The SWELL1-LRRC8 complex regulates endothelial AKT-eNOS signaling and vascular function
Abstract
The endothelium responds to numerous chemical and mechanical factors in regulating vascular tone, blood pressure, and blood flow. The endothelial volume-regulated anion channel (VRAC) has been proposed to be mechanosensitive and thereby sense fluid flow and hydrostatic pressure to regulate vascular function. Here, we show that the leucine-rich repeat-containing protein 8a, LRRC8A (SWELL1), is required for VRAC in human umbilical vein endothelial cells (HUVECs). Endothelial LRRC8A regulates AKT-endothelial nitric oxide synthase (eNOS) signaling under basal, stretch, and shear-flow stimulation, forms a GRB2-Cav1-eNOS signaling complex, and is required for endothelial cell alignment to laminar shear flow. Endothelium-restricted Lrrc8a KO mice develop hypertension in response to chronic angiotensin-II infusion and exhibit impaired retinal blood flow with both diffuse and focal blood vessel narrowing in the setting of type 2 diabetes (T2D). These data demonstrate that LRRC8A regulates AKT-eNOS in endothelium and is required for maintaining vascular function, particularly in the setting of T2D.
Introduction
The endothelium integrates mechanical and chemical stimuli to regulate vascular tone, angiogenesis, blood flow, and blood pressure (Cahill and Redmond, 2016). Endothelial cells express a variety of mechanosensitive and mechanoresponsive ion channels that regulate vascular function (Gerhold and Schwartz, 2016), including TRPV4 (Sonkusare et al., 2012; Earley et al., 2009; Zhang et al., 2009; Mendoza et al., 2010) and Piezo1 (Rode et al., 2017; Li et al., 2014; Coste et al., 2010). The volume-regulated anion channel (VRAC) current is also prominent in endothelium and has been proposed to be mechanoresponsive (Nilius and Droogmans, 2001), to activate in response to fluid flow/hydrostatic pressure (Barakat et al., 1999) and to putatively regulate vascular reactivity. However, the molecular identity of this endothelial ion channel has remained a mystery for nearly two decades.
Lrrc8a (leucine-rich repeat-containing protein 8A, also known as SWELL1) encodes a transmembrane protein first described as the site of a balanced translocation in an immunodeficient child with agammaglobulinemia and absent B-cells (Sawada et al., 2003; Kubota et al., 2004). Subsequent work revealed the mechanism for this condition to be due to impaired LRRC8A-dependent GRB2-PI3K-AKT signaling in lymphocytes, resulting in a developmental block in lymphocyte differentiation (Kumar et al., 2014). Thus, for ~11 years, LRRC8A was conceived of as a membrane protein that regulates PI3K-AKT mediated lymphocyte function (Sawada et al., 2003; Kubota et al., 2004). Although LRRC8A had been predicted to form a hetero-hexameric ion channel complex with other LRRC8 family members (Abascal and Zardoya, 2012), it was not until 2014 that LRRC8A was shown to form an essential component of the volume-regulated anion channel (VRAC) (Qiu et al., 2014; Voss et al., 2014), forming hetero-hexamers with LRRC8b-e (Voss et al., 2014; Syeda et al., 2016). Therefore, historically, LRRC8A was first described as a membrane protein that signaled via protein-protein interactions and then later found to form an ion channel signaling complex.
We showed previously that LRRC8A is an essential component of VRAC in adipocytes (Zhang et al., 2017) and skeletal myotubes (Kumar et al., 2020), which is required for insulin-PI3K-AKT signaling (Zhang et al., 2017; Kumar et al., 2020) to mediate adipocyte hypertrophy (Zhang et al., 2017), skeletal myotube differentiation (Kumar et al., 2020), skeletal muscle function (Kumar et al., 2020), and systemic glucose homeostasis (Zhang et al., 2017; Kumar et al., 2020; Xie et al., 2017; Gunasekar et al., 2019). The PI3K-AKT-endothelial nitric oxide synthase (eNOS) signaling pathway is central to transducing both mechanical stretch (Hu et al., 2013) and hormonal inputs (insulin) to regulate eNOS expression and activity, which, in turn, regulates vasodilation (blood flow and pressure), inhibits leukocyte aggregation, and limits proliferation of vascular smooth muscle cells (atherosclerosis). Indeed, insulin resistance is thought to be a systemic disorder in the setting of type 2 diabetes (T2D), affecting endothelium in addition to traditional metabolically important tissues, such as adipose, liver, and skeletal muscle (Janus et al., 2016; Kearney et al., 2008; Muniyappa and Sowers, 2013). In fact, insulin-resistant endothelium and the resultant impairment in PI3K-AKT-eNOS signaling have been proposed to underlie much of the endothelial dysfunction observed in the setting of obesity and T2D, predisposing to hypertension, atherosclerosis, and vascular disease (Janus et al., 2016; Kearney et al., 2008; Muniyappa and Sowers, 2013).
In this study, we demonstrate that VRAC is LRRC8A-dependent in endothelium, associates with GRB2, caveolin-1 (Cav1), eNOS, and regulates PI3K-AKT-eNOS signaling and flow-mediated endothelial cell orientation – suggesting that LRRC8 channel complexes link insulin and mechano-signaling in endothelium. LRRC8A-dependent AKT-eNOS, ERK1/2, and mTOR signaling influences blood pressure and vascular function in vivo, while impaired endothelial LRRC8A signaling predisposes to vascular dysfunction in the setting of diet-induced T2D.
Results
Lrrc8a functionally encodes VRAC current in endothelium
The volume-regulatory anion channel (VRAC) current has been measured and characterized in endothelial cells for decades but the molecular identity of this endothelial ion channel remains elusive (Nilius and Droogmans, 2001; Barakat et al., 1999; Barakat, 1999). To determine if the leucine-rich repeat-containing membrane protein LRRC8A recently identified in cell lines (Qiu et al., 2014; Voss et al., 2014) is required for VRAC in endothelial cells, as it is in adipocytes (Zhang et al., 2017), skeletal myoblasts (Kumar et al., 2020) pancreatic ß-cells (Kang et al., 2018; Stuhlmann et al., 2018), nodose neurons (Wang et al., 2017), and spermatozoa (Lück et al., 2018), we first confirmed robust LRRC8A protein expression by Western blot (Figure 1A) and immunostaining (Figure 1B) in human umbilical vein endothelial cells (HUVECs). LRRC8A protein expression is substantially reduced upon adenoviral transduction with a short-hairpin RNA directed to Lrrc8a (Ad-shLrrc8a-mCherry) as compared to a scrambled control (Ad-shScr-mCherry). Next, we measured hypotonically induced (210 mOsm) endothelial VRAC currents in HUVECs. These classic outwardly rectifying hypotonically induced VRAC currents are prominent in HUVECs, largely blocked by the VRAC current inhibitor 4-(2-butyl-6,7-dichloro-2-cyclopentyl-indan-1-on-5-yl) oxobutyric acid (DCPIB; Figure 1C&D), and significantly suppressed upon shLrrc8a-mediated LRRC8A knock-down (KD) (Figure 1E&F), consistent with LRRC8A functionally encoding endothelial VRAC.
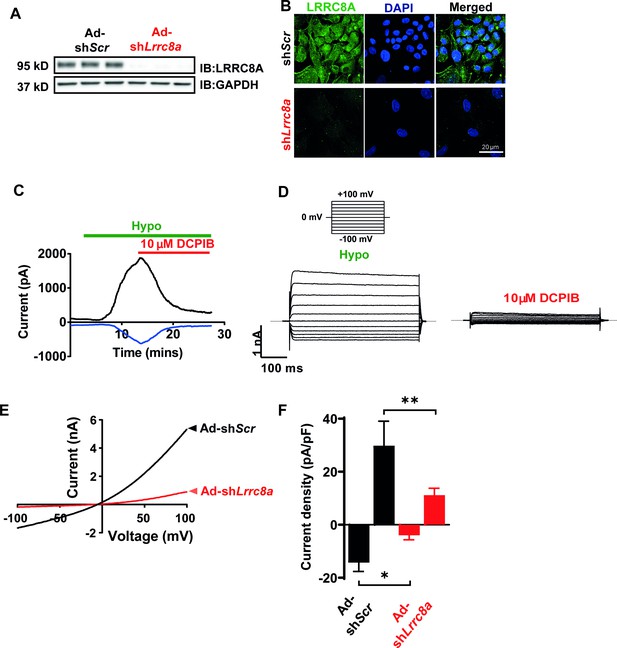
Leucine-rich repeat-containing protein 8a (LRRC8A) mediates volume-regulated anion channel (VRAC) currents in human umbilical vein endothelial cells (HUVECs).
(A) LRRC8A Western blot in HUVECs transduced with adenovirus expressing a short-hairpin RNA directed to LRRC8A (Ad-shLrrc8a) compared to control scrambled short-hairpin RNA (Ad-shScr). GAPDH is used as loading control. (B) Immunofluorescence staining of the HUVECs transduced with Ad-shLrrc8a and Ad-shScr. (C) Current-time relationship of VRAC current (hypotonic, 210 mOsm) in Ad-shScr transduced HUVEC and co-application of 10 µM 4-(2-butyl-6,7-dichloro-2-cyclopentyl-indan-1-on-5-yl) oxobutyric acid (DCPIB). (D) Representative current traces upon hypotonic activation (left) during voltage steps (from -100 to +100 mV, shown in inset) and inhibition by DCPIB (right). (E) Current-voltage relationship of VRAC during voltage ramps from -100 mV to +100 mV after hypotonic swelling in HUVECs transduced with Ad-shScr and Ad-shLrrc8a. (F) Mean current outward and inward densities at +100 and -100 mV (n,shSCR=4 cells; n,shLrrc8a=6 cells). Data are shown as mean ± s.e.m. *p<0.05; **p<0.01; unpaired t-test for (F).
