Alcohol potentiates a pheromone signal in flies
Abstract
For decades, numerous researchers have documented the presence of the fruit fly or Drosophila melanogaster on alcohol-containing food sources. Although fruit flies are a common laboratory model organism of choice, there is relatively little understood about the ethological relationship between flies and ethanol. In this study, we find that when male flies inhabit ethanol-containing food substrates they become more aggressive. We identify a possible mechanism for this behavior. The odor of ethanol potentiates the activity of sensory neurons in response to an aggression-promoting pheromone. Finally, we observed that the odor of ethanol also promotes attraction to a food-related citrus odor. Understanding how flies interact with the complex natural environment they inhabit can provide valuable insight into how different natural stimuli are integrated to promote fundamental behaviors.
Introduction
Conflict that results in aggression occurs across the animal kingdom. Aggression in Drosophila melanogaster is a well-documented behavior; studies have identified several aggression-regulating pheromones, circuits, and genes (Wang and Anderson, 2010; Vrontou et al., 2006; Nilsen et al., 2004; Asahina et al., 2014; Dow and von Schilcher, 1975). The most well-studied pheromone, 11-cis-Vaccenyl acetate (cVa) is produced by males and has been shown to increase aggression in male flies (Wang and Anderson, 2010). Most studies of cVa use this pheromone in isolation by adding it to a behavioral arena or painting flies with it. However, in the wild, flies will encounter cVa when they aggregate on fermenting fruits where they will experience cVa in combination with volatile compounds produced by fermentation (Zhu et al., 2003; Keesey et al., 2016). Despite the ecological complexity of the fruit fly niche, little is understood about how ethologically relevant combinations of odors influence the underlying neurobiology of behavior.
Ethanol is one of the products of fermentation and fruit flies in particular are attracted to alcohol-containing fruits (<7% ethanol by volume) (McKenzie and McKechnie, 1979). Remarkably, there is no identified canonical ethanol olfactory receptor despite the fact that a wide range of ethanol-related behaviors have been identified in flies (reviewed in Park et al., 2017). Kim et al., 1998 identified an odorant-binding protein known as LUSH that mediates aversion to high concentrations of alcohol. LUSH was the first protein shown to directly bind ethanol (Kruse et al., 2003). In the fly antennae, the only neurons known to respond to cVa are also those that express LUSH (Xu et al., 2005). However, these neurons do not show any electrophysiological responses to ethanol alone (Figure 1a and Kim et al., 1998). We hypothesized that these neurons could respond to the combination of ethanol and cVa. Here, we ask if ethanol potentiates the cVa signal to enhance inter-male aggression.
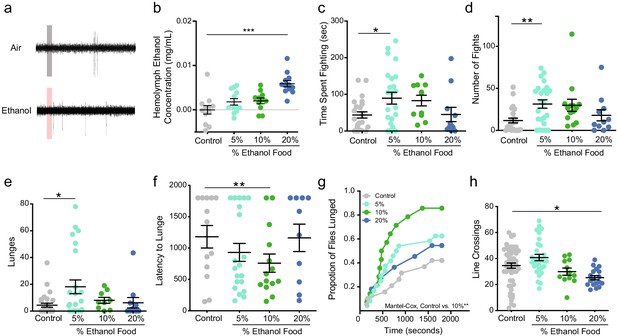
Alcohol odor increases aggression in male flies.
