2. All statistical tests and p-values are listed in Supplementary file 1. Exact diets used in this study are described in Supplementary file 3 for ease of replication. All genotypes used in this study are listed in Supplementary file 4. A complete list of primers used in this study is provided in Supplementary file 5.
References
-
The lnk/SH2B adaptor provides a fail-safe mechanism to establish the insulin receptor-Chico interactionCell Communication and Signaling 11:26.https://doi.org/10.1186/1478-811X-11-26
-
Phenotypical variation in body and cell size of Drosophila melanogasterThe Biological Bulletin 58:85–103.https://doi.org/10.2307/1537121
-
Molecular genetic dissection of the sex-specific and vital functions of the Drosophila melanogaster sex determination gene fruitlessGenetics 158:1569–1595.
-
Optimization of dietary restriction protocols in DrosophilaThe Journals of Gerontology: Series A 62:1071–1081.https://doi.org/10.1093/gerona/62.10.1071
-
Food level in relation to rate of development and eye pigmentation in Drosophila melanogasterThe Biological Bulletin 75:447–462.https://doi.org/10.2307/1537573
-
The systemic control of growthCold Spring Harbor Perspectives in Biology 7:a019117.https://doi.org/10.1101/cshperspect.a019117
-
Environmental control of the cell cycle in Drosophila: Nutrition activates mitotic and endoreplicative cells by distinct mechanismsDevelopment 125:2149–2158.
-
The creation of sexual dimorphism in the Drosophila somaCurrent Topics in Developmental Biology 83:65–107.https://doi.org/10.1016/S0070-2153(08)00403-1
-
Sex and genotype effects on nutrient-dependent fitness landscapes in Drosophila melanogasterProceedings of the Royal Society B: Biological Sciences 284:20172237.https://doi.org/10.1098/rspb.2017.2237
-
The role of mitochondria in Drosophila agingExperimental Gerontology 46:331–334.https://doi.org/10.1016/j.exger.2010.08.010
-
Sex comes in from the cold: the integration of sex and patternTrends in Genetics 18:510–516.https://doi.org/10.1016/S0168-9525(02)02769-5
-
Two closely linked mutations in Drosophila melanogaster that are lethal to opposite sexes and interact with daughterlessGenetics 90:683–698.
-
Vive la différence: males vs females in flies vs wormsAnnual Review of Genetics 30:637–702.https://doi.org/10.1146/annurev.genet.30.1.637
-
The roles of fruitless and doublesex in the control of male courtshipInternational Review of Neurobiology 99:87–105.https://doi.org/10.1016/B978-0-12-387003-2.00004-5
-
How flies get their size: genetics meets physiologyNature Reviews Genetics 7:907–916.https://doi.org/10.1038/nrg1989
-
ALLOMETRY FOR SEXUAL SIZE DIMORPHISM:pattern and process in the coevolution of body size in males and femalesAnnual Review of Ecology and Systematics 28:659–687.https://doi.org/10.1146/annurev.ecolsys.28.1.659
-
Phenotypic plasticity and experimental evolutionJournal of Experimental Biology 209:2344–2361.https://doi.org/10.1242/jeb.02244
-
Remote control of insulin secretion by fat cells in DrosophilaCell Metabolism 10:199–207.https://doi.org/10.1016/j.cmet.2009.08.002
-
Aberrant splicing and altered spatial expression patterns in fruitless mutants of Drosophila melanogasterGenetics 154:725–745.