-
Figure 1—source data 1
Source data for Figure 1F.
- https://cdn.elifesciences.org/articles/61313/elife-61313-fig1-data1-v2.xlsx
LRRC8A regulates PI3K-AKT-eNOS, ERK, and mTOR signaling in endothelium
Previous studies in adipocytes (Zhang et al., 2017) and skeletal muscle (Kumar et al., 2020) demonstrate that LRRC8A regulates insulin-PI3K-AKT signaling (Zhang et al., 2017; Kumar et al., 2020), adipocyte expansion (Zhang et al., 2017), skeletal muscle function (Kumar et al., 2020), and systemic glycemia, whereby LRRC8A loss-of-function induces an insulin-resistant pre-diabetic state (Zhang et al., 2017; Kumar et al., 2020; Xie et al., 2017). Insulin signaling is also important in regulating endothelium and vascular function (Kearney et al., 2008; Muniyappa and Sowers, 2013; Duncan et al., 2008). Moreover, insulin resistance in T2D is considered a systemic disorder and insulin-resistant endothelium is postulated to underlie impaired vascular function in T2D (Kearney et al., 2008; Muniyappa and Sowers, 2013). As LRRC8A is highly expressed in endothelium (Figure 1) and PI3K-AKT-eNOS signaling is critical for endothelium-dependent vascular function (Morello et al., 2009), we next examined AKT-eNOS, ERK1/2, and mTOR signaling in LRRC8A KD compared to control HUVECs under basal conditions. Basal phosphorylated AKT2 (pAKT2, Figure 2A&D), pAKT1 (Figure 2A&E), phosphorylated eNOS (p-eNOS) (Figure 2A&C), and pERK1/2 (Figure 2A&F) are abrogated in HUVECs upon LRRC8A KD, indicating that LRRC8A contributes to AKT-eNOS and ERK signaling in endothelium. Curiously, basal pS6 ribosomal protein, indicative of mTOR signaling, is augmented in LRRC8A KD HUVECs compared to control (Figure 2A&G), suggesting LRRC8A to be a negative regulator of mTOR in endothelium. As a complementary approach, we used siRNA mediated LRRC8A KD using a silencer select siRNA targeting Lrrc8a mRNA with a different sequence from shLrrc8a. siRNA mediated LRRC8A KD in HUVECs yielded nearly identical results to the shRNA KD approach (Figure 2—figure supplement 1). In summary, LRRC8A expression level regulates AKT, eNOS, ERK, and mTOR signaling in endothelium.
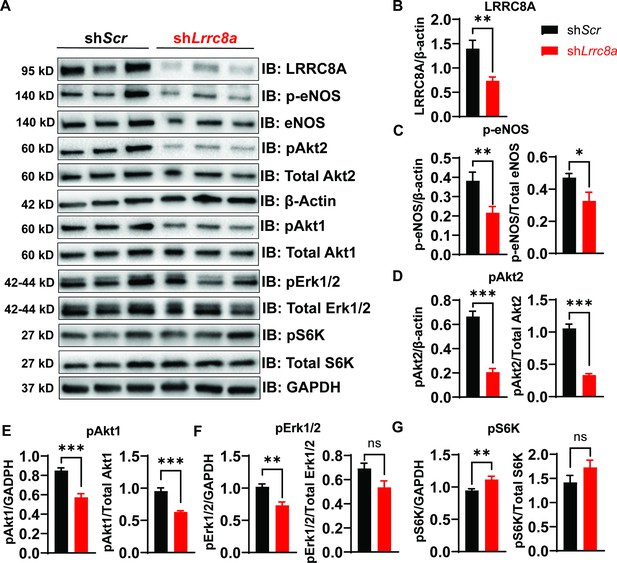
Leucine-rich repeat-containing protein 8a (LRRC8A) regulates PI3K-AKT-endothelial nitric oxide synthase (eNOS), ERK signaling in endothelium.
(A) Western blots of LRRC8A, pAkt2, pAkt1, Akt2, Akt1, pErk1/2, Erk1/2, phosphorylated eNOS (p-eNOS), eNOS, pS6K ribosomal protein, S6K ribosomal protein, GAPDH, and ß-actin in Ad-shScr and Ad-shLrrc8a transduced human umbilical vein endothelial cells (HUVECs) under basal conditions. Quantification of LRRC8A/ß-actin (B), p-eNOS/ß-actin, p-eNOS/total eNOS (C), pAkt2/ß-actin, pAkt2/total Akt2 (D), pAkt1/GAPDH, pAkt1/total Akt1 (E), pERK1/2/GAPDH, pErk1/2/total Erk1/2 (F), pS6 ribosomal protein/GAPDH, and pS6K ribosomal protein/total S6K ribosomal protein (G). N = 6 independent experiments. Significance between the indicated groups in all blots was calculated using a two-tailed unpaired Student’s t-test. Data are shown as mean ± s.e.m. *p<0.05; **p<0.01; ***p<0.001.
-
Figure 2—source data 1
Source data for Figure 2.
- https://cdn.elifesciences.org/articles/61313/elife-61313-fig2-data1-v2.xlsx
LRRC8A interacts with GRB2, Cav1, and eNOS and mediates stretch-dependent eNOS signaling
In adipocytes, the mechanism of LRRC8A-mediated regulation of PI3K-AKT signaling involves LRRC8A/GRB2/Cav1 molecular interactions (Zhang et al., 2017). To determine if LRRC8A resides in a similar macromolecular signaling complex in endothelium, we immunoprecipitated (IP) endogenous GRB2 from HUVECs. Upon GRB2 IP, we detected LRRC8A protein in shScr-treated HUVECs and less LRRC8A upon GRB2 IP from shLrrc8a-treated HUVECs, consistent with an LRRC8A-GRB2 interaction (Figure 3A&B). In addition, with GRB2 IP, we also detected both Cav1 (Figure 3A&B) and eNOS (Figure 3B). These data suggest that endothelial LRRC8A resides in a signaling complex that includes GRB2, Cav1, and eNOS, consistent with the findings that GRB2 and Cav1 interact, and that Cav1 regulates eNOS via a direct interaction (Ju et al., 1997; Venema et al., 1997; Goligorsky et al., 2002). Also, GRB2 has been shown to regulate endothelial ERK, AKT, and JNK signaling (Salameh et al., 2005). Moreover, these data are also in line with the notion that caveoli form mechanosensitive microdomains (Nassoy and Lamaze, 2012; Sinha et al., 2011; Sedding et al., 2005) which regulate VRAC (Trouet et al., 2001; Trouet et al., 1999; Egorov et al., 2019), and VRAC can be activated by mechanical stimuli in a number of cell types, including endothelium (Nilius and Droogmans, 2001; Barakat, 1999; Browe and Baumgarten, 2003; Browe and Baumgarten, 2006; Nakao et al., 1999; Romanenko et al., 2002).
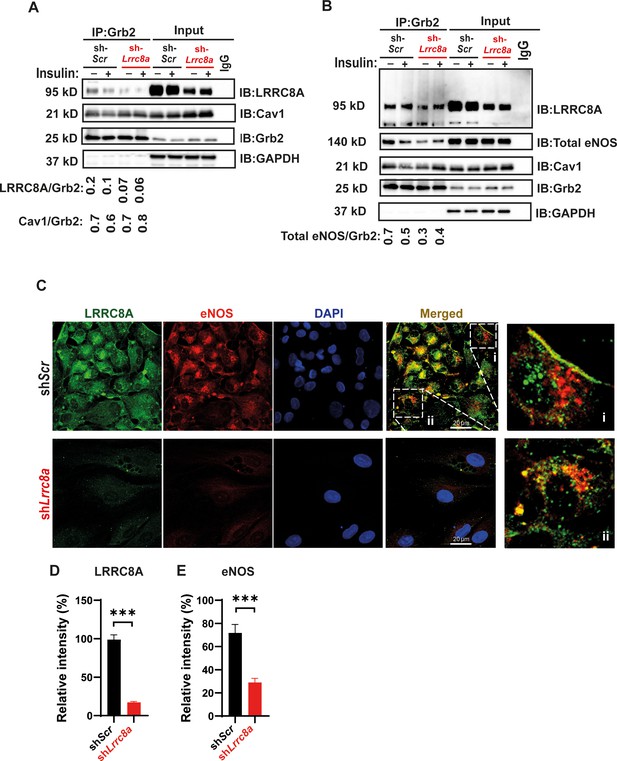
Leucine-rich repeat-containing protein 8A (LRRC8A) interacts with Grb2, Cav1, and endothelial nitric oxide synthase (eNOS) in human endothelium.
(A) GRB2 immunoprecipitation from Ad-shScr and Ad-shLrrc8a transduced human umbilical vein endothelial cells (HUVECs) and immunoblot with LRRC8A, Cav1, and GRB2 antibodies. Densitometry values for GRB2 co-immunoprecipitated LRRC8A (LRRC8A/GRB2) and GRB2 co-immunoprecipitated Cav1 (Cav1/GRB2). GAPDH serves as loading control for input samples. (B) GRB2 immunoprecipitation from Ad-shScr and Ad-shLrrc8a transduced HUVECs and immunoblot with LRRC8A, eNOS, Cav1, and GRB2 antibodies. Insulin stimulation: 100 nM insulin for 10 min. Densitometry values for GRB2 co-immunoprecipitated eNOS (eNOS/GRB2). Representative blots from three independent experiments. (C) Representative endogenous LRRC8A and eNOS immunofluorescence staining in Ad-shScr and Ad-shLrrc8a transduced HUVECs. Representative image from six independent experiments. (D–E) Quantification of LRRC8A (D, n = 6) and eNOS (E, n = 6) immunofluorescence staining upon LRRC8A knock-down (KD). Evidence of LRRC8A-eNOS co-localization (C, insets) in plasma membrane (i) and perinuclear regions (ii). Significance between the indicated groups is calculated using an unpaired two-tailed Student’s t-test. p-Values are indicated on figures. Data are shown as mean ± s.e.m. ***p<0.001.