(a) Traces of T1 sensilla recordings with a 300 ms exposure to air or vapor from 30% ethanol. (b) Hemolymph ethanol concentration (mg/mL) in flies in aggression arenas for 30 mins show no significant increases except with 20% ethanol (one-way ANOVA with Dunnett’s p<0.0001, n = 11–12). (c) Time spent fighting on ethanol-containing food (Control vs. 5% p=0.038 Kruskal-Wallis test with Dunn’s correction, n = 10–20). (d) Number of fights on ethanol-containing food (Control vs. 5% p=0.0012, statistical tests as in c). (e) Lunges on ethanol-containing food (Control vs. 5% p=0.0225, statistical tests as in c). (f) Latency to lunge (p=0.009, Log-rank Mantel-Cox with Bonferroni correction). (g) Cumulative latency of flies that lunged during the test (Control vs. 10% p=0.009, Log-rank Mantel-Cox with Bonferroni correction). (h) Locomotion as measured by line crossings during the test (Control vs. 20% p=0.0048, statistical tests as in 1 c). p<0.05 *; p<0.01 **; p<0.001 ***. Error bars denote SEM.
Using fly aggression arenas, we added ethanol to fly food in amounts of 5%, 10%, and 20% by volume (Mundiyanapurath et al., 2006). To determine the fly’s level of aggression we video recorded two wild-type (Canton-S) males in the arena for 30 min and scored fighting events manually. We catalogued fencing, shoving, boxing, tussling, and lunging as aggressive behaviors (described in Chen et al., 2002 as offensive actions). We measured hemolymph or ‘blood’ ethanol concentration (BEC), after the flies were in the arena for 30 min (Figure 1b), to determine if the flies were receiving an intoxicating dose of ethanol. Neither the 5% nor the 10% ethanol-containing foods caused any detectable rise in ethanol in the flies.
Males that fought on 5% ethanol-containing food exhibited an increase in time spent fighting, number of fights they engaged in, and number of lunges executed (Figure 1c,d and e). Although, males on 10% ethanol food exhibited an increase in the proportion that lunged and decreased latency to lunge, they did not show an increase in total number of lunges executed compared to control animals (Figure 1e,f & g). These data demonstrate that when flies occupy food patches with ethologically relevant concentrations of ethanol, they display elevated levels of aggression that are not due to increased locomotion (Figure 1h). One possible explanation for the reduction of aggression in flies that fought on 20% ethanol compared to those on 5% ethanol can be explained by Wang and Anderson, 2010 observation that males respond to cVa in a dose-dependent manner with low concentrations of cVa promoting aggregation and aggression, whereas high concentrations of cVa cause dispersion (Wang and Anderson, 2010). Twenty-percent ethanol may have potentiated the cVa signal so that it mimics a high cVa concentration causing males to disperse and spend less time fighting.
We sought to determine if ethanol influences the neuronal responses to cVa pheromone by monitoring activity of the T1 cVa sensing neurons in the presence of mixtures of these two odorants. We recorded from cVa-responsive neurons while exposing them to 1% cVa and 5% or 30% ethanol (cVA concentration chosen for comparison to prior literature; ethanol concentrations chosen because they were commonly used in other experiments; Figure 2a). Although ethanol does not acutely activate these neurons, we found that it substantially enhanced their cVa responses (Figure 2b). We found that 30% ethanol substantially increased cVa-evoked activity (Δ Spikes), while 5% ethanol increased activity to a lesser extent (Figure 2c,e,f and g). One possible explanation for why we did not observe an increase in Δ Spikes with cVa and 5% ethanol even though 5% ethanol increased aggression is because the duration of exposure was considerably shorter (1 min) compared to the behavioral assay (30 min), which may not have allowed for sufficient accumulation of alcohol. These T1 neurons have an unstimulated spontaneous firing rate of approximately 1 Hz and increase their firing in response to cVa (Xu et al., 2005). Spontaneous responses consistently increased when we applied 5% or 30% ethanol (Figure 2d). Finally, the time constant to deactivation increased with 30% ethanol application indicating that neurons had greater sustained activity following cVa treatment (Figure 2h). These data are consistent with the notion that ethanol increases inter-male aggression by potentiating responses to cVa.