-
Insulin signalling underlies both plasticity and divergence of a reproductive trait in DrosophilaProceedings of the Royal Society B: Biological Sciences 281:20132673.https://doi.org/10.1098/rspb.2013.2673
-
Insulin/TOR signaling in growth and homeostasis: a view from the fly worldThe International Journal of Biochemistry & Cell Biology 41:1006–1010.https://doi.org/10.1016/j.biocel.2008.10.010
-
Regulation of tissue growth through nutrient sensingAnnual Review of Genetics 43:389–410.https://doi.org/10.1146/annurev-genet-102108-134815
-
Sex differences in the response to dietary restriction in rodentsCurrent Opinion in Physiology 6:28–34.https://doi.org/10.1016/j.cophys.2018.03.008
-
Unravelling the diversity of mechanisms through which nutrition regulates body size in insectsCurrent Opinion in Insect Science 25:1–8.https://doi.org/10.1016/j.cois.2017.11.002
-
Regulation of adult female germline stem cells by nutrient-responsive signalingCurrent Opinion in Insect Science 37:16–22.https://doi.org/10.1016/j.cois.2019.10.005
-
Sex differences in the effect of dietary restriction on life span and mortality rates in female and male Drosophila melanogasterThe Journals of Gerontology Series A: Biological Sciences and Medical Sciences 59:B3–B9.https://doi.org/10.1093/gerona/59.1.B3
-
Gender differences in glucose homeostasis and diabetesPhysiology & Behavior 187:20–23.https://doi.org/10.1016/j.physbeh.2017.08.016
-
Sex differences in Drosophila development and physiologyCurrent Opinion in Physiology 6:46–56.https://doi.org/10.1016/j.cophys.2018.04.002
-
Matching complex dietary landscapes with the signalling pathways that regulate life history traitsCurrent Opinion in Genetics & Development 47:9–16.https://doi.org/10.1016/j.gde.2017.08.001
-
The control of body size in insectsDevelopmental Biology 261:1–9.https://doi.org/10.1016/S0012-1606(03)00276-8
-
The developmental control of size in insectsWiley Interdisciplinary Reviews: Developmental Biology 3:113–134.https://doi.org/10.1002/wdev.124
-
Characterization of Drosophila insulin receptor substrateJournal of Biological Chemistry 275:23346–23354.https://doi.org/10.1074/jbc.M003579200
-
Transcriptional feedback control of insulin receptor by dFOXO/FOXO1Genes & Development 19:2435–2446.https://doi.org/10.1101/gad.1340505
-
Gender and longevity: why do men die earlier than women? comparative and experimental evidenceBest Practice & Research Clinical Endocrinology & Metabolism 27:467–479.https://doi.org/10.1016/j.beem.2013.05.016
-
Insulin signaling is necessary for vitellogenesis in Drosophila melanogaster independent of the roles of juvenile hormone and ecdysteroids: female sterility of the chico1 insulin signaling mutation is autonomous to the ovaryJournal of Insect Physiology 51:455–464.https://doi.org/10.1016/j.jinsphys.2004.12.013
-
Studies in quantitative inheritance X. genetic variation of ovary size in DrosophilaJournal of Genetics 55:410–427.https://doi.org/10.1007/BF02984060
-
The roles of cell size and cell number in determining ovariole number in DrosophilaDevelopmental Biology 363:279–289.https://doi.org/10.1016/j.ydbio.2011.12.017
-
Fiji: an open-source platform for biological-image analysisNature Methods 9:676–682.https://doi.org/10.1038/nmeth.2019
-
Insulin-Like peptides regulate feeding preference and metabolism in DrosophilaFrontiers in Physiology 9:1083.https://doi.org/10.3389/fphys.2018.01083
-
The sex-specific effects of diet quality versus quantity on morphology in Drosophila melanogasterRoyal Society Open Science 4:170375.https://doi.org/10.1098/rsos.170375
-
Integration of taste and calorie sensing in DrosophilaJournal of Neuroscience 32:14767–14774.https://doi.org/10.1523/JNEUROSCI.1887-12.2012
-
A gene in Drosophila melanogaster That Transforms Females into MalesGenetics 30:297–299.