-
Figure 3—source data 1
Source data for Figure 3D.
- https://cdn.elifesciences.org/articles/61313/elife-61313-fig3-data1-v2.xlsx
-
Figure 3—source data 2
Source data for Figure 3E.
- https://cdn.elifesciences.org/articles/61313/elife-61313-fig3-data2-v2.xlsx
We also examined the relationship between LRRC8A and eNOS protein expression and localization in HUVECs by immunofluorescence (IF) staining (Figure 3C). Similar to observed by Western blot (Figure 2A, Figure 2—figure supplement 1), IF staining revealed that reductions in LRRC8A expression correlate with reduced eNOS expression (Figure 3C–E, Figure 3—figure supplement 1). Moreover, LRRC8A and eNOS co-localization in plasma membrane and perinuclear intracellular domains (Figure 3C, inset) were consistent with the IP data, revealing an LRRC8A-GRB2-Cav-eNOS interaction (Figure 3A&B), and also with the previously described intracellular eNOS localization (Fulton et al., 2002).
Given that endothelial cells respond to stretch stimuli to regulate vascular tone via activation of eNOS (Jufri et al., 2015), we next examined the LRRC8A dependence of stretch-induced AKT, ERK1/2, and eNOS signaling in HUVECs (Figure 4), similar to several previous studies (Hsu et al., 2010; Shi et al., 2007; Liu et al., 2003; Goettsch et al., 2009). Stretch (5%) was sufficient to stimulate AKT1 and AKT2 signaling (Figure 4A–C), though not ERK1/2 signaling (Figure 4D) in HUVECs, and all were blunted in LRRC8A KD HUVECS (Figure 4A–D). Similarly, we observed abrogation of time-dependent p-eNOS signaling with 5% stretch in LRRC8A KD HUVECs compared to control (Figure 4E&F). Taken together, these data position LRRC8A as a regulator stretch-mediated PI3K-AKT-eNOS signaling in endothelium.
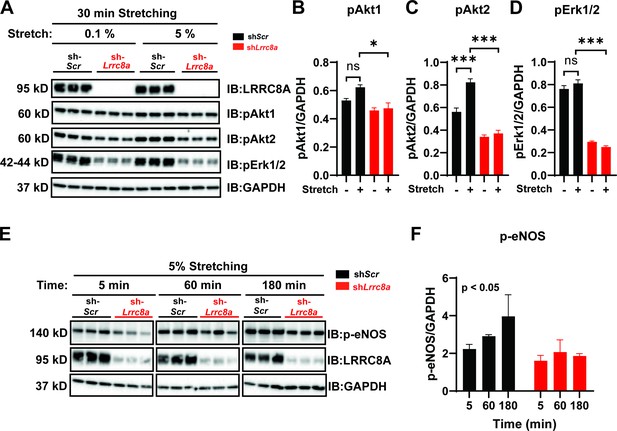
Leucine-rich repeat-containing protein 8a (LRRC8A) is required for intact stretch-induced AKT-endothelial nitric oxide synthase (eNOS) signaling.
(A) Western blot of LRRC8A, pAKT1, pAKT2, pERK1/2 in response to 30 min of 0.1% and 5% static stretch in Ad-shScr (n = 3) and Ad-shLrrc8a (n = 3) transduced human umbilical vein endothelial cells (HUVECs). GAPDH is used as a loading control. (B–D) Densitometry quantification from A of pAKT1 (B), pAKT2 (C), and pErk1/2 (D). (E) Western blot of peNOS, LRRC8A, in response to 5% static stretching for 5, 60, and 180 min in Ad-shScr (n = 3) and Ad-shLrrc8a (n = 3) transduced HUVECs. (F) Densitometry quantification from E of eNOS. GAPDH is used as a loading control. Statistical significance between the indicated group is calculated using one-way analysis of variance (ANOVA), Tukey's multiple comparisons test for (B–D), and two-way ANOVA for (F) with p-value in upper left corner. Data are shown as mean ± s.e.m. *p<0.05; **p<0.01; ***p<0.001.
-
Figure 4—source data 1
Source data for Figure 4F.
- https://cdn.elifesciences.org/articles/61313/elife-61313-fig4-data1-v2.xlsx
-
Figure 4—source data 2
Source data for Figure 4B,C, D.
- https://cdn.elifesciences.org/articles/61313/elife-61313-fig4-data2-v2.xlsx
Endothelial cell orientation to the direction of shear flow is impaired upon LRRC8A KD
To evaluate the requirement of LRRC8A for endothelial cell responses to physiological mechanical stimuli relevant to endothelium, we examined the LRRC8A dependence of HUVEC orientation to laminar shear flow. In response to laminar shear flow at 15 dynes/cm2 for 24 hr (Figure 5A), siControl transfected HUVECs oriented well to the direction of flow (Figure 5B–F and Figure 5—videos 1 and 2), while LRRC8A KD HUVEC exhibited impaired flow-mediated alignment (Figure 5B–F and Figure 5—videos 3 and 4). These LRRC8A-dependent differences were also apparent in the degree of impairment of HUVEC elongation to the direction of fluid flow (Figure 5G) and in the velocity of movement against the direction of flow (Figure 5H&I).
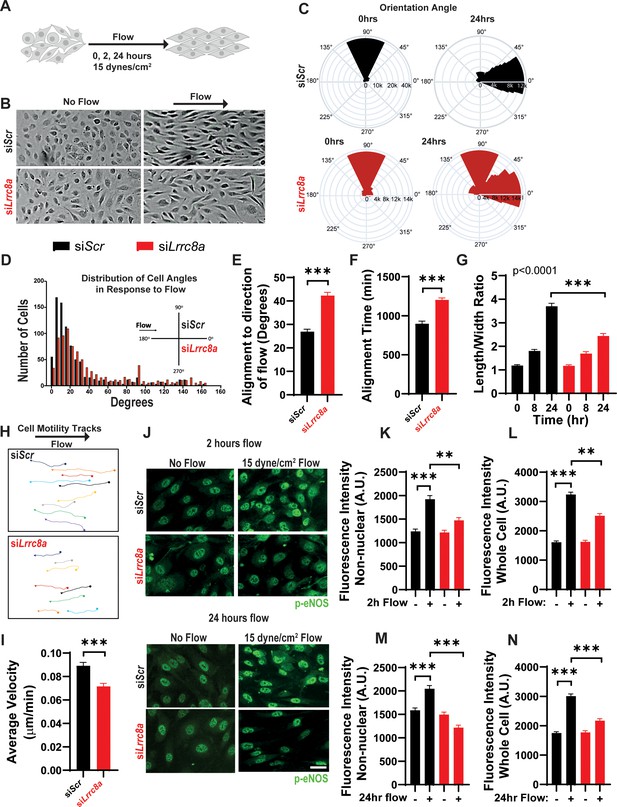
Leucine-rich repeat-containing protein 8a (LRRC8A) depletion impairs human umbilical vein endothelial cell (HUVEC) alignment to the direction of shear flow.
(A) Cartoon of depicting the laminar shear flow applied to HUVEC at 15 dyne/cm2 for 0, 2, and 24 hr. (B) Bright field image of HUVECs transfected with siScr (top) and siLrrc8a (bottom) with no flow (left) and after 24 hr of flow (right). (C) Distributions of angle of HUVEC orientation relative to the direction of flow at 0 hr and after 24 hr of laminar shear flow in siScr-treated cells (top, 0 hr: n = 800; 24 hr: n = 800) and siLrrc8a treated cells (bottom, 0 hr: n = 600; 24 hr: 800). Cuboidal or symmetrical cells at 0 hr were measured as 90o to the direction of flow. (D) Distributions of angle of HUVEC orientation relative to the direction of flow at 24 hr siScr (black, n = 803) and siLrrc8a treated cells (red, n = 803). (E) Mean alignment to direction of flow of siScr (black, n = 803) and siLrrc8a treated cells (red, n = 803). (F) Mean time required for HUVEC to align to within 20o of the direction of shear flow in siScr (black, n = 110) and siLrrc8a treated cells (red, n = 110). (G) Mean HUVEC length-width ratio over time upon stimulation with shear flow in siScr (black, n = 157) and siLrrc8a treated cells (red, n = 157). (H) Representative HUVEC real-time cell tracking during the 24 hr period of shear flow. Arrow heads represent the direction of travel against the direction of shear flow. (I) Average velocity calculated from the mean distance traveled over the 24 hr period of shear flow (nsiScr = 77; nsiLrrc8a = 78). (J) Representative phosphorylated endothelial nitric oxide synthase (p-eNOS) immunofluoresence (green) under conditions of no flow (n = 100), 2 hr (n = 100, top) and no flow (n = 110), 24 hr flow (n = 110, bottom). (K–L) Quantification of non-nuclear p-eNOS immunofluoresence (K) and whole-cell p-eNOS (L) under conditions of no flow (n = 100) and 2 hr flow (n = 100). (M–N) Quantification of non-nuclear p-eNOS immunofluoresence (M) and whole-cell p-eNOS (N) under conditions of no flow (n = 110) and 24 hr flow (n = 110). Statistical significance between the indicated values is calculated using a two-tailed Student’s t-test for (E), (F), and (I), using one-way analysis of variance (ANOVA), Tukey's multiple comparisons test for (K), (L), (M), and (N), and using two-way ANOVA with Bonferroni multiple comparison test in (G). In (G), p<0.001 for the time dependence of siScr versus siLrrc8a treated cells, and p<0.001 for the 24 hr timepoint. Error bars represent mean ± s.e.m. n = 3, independent experiments. **p<0.01 and ***p<0.001.