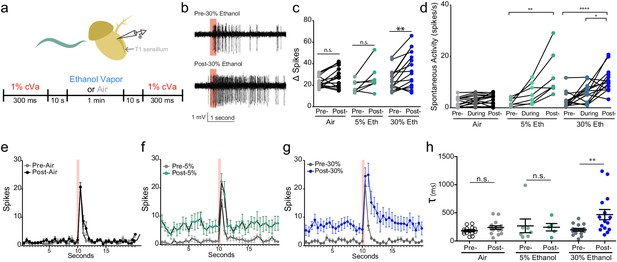
Alcohol odor potentiates the response to cVa.
(a) Experimental timeline and diagram of recording site on fly antenna. (b) Traces of cVa-sensing T1 neurons. The red bar denotes 300 ms cVa exposure. (c) Δ Spikes calculated as cVa-induced activity (1 s during and after cVa) – spontaneous activity (paired two-tailed t-test, p=0.002, n = 7,15,15). (d) Spontaneous activity before, during, and after ethanol exposure. Spontaneous activity calculated as the total number of spikes 10 s prior to cVa delivery/10 s (Pre- vs. Post-5% p=0.002, Pre- vs. Post-30% p<0.0001, During vs. Post-30% p=0.0152, Kruskal-Wallis test with Dunn’s correction). (e), (f), (g) Averaged spikes over time for air, 5% ethanol, and 30% ethanol, respectively. Red bar denotes 300 ms cVa exposure (h) Time constant (τ) of decay of the cVa-induced spikes (Mann-Whitney test, p=0.0043). p>0.05, n.s. (not significant); p<0.05 *; p<0.01 **; p<0.0001 ****. Error bars denote SEM.
In the wild, ethanol is almost always present with other fruit volatiles and fermentation odorants. Farnesol is an odorant present in the rinds of citrus fruits, which are known to be attractive to flies (Ronderos et al., 2014; Dweck et al., 2013). We asked if ethanol would potentiate the electrophysiological response to farnesol and attraction to farnesol. We chose farnesol because Or83c neurons are not broadly tuned and display strong activation to farnesol (similar to how cVa-sensing neurons are only tuned to cVa) and do not show a response to ethanol alone (Ronderos et al., 2014). Farnesol also conveys an entirely different behavioral response from cVa. We performed single-sensillum recordings (SSR) of the ai2 sensilla while exposing them to 30% ethanol and recorded their responses to farnesol (Figure 3a). We found that both evoked activity and spontaneous activity increased following ethanol treatment (Figure 3b-e). To determine if ethanol can augment attraction to farnesol we used two-choice olfactory trap assay (pictured Figure 3f). We used a dilution of farnesol that elicited no attraction when used alone (10−5). However, when combined with 30% ethanol the odor from a 10−5 dilution of farnesol displayed much greater attractiveness than either the odor of farnesol or ethanol alone (Figure 3g). Flies preferred the mixed farnesol and ethanol odor over farnesol alone or ethanol alone suggesting ethanol potentiates attraction to farnesol.
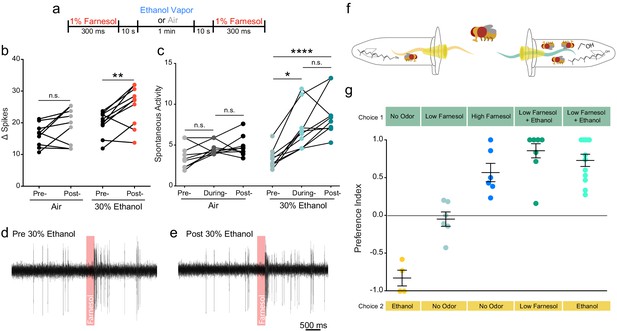
Ethanol increases attraction to and potentiates the neuronal response of a food related odor.