-
Molecular mechanisms of metabolic regulation by insulin in DrosophilaBiochemical Journal 425:13–26.https://doi.org/10.1042/BJ20091181
-
FlyBase 2.0: the next generationNucleic Acids Research 47:D759–D765.https://doi.org/10.1093/nar/gky1003
-
Mitochondrial maintenance failure in aging and role of sexual dimorphismArchives of Biochemistry and Biophysics 576:17–31.https://doi.org/10.1016/j.abb.2014.10.008
-
Sex-Specific gene expression and life span regulationTrends in Endocrinology & Metabolism 28:735–747.https://doi.org/10.1016/j.tem.2017.07.002
-
Cell-autonomous regulation of cell and organ growth in Drosophila by akt/PKBNature Cell Biology 1:500–506.https://doi.org/10.1038/70293
Article and author information
Author details
Funding
Canadian Institutes of Health Research (PJT-153072)
- Elizabeth J Rideout
Natural Sciences and Engineering Research Council of Canada (RGPIN-2016-04249)
- Elizabeth J Rideout
Michael Smith Foundation for Health Research (16876)
- Elizabeth J Rideout
Canada Foundation for Innovation (JELF-34879)
- Elizabeth J Rideout
H2020 European Research Council (ERCAdG787470)
- Irene Miguel-Aliaga
European Molecular Biology Organization (aALTF782-2015)
- Bruno Hudry
University of British Columbia (CELL Fellowship)
- Jason W Millington
University of British Columbia (British Columbia Graduate Scholarship Award)
- Lianna W Wat
NSERC (Undergraduate Student Research Award)
- Ziwei Sun
MRC Intramural funding
- Irene Miguel-Aliaga
The funders had no role in study design, data collection and interpretation, or the decision to submit the work for publication.
Acknowledgements
We thank Dr. William Ja for the UAS-sun strain (Delanoue et al., 2016), Dr. Pierre Léopold for the anti-Sun antibody (Delanoue et al., 2016), Dr. Suzanne Eaton and Dr. Natalie Dye for the anti-Cv-d antibody (Palm et al., 2012), Dr. Bruce Edgar for the GFP-PH reporter (tGPH), and Dr. Linda Partridge for sharing the dilp2 mutant strain. Stocks obtained from the Bloomington Drosophila Stock Center (NIH P40OD018537) were used in this study. We thank the TRiP at Harvard Medical School (NIH/NIGMS R01-GM084947) for providing transgenic RNAi fly stocks and/or plasmid vectors used in this study. Transgenic fly stocks and/or plasmids were also obtained from the Vienna Drosophila Resource Center (VDRC, http://www.vdrc.at). We acknowledge critical resources and information provided by FlyBase (Thurmond et al., 2019) FlyBase is supported by a grant from the National Human Genome Research Institute at the U.S. National Institutes of Health (U41 HG000739) and by the British Medical Research Council (MR/N030117/1). Funding for this study was provided by grants to EJR from the Canadian Institutes for Health Research (PJT-153072), Natural Sciences and Engineering Research Council of Canada (NSERC, RGPIN-2016–04249), Michael Smith Foundation for Health Research (16876), and the Canadian Foundation for Innovation (JELF-34879), and to IMA from the European Research Council (ERCAdG787470) and MRC Intramural Funding. JWM was supported by a 4 year CELL Fellowship from UBC, LWW was supported by a British Columbia Graduate Scholarship Award, ZS was supported by an NSERC Undergraduate Student Research Award, and BH was supported by an European Molecular Biology Organization Fellowship (aALTF782-2015). We would like to acknowledge that our research takes place on the traditional, ancestral, and unceded territory of the Musqueam people; a privilege for which we are grateful.
Copyright
© 2021, Millington et al.
This article is distributed under the terms of the Creative Commons Attribution License, which permits unrestricted use and redistribution provided that the original author and source are credited.
Metrics
-
- 3,670
- views
-
- 519
- downloads
-
- 39
- citations
Views, downloads and citations are aggregated across all versions of this paper published by eLife.
Citations by DOI
-
- 39
- citations for umbrella DOI https://doi.org/10.7554/eLife.58341