-
Figure 5—source data 1
Source data for Figure 5E.
- https://cdn.elifesciences.org/articles/61313/elife-61313-fig5-data1-v2.xlsx
-
Figure 5—source data 2
Source data for Figure 5F.
- https://cdn.elifesciences.org/articles/61313/elife-61313-fig5-data2-v2.xlsx
-
Figure 5—source data 3
Source data for Figure 5G.
- https://cdn.elifesciences.org/articles/61313/elife-61313-fig5-data3-v2.xlsx
-
Figure 5—source data 4
Source data for Figure 5I.
- https://cdn.elifesciences.org/articles/61313/elife-61313-fig5-data4-v2.xlsx
-
Figure 5—source data 5
Source data for Figure 5K.
- https://cdn.elifesciences.org/articles/61313/elife-61313-fig5-data5-v2.xlsx
-
Figure 5—source data 6
Source data for Figure 5L.
- https://cdn.elifesciences.org/articles/61313/elife-61313-fig5-data6-v2.xlsx
-
Figure 5—source data 7
Source data for Figure 5M.
- https://cdn.elifesciences.org/articles/61313/elife-61313-fig5-data7-v2.xlsx
-
Figure 5—source data 8
Source data for Figure 5N.
- https://cdn.elifesciences.org/articles/61313/elife-61313-fig5-data8-v2.xlsx
Next, we asked whether shear-flow-mediated eNOS phosphorylation is LRRC8A-dependent, as observed with stretch-mediated signaling (Figure 4). We stimulated siScr and siLrrc8a transfected HUVECs with either no flow, or laminar shear flow for 2 or 24 hr and then immunostained for p-eNOS (Figure 5J). We observed marked p-eNOS induction in response to shear flow after both 2 and 24 hr in siControl transfected HUVECs, and this was significantly abrogated upon LRRC8A KD (Figure 5J). Interestingly, this shear-flow stimulated p-eNOS displayed significant nuclear localization, consistent with prior reports (Feng et al., 1999; Andersson and Brittebo, 2012; Wang et al., 2019; Gobeil et al., 2006). The same nuclear distribution was also observed using a different p-eNOS antibody (Figure 5—figure supplement 1). We quantified the intensity of p-eNOS staining in two ways: p-eNOS excluding the nuclear signal (Figure 5K,L) and p-eNOS in the entire cell (Figure 5M,O). In both cases, we observed significant shear-flow-mediated p-eNOS induction in siScr HUVECs, after both 2 hr and 24 hr flow, and this was reduced in LRRC8A KD HUVEC (Figure 5K–O), consistent with LRRC8A-dependent flow-mediated p-eNOS signaling.
Genome-wide mRNA profiling reveals LRRC8A-dependent regulation of multiple processes involved in angiogenesis, atherosclerosis, and vascular function
Genome-wide transcriptome analysis of LRRC8A KD HUVEC compared to control (RNA sequencing) revealed multiple pathways enriched regulating angiogenesis, migration, and tumorigenesis, including GADD45, IL-8, p70S6K (mTOR), TREM1, angiopoietin, and HGF signaling (Figure 6, Supplementary file 1 and 2). Pathways linked to cell adhesion and renin-angiotensin signaling are also enriched – both pathways and processes that are known to be altered in vasculature in the setting of atherosclerosis and T2D. Also, notable are statistically significant increases in VEGFA (1.6-fold) and CD31 (2.0-fold) expression in LRRC8A KD HUVECs (Figure 6C), both of which are pro-angiogenic and associated with mTORC1 hyperactivation (Ding et al., 2018). CD36 is increased 3.4-fold and is also pro-angiogenic (Silverstein and Febbraio, 2009) and pro-atherosclerotic (Park, 2014; Harb et al., 2009; Kennedy et al., 2009). Moreover, the trends toward reduced eNOS protein expression observed upon LRRC8A KD in HUVECs (Figure 2A, Figure 2—figure supplement 1, Figure 3C&E, Figure 3—figure supplement 1) are also associated with twofold reduced eNOS mRNA expression (NOS3, Figure 6C). Notably, Lrrc8a mRNA expression in HUVECs is higher than many classical endothelial markers expressed in endothelium (EPOR, CDH5, CD36, VWF, PECAM1, KDR, VEGFA, Figure 6C). HUVEC Lrrc8a mRNA expression is also 2.5-fold higher than Piezo1 (Figure 6C), a mechanosensitive ion channel (Coste et al., 2010; Coste et al., 2012) with a well-established role in endothelial biology and vascular function (Rode et al., 2017; Li et al., 2014), and Piezo1 mRNA expression does not change upon LRRC8A KD. Also, Lrrc8a mRNA expression is the highest among all LRRC8 genes expressed in HUVECs, 3-fold higher than Lrrc8a mRNA in 3T3-F442A adipocytes (Zhang et al., 2017), 15-fold higher than C2C12 myotubes (Kumar et al., 2020; Figure 6D), consistent with the prominent endogenous ICl,SWELL currents present in HUVEC (Figure 1C–F).
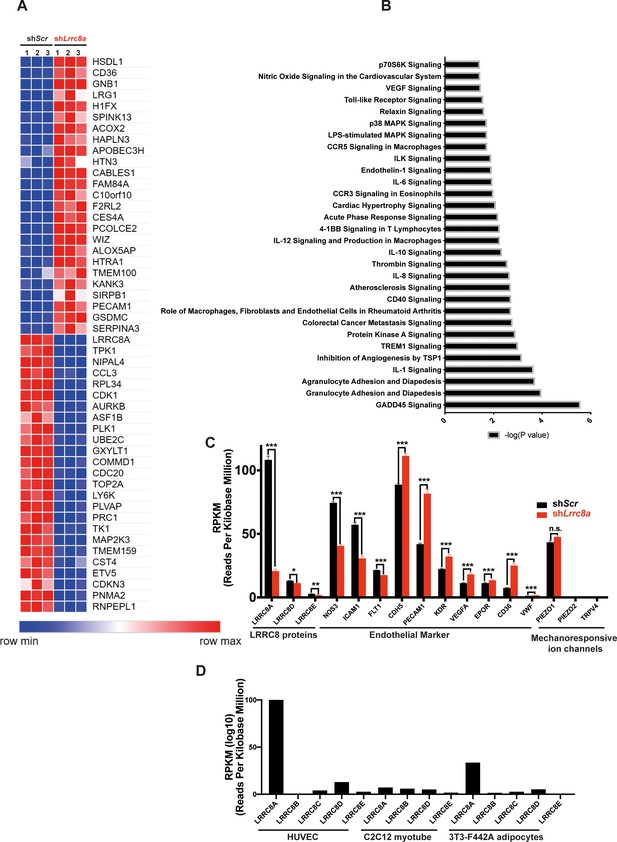
RNA sequencing of Ad-shScr and Ad-shLrrc8a transduced human umbilical vein endothelial cells (HUVECs).
(A) Heatmap analysis displaying top 25 upregulated or 25 downregulated genes between shScr and shLrrc8a. (B) IPA canonical pathway analysis of genes significantly regulated by shLrrc8a in comparison to shScr n = 3 for each group. For analysis with Ingenuity Pathway Analysis (IPA), Fragments Per Kilobase of transcripts per Million mapped reads (FPKM) cutoffs of 1.5, fold change of 1.5, and false discovery rate <0.05 were utilized for significantly differentially regulated genes. (C) Expression levels of select LRRC8 mRNA, classic endothelial markers, and mechanoresponsive ion channels in shScr and shLrrc8a-treated HUVECs. (D) Expression levels LRRC8a-e mRNA in HUVECs compared to data previously published from C2C12 myotubes (Kumar et al., 2020) and differentiated 3T3-F442A adipocytes (Zhang et al., 2017). For (C), statistical significance between the indicated values was calculated using an unpaired two-tailed Student’s t-test. Error bars represent mean ± s.e.m. n = 3, independent experiments. *p<0.05; **p<0.01; ***p<0.001.
-
Figure 6—source data 1
Source data for Figure 6C.
- https://cdn.elifesciences.org/articles/61313/elife-61313-fig6-data1-v2.xlsx
-
Figure 6—source data 2
Source data for Figure 6D.
- https://cdn.elifesciences.org/articles/61313/elife-61313-fig6-data2-v2.xlsx
Endothelial-targeted LRRC8A KO mice exhibit mild angiotensin-II stimulated hypertension and impaired retinal blood flow in the setting of T2D
To examine the functional consequences of endothelial LRRC8A ablation in vivo, we generated endothelial-targeted Lrrc8a knock-out mice (eLrrc8a KO) by crossing Lrrc8a floxed mice (Zhang et al., 2017; Kumar et al., 2020; Kang et al., 2018) with the endothelium-restricted CDH5-Cre mouse (CDH5-Cre; Lrrc8a fl/fl; Figure 7A). Patch-clamp recordings of primary endothelial cells isolated from WT and eLrrc8a KO mice (Figure 7B) reveal robust hypotonically activated currents (Hypo, 210 mOsm) in WT endothelial cells, which are DCPIB inhibited (Figure 7C&E), while eLrrc8a KO endothelial cells exhibit markedly reduced hypotonically activated currents (Figure 7D&E). Consistent with this, IF staining of aortic ring explants from WT and eLrrc8a KO mice confirmed LRRC8A ablation from CD31+ primary endothelial cells (Figure 7F&G).