(a) Paradigm used to evaluate farnesol responses pre- and post-ethanol treatment. Responses are shown in panels b-e. (b) Δ Spikes of farnesol-induced activity in ai2 sensilla, calculated as in 2b (paired two-tailed t-test, p=0.0059, n = 9–10). (c) Spontaneous activity before, during, and after ethanol exposure, calculated as in 2 c (Pre- vs. During Ethanol p=0.017, Pre- vs. Post-Ethanol p<0.0001, Kruskal-Wallis test with Dunn’s correction). (d, e) Traces from ai2 farnesol-sensing neurons. The red bar denotes a 300 ms farnesol exposure. (f) Graphic of the two-choice olfactory trap assay used to measure relative attraction to odors. (g) Preference Indices calculated as Number of flies in Choice 1-Number of flies in Choice 2/Total Number of flies. p>0.05, n.s. (not significant); p<0.05 *; p<0.01 **; p<0.0001 ****. Error bars denote SEM.
Previous studies on behavioral responses of Drosophila to ethanol have focused on the systemic effects of ethanol. These studies demonstrated that flies, like mammals, become hyperactive with low doses of ethanol, sedate at high doses of ethanol, acquire tolerance to ethanol, display a withdrawal response, and seek ethanol despite negative consequence (reviewed in Park et al., 2017). However, ethanol responses described in this paper are fundamentally distinct in that they are most likely olfactory (non-systemic) and are ethologically important to the life of a fly. Fischer et al., 2017 demonstrated that flies are more attracted to natural mixtures of microbial by-products than the individual components of the mixture. We find evidence that ethanol potentiates two fundamentally distinct odorants and behaviors in Drosophila. First, ethanol odor increases cVa signaling, which in turn increases aggression. Second, ethanol augments farnesol signaling, resulting in increased attraction to this food odorant. Female flies are thought to prefer to consume ethanol-laden food and lay their eggs in ethanol-containing food because ethanol has caloric value, antimicrobial properties, and provides a protected niche by suppressing competition from other Drosophila species that find the ethanol to be toxic (Kacsoh et al., 2013; Park et al., 2018; Azanchi et al., 2013). When female flies accumulate on the food substrate, males eventually follow, and fight one another for a chance to mate with the female. Interestingly, male flies are known to deposit cVa directly onto food substrates and will spend more time around the marked area (Keesey et al., 2016; Mercier et al., 2018). Both the cVa pheromone and farnesol odorant are naturally encountered by flies in the wild and evoke behaviors believed to provide selective advantages. The odor of ethanol combined with a food odor could enhance the perceived value of the food as a reproductive resource, while the combination of the scent of ethanol and cVA could increase the male drive to fight for control of this resource. We documented evidence for potentiated responses to two odors, but it seems likely that ethanol could increase attraction to other food odors and perhaps other fly pheromones as well.
Materials and methods
Fly handling
Request a detailed protocolAll flies were raised on corn meal malt extract food (7.6%) CH Guenther and Son Pioneer Corn Meal (Walmart, Inc), 7.6% Karo syrup (Walmart, Inc), 1.8% Brewer’s yeast (SAF, Milwaukee WI), 0.9% Gelidium agar (Mooragar, Inc, Rocklin, CA), 0.1% nipagin (Fisher Scientific, Inc) in 0.5% ethanol, 11.1% #5888 amber malt extract (Austin Homebrew, Austin, Tx) and 0.5% proprionic acid (Fisher Scientific, Inc). Solids are weight/volume and liquids are volume/volume. Flies were housed a 12:12 light:dark cycle. Flies used in aggression and courtship receptivity behavioral assays were all taken from group housed bottles as pupae and individually raised in vials. Flies used in imaging, immunohistochemistry, and qPCR were group housed.
Behavioral tests
Request a detailed protocolAggression chambers were assembled based on description by Mundiyanapurath et al., 2006. using a fly vial cut one inch high and glued to one petri dish. The top of the chamber has two holes; one large hole is used for loading flies and one other smaller hole is in the center of the top and is used for circulation. Food wells were made by cutting 1.5 mL microfuge tube tops. Fly food was melted and pipetted into the microfuge tube tops. Ethanol was added into the fly food once the food cooled down to roughly 35°C. We added sucrose to the top of each fly food surface and a decapitated virgin female fly. Flies were loaded into the chamber by gentle aspiration and the video camera began recording 5 min after the flies were in the chamber. Aggression tests were conducted between the hours of 9 AM and 4 PM (Lights on 8 AM – 8PM). Flies tested for aggression were between 4 and 6 days old and Canton S.
Line crossing assays were performed in the aggression chambers. Flies were aspirated into the chamber in pairs and we recorded the total number of line crossings within a 5-min time period. We recorded video from the top of the chambers and drew a line bisecting the chamber. We used Canton S males between 4 and 6 days old.
Olfactory Traps were based on the protocol developed by Woodard et al., 1989 and were constructed using a 1.5-microfuge tube with a hole drilled on the cap. A yellow-tip pipette was then cut to fit in the hole so that the tops were flush against the cap of the microfuge and cut on the bottom so that flies would be able to enter. For the odorants, we cut pieces of Fisherbrand medium porosity filter paper (Hampton, NH, Catalog No. 09-801E) into 2.5 × 2 cm squares. We used Sigma-Aldrich 95% Farnesol (St. Louis, MO) diluted in Paraffin Oil. For the low concentration Farnesol we used 0.1% and for the high concentration of Farnesol we used 10%. For ethanol, we used a 5% (w/v) solution in water. We pipetted 35 µL of each odorant onto the filter paper squares and folded them up into the microfuge tubes. The paper was pushed to the bottom of the tube to prevent obstruction of the yellow tip pipettes. For the tubes with single odorants, we added the solvent in the opposite tube (e.g. Farnesol in Paraffin oil + Ethanol in water vs. Ethanol in water and Paraffin oil). For the no odorant tubes we used both paraffin oil and water. For each test, we used 20 male Canton S flies that were 4–5 days old. We aspirated the flies into the testing arenas and left them for about 12 hr overnight then placed the whole arena in −20°C kill the flies prior to counting the number of flies in each trap.
Ethanol assay kit
Request a detailed protocolWe used a Megazyme Ethanol Assay Kit Cat # K-ETOH (Megazyme, Bray, CO) to measure BACs (limit of detection 0.093 mg/L). About 40 flies were treated with ethanol or air, then homogenized in ddH2O and centrifuged at 10,000 xg for 10 min. The supernatant was taken and used to measure ethanol concentrations. A negative control without fly homogenate was also used. For concentration calculations, all flies were estimated to contain 1 µL of water (calculated from previous data in Park et al., 2018).
Single sensillum recording electrophysiology
Request a detailed protocolSingle Sensillum extracellular electrophysiology was conducted according to de Bruyne et al., 1999 using 3–5 days old w1118 flies. Flies were assayed under a constant stream of charcoal filtered air (36 ml/min, 22–25°C) to prevent any contamination from environmental odors. cVa was diluted in paraffin oil (1% dilution); 35 μl was applied to filter paper and inserted into a Pasteur pipette; air was passed over the filter and presented as the stimulus. We used 1% cVa because the responses it evokes is most functionally relevant, as the magnitude of response it evokes is similar to exposing a virgin female to a male fly. Signals were amplified 1000x, fed into a computer via a 16-bit analog-to-digital converter (USB-IDAC system; Syntech), and analyzed offline with AUTOSPIKE software. The low cut-off filter setting was 200 Hz and the high cut-off setting was 3 kHz. Action potentials were recorded by inserting a glass electrode in the base of a sensillum. Data analysis was performed as reported by Xu et al., 2005. Signals were recorded starting 10 s before odorant stimulation. cVa-evoked action potentials were counted by subtracting the number of spikes 1 s before cVa stimulation from the spike number 1 s after cVa stimulation (Spikes/sec). The recordings were performed from separate sensilla with a maximum of two sensilla recorded from any single fly.