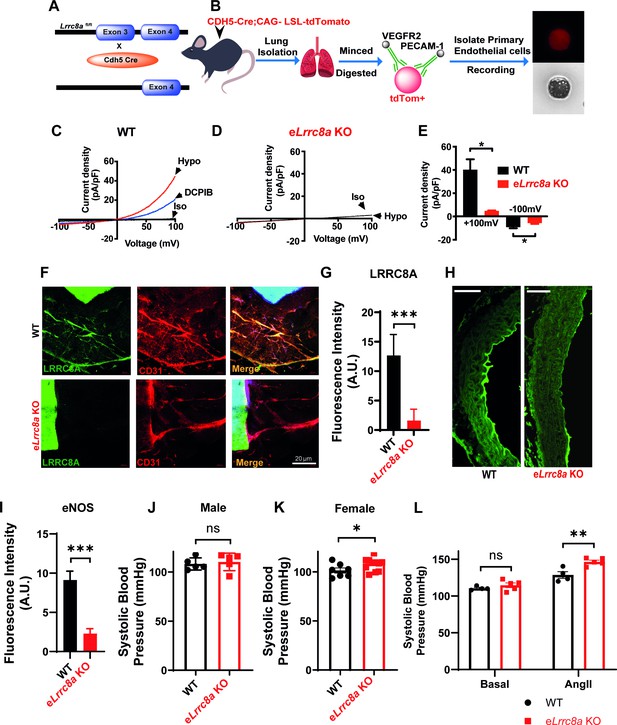
Endothelial-targeted Lrrc8a KO mice exhibit reduced phosphorylated endothelial nitric oxide synthase (p-eNOS) and mild angiotensin-II stimulated hypertension.
(A) Strategy for endothelium targeted Lrrc8a ablation to generate eLrrc8a KO. (B) Isolation of murine primary endothelial cells from WT and eLrrc8a KO using tdTomato reporter mice. (C–D) Current-voltage relationships of volume-regulatory anion channel (VRAC) current in isotonic (Iso, 300 mOsM) and hypotonic (Hypo, 210 mOsm) solution in response to voltage ramps from -100 to +100 mV over 500 ms in WT (C) and KO (D) primary murine endothelial cells. DCPIB (10 µM) inhibition in C (WT). (E) Mean outward (+100 mV) and inward (-100 mV) currents from WT (n = 3 cells) and eLrrc8a KO (n = 3 cells). (F) Ex vivo aorta sprouting assay performed in aortic rings isolated from WT and eLrrc8a KO mice and cultured in FGM media for 3 days at 37°C. Immunofluorescence staining with antibodies to LRRC8A (green), CD31 (red), LRRC8A+CD31+ (Merge) demonstrating endothelial-targeted Lrrc8a deletion. (G) Quantification of LRRC8A immunofluorescence staining in WT (n = 15) and eLrrc8a KO (n = 15) endothelial cell tubes. (H–I) Representative images of aortic sections from WT (n = 3) and eLrrc8a KO (n = 3) mice immunostained with p-eNOS (H, green), and immunofluorescence quantification of regions of WT (n = 5) and eLrrc8a KO (n = 5) endothelium (I). Scale bar in (H) is 50 μm. (J–L) Tail-cuff systolic blood pressures of male (J) and female (K) WT (n = 5 males and 7 females) and eLrrc8a KO (n = 5 males and 12 females) mice, and systolic blood pressures of male WT (n = 4) and eLrrc8a KO (n = 5) mice under basal conditions and after 4 weeks of chronic angiotensin-II infusion (L). Statistical significance between the indicated values is calculated using a two-tailed unpaired Student’s t-test. Error bars represent mean ± s.e.m. *p<0.05; **p<0.01; ***p<0.001.
-
Figure 7—source data 1
Source data for Figure 7E.
- https://cdn.elifesciences.org/articles/61313/elife-61313-fig7-data1-v2.xlsx
-
Figure 7—source data 2
Source data for Figure 7I.
- https://cdn.elifesciences.org/articles/61313/elife-61313-fig7-data2-v2.xlsx
-
Figure 7—source data 3
Source data for Figure 7J.
- https://cdn.elifesciences.org/articles/61313/elife-61313-fig7-data3-v2.xlsx
-
Figure 7—source data 4
Source data for Figure 7K.
- https://cdn.elifesciences.org/articles/61313/elife-61313-fig7-data4-v2.xlsx
-
Figure 7—source data 5
Source data for Figure 7l.
- https://cdn.elifesciences.org/articles/61313/elife-61313-fig7-data5-v2.xlsx
Based on our findings that LRRC8A regulates AKT-eNOS signaling in HUVECs in vitro, we next examined p-eNOS in aortic endothelium by IF staining in WT and LRRC8A KO mice. Similar to results in LRRC8A KD HUVEC, we found reduced p-eNOS intensity in aortic endothelium of eLrrc8a KO mice as compared to WT mice (Figure 7H,I).
Since eNOS signaling is central to blood pressure regulation, we next examined blood pressures in eLrrc8a KO mice compared to WT controls (Lrrc8a fl/fl mice). Male mice exhibited no significant differences in systolic blood pressure under basal conditions (Figure 7J), while female mice were mildly hypertensive relative to WT mice (Figure 7K). However, after 4 weeks of angiotensin-II (Ang II) infusion, male eLrrc8a KO mice developed exacerbated systolic hypertension as compared to Ang II-treated WT mice (Figure 7L). These data are consistent with endothelial dysfunction and impaired vascular relaxation in eLrrc8a KO mice, resulting in a propensity for systolic hypertension.
As endothelial dysfunction may also result in impaired blood flow, we performed retinal imaging during intraperitoneal (i.p.) injection of fluorescein to assess retinal vessel blood flow and morphology in WT and eLrrc8a KO mice. Mice raised on a regular diet had mild, non-significant impairments in retinal blood flow, based on the relative rate of rise of the fluorescein signal in retinal vessels (Figure 8—figure supplement 1). There was evidence of mild focal narrowing of retinal vessels in eLrrc8a KO as compared to WT mice (Figure 8—figure supplement 1A,D&E), with no significant differences in other parameters (Figure 8—figure supplement 1F–K). In mice raised on high-fat high-sucrose (HFHS) diet, retinal blood flow was more severely impaired (Figure 8A–C) with significant focal and diffuse retinal vessel narrowing in eLrrc8a KO mice compared to WT mice (Figure 8A,F–H, Figure 8—figure supplement 2, Figure 8—video 1), and this relative difference was markedly worse in female compared to male mice. These findings are all consistent with endothelial dysfunction and impaired retinal vessel vasorelaxation due to reduced eNOS expression and activity, particularly in the setting of HFHS diet. Also consistent with impaired eNOS activity are reductions in vessel number (Figure 8E), vessel surface area (Figure 8H), number of end points (Figure 8K), branching index (Figure 8L), and increased lacunarity (Figure 8J). These parameters are all suggestive of diabetes-induced retinal vessel dysfunction in the eLrrc8a KO mice, consistent with the loss of eNOS activity that is expected when insulin signaling is compromised (Brooks et al., 2001; Kondo et al., 2003). Notably, both WT and eLrrc8a KO mice were found to be equally glucose-intolerant and insulin-resistant (Figure 8—figure supplement 3), indicating that these differences in microvascular dysfunction were not due to increased hyperglycemia and more severe diabetes in eLrrc8a KO mice. However, while there were no differences in mean body weight or fat mass between WT and eLrrc8a KO males raised on HFHS diet (Figure 8—figure supplement 4), female eLrrc8a KO mice had higher body weights than WT female mice, and this was driven by an increase in total fat mass (Figure 8—figure supplement 4). Taken together, our findings reveal that LRRC8A is highly expressed in endothelium and functionally encodes endothelial VRAC. LRRC8A regulates basal, stretch, and shear-flow-mediated ERK, AKT-eNOS signaling, forms an LRRC8A-GRB2-Cav1-eNOS signaling complex, and regulates vascular function in vivo.
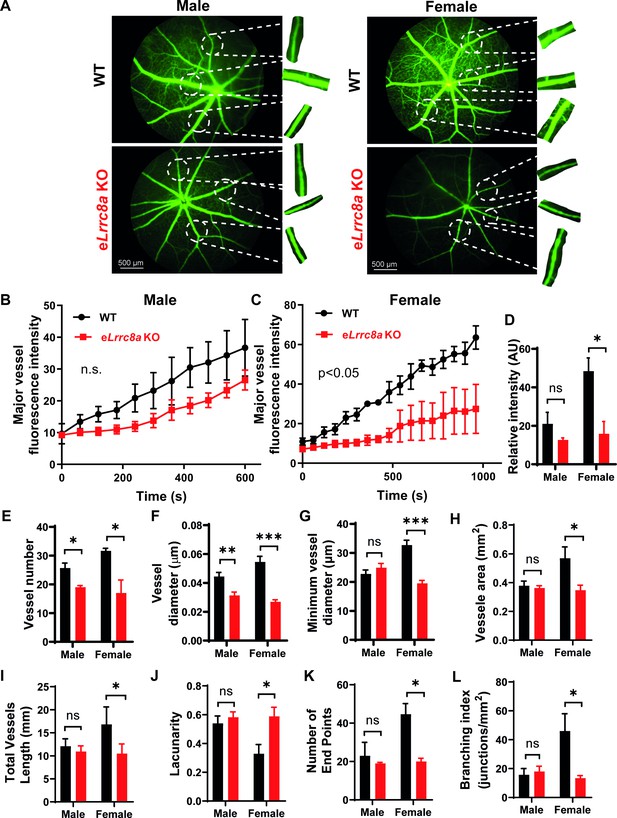
Endothelium-specific Lrrc8a KO mice exhibit exacerbated impairments retinal microvascular disease in the setting of type 2 diabetes.
(A) Representative fluorescein retinal angiograms of WT (top) and eLrrc8a KO (bottom) male (left) and female (right) mice raised on a high-fat high-sucrose (HFHS) diet. Inset shows magnified view of retinal vessels. Quantification of major vessel fluorescence intensity over time after intraperitoneal (i.p.) fluorescein injection in (B) male (n = 3) and (C) female (n = 3) WT and eLrrc8a KO mice. (D–K) Quantification of total retinal vessel intensity (D), total vessel number (E), vessel diameter (F), minimum vessel diameter (G), vessel area (H), total vessel length (I), lacunarity (J), number of end points (K), and branching index (L) of retinal vessels in WT and eLrrc8a KO mice. Statistical significance between the indicated values for B and C was calculated using two-way analysis of variance (ANOVA) and (D–L) using a two-tailed unpaired Student’s t-test. Error bars represent mean ± s.e.m. *p<0.05; **p<0.01; ***p<0.001.
-
Figure 8—source data 1
Source data for Figure 8B.
- https://cdn.elifesciences.org/articles/61313/elife-61313-fig8-data1-v2.xlsx
-
Figure 8—source data 2
Source data for Figure 8C.
- https://cdn.elifesciences.org/articles/61313/elife-61313-fig8-data2-v2.xlsx
-
Figure 8—source data 3
Source data for Figure 8D.
- https://cdn.elifesciences.org/articles/61313/elife-61313-fig8-data3-v2.xlsx
-
Figure 8—source data 4
Source data for Figure 8E.
- https://cdn.elifesciences.org/articles/61313/elife-61313-fig8-data4-v2.xlsx
-
Figure 8—source data 5
Source data for Figure 8F.
- https://cdn.elifesciences.org/articles/61313/elife-61313-fig8-data5-v2.xlsx
-
Figure 8—source data 6
Source data for Figure 8G.
- https://cdn.elifesciences.org/articles/61313/elife-61313-fig8-data6-v2.xlsx
-
Figure 8—source data 7
Source data for Figure 8H.
- https://cdn.elifesciences.org/articles/61313/elife-61313-fig8-data7-v2.xlsx
-
Figure 8—source data 8
Source data for Figure 8I.
- https://cdn.elifesciences.org/articles/61313/elife-61313-fig8-data8-v2.xlsx
-
Figure 8—source data 9
Source data for Figure 8J.
- https://cdn.elifesciences.org/articles/61313/elife-61313-fig8-data9-v2.xlsx
-
Figure 8—source data 10
Source data for Figure 8K.
- https://cdn.elifesciences.org/articles/61313/elife-61313-fig8-data10-v2.xlsx
-
Figure 8—source data 11
Source data for Figure 8L.
- https://cdn.elifesciences.org/articles/61313/elife-61313-fig8-data11-v2.xlsx
Discussion
Our findings demonstrate that the LRRC8 hetero-hexamer functionally encodes endothelial VRAC, whereby LRRC8A associates with GRB2 and Cav1 and positively regulates PI3K-AKT-eNOS and ERK1/2 signaling. LRRC8A depletion in HUVECs reduces pAKT2, pAKT1, p-eNOS, and pERK1/2 under basal conditions, and abrogates both stretch and shear-flow stimulated p-eNOS stimulation. We also discovered the ability of HUVECs to align along the direction of laminar shear flow is markedly impaired in LRRC8A KD cells – suggesting these cells have an impaired ability to either sense or respond to laminar shear flow (or both). These data reveal that LRRC8A-mediated PI3K-AKT signaling is conserved in endothelium, similar to previous observations in adipocytes (Zhang et al., 2017) and skeletal myotubes (Kumar et al., 2020), and in turn positively regulates eNOS expression and activity. Consistent with this mechanism, endothelial-targeted Lrrc8a ablation in vivo predisposes to microvascular dysfunction in the setting of T2D and to hypertension in response to Ang II infusion. These results are in line with the notion that LRRC8A depleted endothelium contributes to an insulin-resistant state in which impaired PI3K-AKT-eNOS signaling results in a propensity for vascular dysfunction (Kearney et al., 2008; Muniyappa and Sowers, 2013). Insulin-mediated regulation of NO is physiologically (Zeng and Quon, 1996; Zeng et al., 2000; Montagnani et al., 2002) and pathophysiologically (Steinberg et al., 1996) important, as NO has vasodilatory (Quillon et al., 2015; Palmer et al., 1987), anti-inflammatory (Kataoka et al., 2002), antioxidant (Clapp et al., 2004), and antiplatelet effects (Schäfer et al., 2004a; Schäfer et al., 2004b; Radomski et al., 1987a; Radomski et al., 1987b). Impaired NO-mediated vascular reactivity is a predictor of future adverse cardiac events (Schächinger et al., 2000) and portends increased risk of atherosclerosis (Bugiardini et al., 2004). Consistent with these NO-mediated effects, the RNA sequencing data derived from LRRC8A KD HUVECs revealed enrichment in inflammatory, cell adhesion, and proliferation pathways (GADD45, IL-8, mTOR, TREM1 signaling) that may arise from LRRC8A-mediated dysregulation of eNOS activity. Moreover, Lrrc8a mRNA is highly expressed in HUVECs relative to adipocyte and myotube cell lines examined previously (Zhang et al., 2017; Kumar et al., 2020), higher than many endothelial genes, and higher than other mechanosensitive (Piezo1) and mechanoresponsive (TRPV4) ion channels with well-established roles in endothelial biology (Sonkusare et al., 2012; Rode et al., 2017; Li et al., 2014; Cappelli et al., 2019; Dalsgaard et al., 2016; Dunn et al., 2013; Earley et al., 2005; Harraz et al., 2018; Mercado et al., 2014; Sonkusare et al., 2014).
In addition to reductions in AKT-eNOS signaling, LRRC8A depletion in HUVECs also reduced ERK1/2 signaling. This decrease in pERK1/2 suggests impaired MAPK signaling which is connected to the insulin receptor by GRB2-SOS. Indeed, we also found that LRRC8A and GRB2 interact in HUVECs, and this may provide the molecular mechanism for the observed defect in ERK signaling. Interestingly, GRB2-MAPK signaling is thought to promote angiogenesis, migration, and proliferation (Zhao et al., 2011), so reductions in ERK1/2 signaling would be predicted to inhibit these processes. One hypothesized mechanism of LRRC8A-mediated regulation of ERK1/2 and AKT2-eNOS signaling involves non-conductive protein-protein signaling via the LRRD as described previously in adipocytes (Zhang et al., 2017; Gunasekar et al., 2019). An alternative hypothesis is that LRRC8A-mediated VRAC channel activity may alter endothelial membrane potential to regulate other endothelial ion channels, such as Piezo1 or TRPV4, and downstream calcium signaling to influence downstream signaling. Future studies will further delineate the molecular mechanisms of LRRC8A regulation of intracellular signaling in endothelial cells and vascular function, and also directly explore whether endothelial LRRC8A-mediated VRAC channel complexes are themselves mechanoresponsive, as suggested in prior studies in other cell types (Browe and Baumgarten, 2003; Browe and Baumgarten, 2006).
Another curious observation that warrants further exploration is the reduction in basal Akt2 and NOS2 (eNOS) mRNA expression upon LRRC8A KD in HUVECs, which is also observed at the protein level. We can only speculate that LRRC8A is somehow regulating Akt2 and eNOS gene expression. Both PI3K and ERK1/2 signaling pathways have been described to regulate total eNOS expression (Wu, 2002), and we observe reductions in both PI3K and ERK1/2 signaling in LRRC8A KD HUVECs, so reductions in eNOS expression are certainly consistent with disruptions in these signaling pathways. Consistent with this conserved biology with respect to LRRC8A signaling, we also observed significant twofold reductions in AKT2 expression upon Lrrc8a deletion in both 3T3-F442A adipocytes (Zhang et al., 2017) and C2C12 myotubes (Kumar et al., 2020), in addition to reductions in NOS2 (iNOS) (Kumar et al., 2020) in adipocytes and NOS1 (neuronal NOS) in C2C12 myotubes (Kumar et al., 2020). Collectively, these findings across multiple cell types support a PI3K and ERK1/2 mediated mechanism of regulation of eNOS and AKT2 gene expression.
The phenotype of endothelial-targeted Lrrc8a KO (eLrrc8a KO) mice are consistent with a reduction in eNOS and p-eNOS as these mice exhibit mild hypertension at baseline (females) and exacerbated hypertension in response to chronic angiotensin infusion, suggesting a modulatory effect on vascular reactivity. Similarly, retinal blood flow is only mildly impaired in eLrrc8a KO mice raised on a regular diet, with some evidence of microvascular disease. However, both retinal blood flow and retinal vessel morphology are markedly impaired in obese-T2D, insulin-resistant eLrrc8a KO mice raised on an HFHS diet compared to controls. This is consistent with a synergistic role of endothelial eLrrc8a ablation and T2D/obesity in the pathogenesis of vascular disease. Our results suggest that reductions in LRRC8A signaling may contribute to impaired vascular function observed in humans in response to insulin and/or shear stress in the setting of obesity (Arcaro et al., 1999; Tack et al., 1998; Westerbacka et al., 1999; Williams et al., 2006) and insulin resistance (Murphy et al., 2007).