Ethanol was delivered by adding it into the conical flask that feeds into the IDAC. The ethanol was made from 200 proof ethanol and diluted in ddH2O to make either 30% or 5% ethanol (w/v) and always covered with parafilm. For acute treatments, the flow rate was roughly 36 ml/min, 22–25°C at roughly 2.5 L /min.
Spontaneous Activity = Total number of spikes 10 s prior to cVa delivery/10 s and ΔSpikes = Evoked activity (1 s during and after cVa delivery) – Spontaneous Activity.
Statistical methods
Request a detailed protocolFor data with multiple comparisons we used a One-way ANOVA with Dunnett’s correction for multiple comparisons. To test for normality of the data we used a Shapiro-Wilk’s test. If one of the datasets contained non-normally distributed data we used a Kruskal-Wallis test with Dunn’s correction.
Data availability
All data generated or analysed during this study are included in the manuscript and supporting files.
References
-
Odor coding in a model olfactory organ: the Drosophila maxillary palpThe Journal of Neuroscience 19:4520–4532.https://doi.org/10.1523/JNEUROSCI.19-11-04520.1999
-
Olfactory preference for egg laying on Citrus substrates in DrosophilaCurrent Biology 23:2472–2480.https://doi.org/10.1016/j.cub.2013.10.047
-
Adult frass provides a pheromone signature for Drosophila feeding and aggregationJournal of Chemical Ecology 42:739–747.https://doi.org/10.1007/s10886-016-0737-4
-
LUSH odorant-binding protein mediates chemosensory responses to alcohols in Drosophila melanogasterGenetics 150:711–721.
-
Structure of a specific alcohol-binding site defined by the odorant binding protein LUSH from Drosophila melanogasterNature Structural & Molecular Biology 10:694–700.https://doi.org/10.1038/nsb960
-
Olfactory Landmark-Based communication in interacting DrosophilaCurrent Biology 28:2624–2631.https://doi.org/10.1016/j.cub.2018.06.005
-
Studying aggression in Drosophila (fruit flies)Journal of Visualized Experiments 25:155.https://doi.org/10.3791/155
-
Genetics and genomics of alcohol responses in DrosophilaNeuropharmacology 122:22–35.https://doi.org/10.1016/j.neuropharm.2017.01.032
-
Farnesol-detecting olfactory neurons in DrosophilaJournal of Neuroscience 34:3959–3968.https://doi.org/10.1523/JNEUROSCI.4582-13.2014
-
Fruitless regulates aggression and dominance in DrosophilaNature Neuroscience 9:1469–1471.https://doi.org/10.1038/nn1809
-
Genetic analysis of olfactory behavior in Drosophila: a new screen yields the ota mutantsGenetics 123:315–326.
-
Identification of odors from overripe mango that attract vinegar flies, Drosophila melanogasterJournal of Chemical Ecology 29:899–909.https://doi.org/10.1023/a:1022931816351
Article and author information
Author details
Funding
National Institute on Alcohol Abuse and Alcoholism (2R01AA01803706A1)
- Nigel S Atkinson
National Institute on Alcohol Abuse and Alcoholism (F31AA027160)
- Annie Park
National Institute on Alcohol Abuse and Alcoholism (T32AA07471)
- Annie Park
National Institutes of Health (R01DC015230)
- Dean P Smith
National Institutes of Health (5T32GM008203)
- Elizabeth A Scheuermann
The funders had no role in study design, data collection and interpretation, or the decision to submit the work for publication.
Copyright
© 2020, Park et al.
This article is distributed under the terms of the Creative Commons Attribution License, which permits unrestricted use and redistribution provided that the original author and source are credited.
Metrics
-
- 3,568
- views
-
- 386
- downloads
-
- 9
- citations
Views, downloads and citations are aggregated across all versions of this paper published by eLife.
Citations by DOI
-
- 9
- citations for umbrella DOI https://doi.org/10.7554/eLife.59853