Materials and methods
Reagent type (species) or resource | Designation | Source or reference | Identifiers | Additional information |
---|---|---|---|---|
Genetic reagent (Mus musculus) | eLrrc8a KO (Lrrc8afl/fl) | This paper | Sah lab | SWELL1 is a regulator of adipocyte size, insulin signaling, and glucose homeostasis (Zhang et al., 2017) |
Strain, strain background (Mus musculus) | CDH5-Cre | Gift from Dr. Kaikobad Irani | Vikram, A, Nature Communications 2016 | |
Strain, strain background (Mus musculus) | Rosa26-tdTomato | Jackson lab | Jax 007914 RRID:IMSR_JAX:007914 | |
Other | HUVECs | ATCC | Cat#CRL-2922 RRID:CVCL_3901 | Human primary cells |
Biological sample (Mus musculus) | Endothelial primary cell | Lrrc8afl/fl | Freshly isolated from Lrrc8afl/fl mice | |
Antibody | Anti-ß-actin (rabbit monoclonal) | Cell Signaling | Cat#8457s, RRID:AB_10950489 | WB (1:2000) |
Antibody | Anti-total Akt (rabbit monoclonal) | Cell Signaling | Cat#4685s, RRID:AB_2225340 | WB (1:1000) |
Antibody | Anti-Akt1 (rabbit monoclonal) | Cell Signaling | Cat#2938s, RRID:AB_915788 | WB (1:1000) |
Antibody | Anti-p-eNOS (rabbit monoclonal) | Cell Signaling | Cat#9571, RRID:AB_329837 | WB (1:1000) IF (1:200) |
Antibody | Anti-p-eNOS (rabbit polyclonal) | Invitrogen | Cat#PA5-104858, RRID: AB_2816331 | IF (1:200) |
Antibody | Anti-Akt2 (rabbit monoclonal) | Cell Signaling | Cat#3063s, RRID:AB_2225186 | WB (1:1000) |
Antibody | Anti-p-AS160 (rabbit polyclonal) | Cell Signaling | Cat#4288s, RRID:AB_10545274 | WB (1:1000) |
Antibody | Anti-total eNOS (rabbit polyclonal) | Cell Signaling | Cat#32027, RRID:AB_2728756 | WB (1:1000) |
Antibody | Anti-PECAM (rabbit monoclonal) | Sigma | Cat#SAB4502167 RRID:AB_10762600 | WB (1:1000) |
Antibody | Anti-VEGFR2 (goat polyclonal) | R and D Systems | Cat#BAF357 RRID:AB_356414 | WB (1:1000) |
Antibody | Anti- pS6 ribosomal (rabbit monoclonal) | Cell Signaling | Cat#5364s, RRID:AB_10694233 | WB (1:2000) |
Antibody | Anti- GAPDH (rabbit monoclonal) | Cell Signaling | Cat#5174s, RRID:AB_1062202 | WB (1:2000) |
Antibody | Anti-pErk1/2 (rabbit polyclonal) | Cell Signaling | Cat#9101s, RRID:AB_331772 | WB (1:1000) |
Antibody | Anti-Total Erk1/2 (rabbit polyclonal) | Cell Signaling | Cat#9102s, RRID:AB_330744 | WB (1:1000) |
Antibody | Anti-Grb2 (mouse monoclonal) | BD | Cat#610111s, RRID:AB_397517 | WB (1:1000) |
Antibody | Anti-Grb2 (rabbit monoclonal) | Santa Cruz | Cat#sc-255, RRID:AB_631602 | WB (1:1000) |
Antibody | Anti-LRRC8A (rabbit polyclonal) | Pacific antibodies | Custom made | Epitope: QRTKSRIEQGIVDRSE, WB (1:1000), LRRC8A is a glucose sensor regulating ß-cell excitability and systemic glycemia (Kang et al., 2018) |
Antibody | Anti-CD31 (rabbit polyclonal) | Thermo Fisher | Cat#MA3105, RRID:AB_223592 | IF (1:100) |
Antibody | Anti-IgG (normal mouse IgG) | Santa Cruz | Cat#sc-2027, RRID:AB_737197 | WB (1:1000) |
Antibody | Anti-rabbit-HRP | BioRad | Cat#170–6515, RRID:AB_11125142 | WB (1:10,000) |
Antibody | Anti-mouse-HRP | BioRad | Cat#170–5047, RRID:AB_11125753 | WB (1:10,000) |
Transfected construct (human) | siLrrc8a | Invitrogen | Cat#4392420 | Assay ID: s32107 Transfected construct human |
Transfected construct (human) | Non-targeting control | Invitrogen | Cat#4390846 | Transfected construct human |
Transfected construct (human) | siPORTamine | Invitrogen | Cat#AM4503 | |
Transfected construct (human) | Opti-MEM | Invitrogen | Cat#11058–021 | |
Recombinant DNA reagent | Ad5-U6-hLrrc8a-shRNA-mCherry | Vector biolabs | shADV- 653 214592 | |
Recombinant DNA reagent | Ad5-U6-Scr-shRNA-mCherry | Vector biolabs | 3086 | |
Commercial assay or kit | RNA isolation (PureLink RNA mini kit) | Invitrogen | 12183018A | |
Software, algorithm | GraphPad Prism8 | La Jolla California USA, http://www.graphpad.com’ RRID:SCR_002798 | ||
Software, algorithm | Fiji, ImageJ | Schindelin et al., 2012 (PMID:22743772) | RRID:SCR_002285 | |
Other | DAPI stain | Thermofisher | Cat#T3605 | 1:10,000 |
Animals
The institutional animal care and use committee of the University of Iowa and Washington University School of Medicine approved all experimental procedures involving animals. All mice were housed in temperature, humidity, and light controlled environment and allowed water access and food. Both male and female Lrrc8afl/fl (Zhang et al., 2017; Kang et al., 2018) (WT), CDH5-Cre;Lrrc8afl/fl (eLrrc8a KO) mice were generated and used in these studies. CDH5-Cre mice were obtained from Dr. Kaikobad Irani (University of Iowa, IA). In a subset of experiments, 5–8 week old Lrrc8afl/fl and CDH5-Cre;Lrrc8afl/fl mice were switched to HFHS (high-fat high-sucrose rodent diet, Research Diets, Inc, Cat# D12331) for at least 10 months. CDH5-Cre mice were crossed with Rosa26-tdTomato (Jax# 007914) reporter mice to identify CDH5+ cells for primary endothelium patch-clamp studies.
Antibodies
Rabbit polyclonal anti-LRRC8A antibody was generated against the epitope QRTKSRIEQGIVDRSE (Pacific Antibodies) (Kang et al., 2018). All other primary antibodies were purchased from Cells Signaling: anti-ß-actin (#8457), Total Akt (#4685S), Akt1 (#2938), Akt2 (#3063), p-eNOS (#9571), Total eNOS (#32027), p-AS160 (#4288), p-p70 S6 Kinase (#9205S), pS6 Ribosomal (#5364S), GAPDH (#5174), pErk1/2 (#9101), Total Erk1/2 (#9102). Anti-LRRC8A antibody was custom made as described previously (Zhang et al., 2017; Kang et al., 2018). A second p-eNOS antibody was purchased from Invitrogen (Cat#PA5-104858) and used to compare with anti-p-eNOS from Cell Signaling (#9571) for p-eNOS IF staining. Purified mouse anti-Grb2 was purchased from BD (610111) and Santa Cruz (#sc-255). Rabbit IgG was purchased from Santa Cruz (sc-2027). Anti-CD31 was purchased from Thermo Fisher (MA3105).
Electrophysiology
Request a detailed protocolAll recordings were performed in the whole-cell configuration at room temperature (RT), as previously described (Zhang et al., 2017; Kang et al., 2018). Briefly, currents were measured with either an Axopatch 200B amplifier or a MultiClamp 700B amplifier (Molecular Devices) paired to a Digidata 1550 digitizer. Both amplifiers used pClamp 10.4 software. The intracellular solution contained (in mM): 120 L-aspartic acid, 20 CsCl, 1 MgCl2, 5 EGTA, 10 HEPES, 5 MgATP, 120 CsOH, 0.1 GTP, pH 7.2 with CsOH. The extracellular solution for hypotonic stimulation contained (in mM): 90 NaCl, 2 CsCl, 1 MgCl2, 1 CaCl2, 10 HEPES, 5 glucose, 5 mannitol, pH 7.4 with NaOH (210 mOsm/kg). The isotonic extracellular solution contained the same composition as above except for mannitol concentration of 105 (300 mOsm/kg). The osmolarity was checked by a vapor pressure osmometer 5500 (Wescor). Currents were filtered at 10 kHz and sampled at 100 µs interval. The patch pipettes were pulled from borosilicate glass capillary tubes (WPI) by a P-87 micropipette puller (Sutter Instruments). The pipette resistance was ~4–6 MO when the patch pipette was filled with intracellular solution. The holding potential was 0 mV. Voltage steps (500 ms) were elicited from 0 mV holding potential from -100 to +100 mV in 20 mV increments every 0.6 s. Voltage ramps from -100 to +100 mV (at 0.4 mV/ms) were applied every 4 s.
Adenoviral KD
Request a detailed protocolHUVECs were plated at 550,000 cell/well in 12-well plates. Cells were grown for 24 hr in the plates and transduced with either human adenovirus type 5 with shLrrc8a (shLrrc8a: Ad5-mCherry-U6-hLrrc8a-shRNA, 2.2 × 1010 PFU/ml [shADV-214592], Vector Biolabs) or a scrambled non-targeting control (shScr: Ad5-U6-scramble-mCherry, 1 × 1010 PFU/ml) at a multiplicity of infection of 50 for 12 hr, and studies performed 3–4 days after adenoviral transduction. The shLRRC8a targeting sequence is: GCA CAA CAT CAA GTT CGA CGT.
siRNA KD
Request a detailed protocolHUVECs were plated at 360,000 cell/well in six-well plates. Cells were grown for 24 hr (90–95% confluency) and transduced with either a silencer select siRNA with siLRRC8a (Cat#4392420, sense: GCAACUUCUGGUUCAAAUUTT antisense: AAUUUGAACCAGAAGUUGCTG, Invitrogen) or a non-targeting control silencer select siRNA (Cat#4390846, Invitrogen), as described previously (Koh et al., 2008). The siLRRC8a used targets a different sequence from the shRNA described above. Briefly, siRNAs were transduced twice, 24 and 72 hr after HUVEC plating. Each siRNA was combined with Opti-MEM (285.25 µl, Cat#11058-021, Invitrogen) siPORT amine (8.75 µl, Cat#AM4503, Invitrogen) and the silencer select siRNA (6 µl) in a final volume of 300 µl. HUVECs were transduced over a 4 hr period at 37°C, using DMEM +1% FBS. After transduction, the cells were returned to media containing M199, 20% FBS, 0.05 g heparin sodium salt, and 15 mg ECGS. Cell lysates were collected at basal conditions on day 4.
Isolation of mouse lung endothelial cells
Request a detailed protocolIsolation of mouse lung endothelial cells was performed according to the following protocol: Day 1 – Incubate sheep anti-rat IgG Dynabeads (Invitrogen) overnight with PECAM (Sigma, #SAB4502167) and VEGFR2 (R and D Systems, #BAF357) antibodies at 4°C in PBS with gentle agitation. Day 2 – Lungs were removed from the mice, washed in 10% FBS/DMEM, minced into 1–2 mm squares, and digested with Collagenase Type I (2 mg/ml, Gibco) at 37°C for 1 hr with agitation. The cellular digest was filtered through a 70 µm cell strainer, centrifuged at 1500 rpm, and the cells immediately incubated with the antibody coated Dynabeads at RT for 20 min. The bead-bound cells were recovered with a magnet, washed two times with PBS, and plated overnight on collagen type I (100 μg/ml) coated coverslips. The endothelial cells were maintained in a growth media of M199, 20% FBS, 0.05 g heparin sodium salt, 50 mg/ml ECGS, and 1× anti-anti.
Cell culture
Request a detailed protocolHUVECs were purchased from ATCC and were grown in MCDB-131-complete media overnight. HUVECs for basal condition collection were grown in growth media of M199, 20% FBS, 0.05 g heparin sodium salt (Cat#9041-08-1, Alfa Aesar), and 15 mg ECGS (Cat#02-102, Millipore Sigma). Cells were routinely cultured on 1% of gelatin-coated plates at 37°C at 5% CO2. For insulin stimulation (Cat#SLBW8931), cells were serum starved for at least 13 hr in 1% FBS (Atlanta Bio selected, Cat#S11110) or without FBS using endothelial cells growth basal medium (Lonza Cat#cc-3121) instead of MCDB-131-complete media. Insulin stimulation was used for the times indicated at 100 nM.
Immunoblotting
Request a detailed protocolCells were harvested in ice-cold lysis buffer (150 mM NaCl, 20 mM HEPES, 1% NP-40, 5 mM EDTA, pH 7.5) with added proteinase/phosphatase inhibitor (Roche). Cells were kept on ice with gentle agitation for 20 min to allow complete lysis. Lysate scraped into 1.5 ml tubes and cleared of debris by centrifugation at 14,000 × g for 20 min at 4°C. Supernatants were transferred to fresh tube and solubilized protein was measured using a DC protein assay kit (Bio-Rad). For immunoblotting, an appropriate volume of 1× Laemmli (Bio-Rad) sample loading buffer was added to the sample (10 µg of protein), which then heated at 90°C for 5 min before loading onto 4–20% gel (Bio-Rad). Proteins were separated using running buffer (Bio-Rad) for 2 hr at 150 V. Proteins were transferred to PVDF membrane (Bio-Rad) and membrane blocked in 5% (w/v) BSA in TBST or 5% (w/v) milk in TBST at RT for 2 hr. Blots were incubated with primary antibodies at 4°C overnight, followed by secondary antibody (Bio-Rad, Goat-anti-mouse #170–5047, Goat-anti-rabbit #170–6515, all used at 1:10,000) at RT for 1 hr. Membranes were washed three times and incubated in enhanced substrate Clarity (Bio-Rad) and imaged using a ChemiDoc XRS using Image Lab (Bio-Rad) for imaging and analyzing protein band intensities. ß-Actin or GAPDH levels were quantified to correct for protein loading.
Immunoprecipitation
Request a detailed protocolCells were seeded on gelatin-coated 10 cm dishes in complete media for 24 hr. Adenoviruses, Ad5-mCherry-U6-hLrrc8a-shRNA or Ad5-U6-Scr-mCherry were added to cells for 12 hr. After 4 days cells were serum starved for 16 hr with basal media containing 1% serum before stimulation with insulin (10 nM/ml). Cells were harvested in ice-cold lysis buffer (150 mM NaCl, 20 mM HEPES, 1% NP-40, 5 mM EDTA, pH 7.5) with added proteinase/phosphatase inhibitor (Roche) and kept on ice with gentle agitation for 15 min to allow complete lysis. Lysates were incubated with anti-Grb2 antibody (20 µg/ml) or control rabbit IgG (20 µg/ml, Santa Cruz sc-2027) rotating end over end overnight at 4°C. Protein G Sepharose beads (GE) were added to this for a further 4 hr before samples were centrifuged at 10,000 × g for 3 min and washed three times with RIPA buffer and re-suspended in laemmli buffer (Bio-Rad), boiled for 5 min, separated by SDS-PAGE gel followed by the Western blot protocol.
Stretch assay
Request a detailed protocolEqual amounts of cells were plated in each well in six well plated BioFlex coated with Laminin (BF-3001CCase) culture plate and seeded to ~90% confluence. Plates were placed into a FlexCell Jr. Tension System (FX-6000T) and incubated at 37°C with 5% CO2. Prior to stretch stimulation, basal media of 1% FBS was added for 16 hr. Cells on flexible membrane were subjected to static stretch with the following parameter: a stretch of 0.1% and 5% with static strain. Cells were stretched for 5, 30, 60, or 180 min. Cells were then lysed and protein isolated for subsequent Western blots.
Laminar flow studies
Request a detailed protocolHUVECs were grown in 1× M199 media (Gibco, Cat#11043-023) supplemented with 20% FBS (Gibco, Cat#16140-071), 50 mg bovine hypothalamus extract, and 0.01% heparin until full confluence in six-well plates. The cells were transfected twice across 3 days with 100 nM of siRNA for the Lrrc8a gene (siLrrc8a) (Invitrogen, Cat#4392421, assay #:s32107) and a negative siRNA control (siControl) (Invitrogen, Cat#43900846) using siPORTAmine transfection agent (Invitrogen, Cat#AM4503) following the manufacturer recommendations (Koh et al., 2008). Twenty-four hours after the final siRNA treatment, cells were transferred to µ-Slide 0.4 I Luer slide (iBidi, Cat#80176) and incubated overnight at 37°C, 5% CO2, and 95% humidity. The slides were then cultured for 24 hr in a microscope stage incubator (Invitrogen, EVOS7000) under conditions of constant laminar flow at 15 dynes/cm2/s (Flocel Inc, Cat#QPL1010) and images acquired every 20 min. The peristaltic pump was converted to be laminar through the utilization of dampers on the outflow tracts of the pump. Efficacy in the generation of laminar flow was confirmed through the imaging of Q-Dots in the circulating media prior to the experiment (Invitrogen, Cat#Q21721MP). At the termination of the experiment, the cells were rinsed with 1× PBS and fixed with 4% paraformaldehyde (PFA) for downstream immunostaining applications.
Immunostaining for laminar flow studies
Request a detailed protocolTo detect the levels of p-eNOS, the cell monolayer was stained with the anti-rabbit polyclonal antibody p-eNOS (Invitrogen, Cat#PA5104858 or Cell Signaling, Cat#9571), at a final dilution of 1:200 and a secondary anti-rabbit GFP AlexaFluor 488 (Invitrogen, Cat#R37116) at 1:2000 dilution. The slides were immunostained following the basic protocol: (1) 30 min RT incubation in Tris-Glycine; (2) 1 hr RT incubation ± permeabilization with 0.01% TritonX-100; (3) 2 hr RT incubation in blocking solution (5% Sheep Serum, 1% Roche Blocking Buffer in PBST); (4) 1 hr at RT – overnight 4°C incubation with 1:200 primary antibody unless otherwise noted; (5) wash with PBST; (6) 2–3 hr RT incubation with 1:2000 secondary antibody in 5% Sheep Serum, 1% Roche Blocking Buffer in PBST; (7) wash with PBST and imaging analysis. The samples were observed under light microscopy using a Nikon Ti2. Quantification of immunostaining intensity was performed using ImageJ/Fiji (Schindelin et al., 2012).
Immunofluorescence imaging
Request a detailed protocolCells were plated on gelatin-coated glass coverslips. Cells on coverslip were washed in PBS and fixed with 2% (w/v) PFA for 20 min at RT. PFA was washed three times with PBS and permeabilized in PBS containing 0.2% Triton X-100 for 5 min at RT. Cells on coverslips were washed in PBS and blocked for 30 min at RT with TBS containing 0.1% Tween-20% and 5% BSA. Cells on coverslip were incubated overnight at 4°C with primary antibody (1:250) in TBS containing 0.1% Tween-20% and 1% BSA. Cells were then washed in PBS five times and incubated for 2 hr at RT with 5% BSA in TBST. Cells were washed three times in TBST and then incubated with secondary antibodies at 1:1000 dilution (Invitrogen, Anti-Rabbit 488, A11070; Anti-mouse 568, A11019; Anti-mouse 488, A11017) for 1 hr at RT. Coverslips were then incubated for 10 min with Topro 3 (T3605, Thermofisher) or mounted with mounting media containing DAPI (Invitrogen) to visualize nuclei. Images were taken using Axiocam 503 Mono Camera controlled by Zeiss Blue using a Plan-Apochromate 40× oil immersion objective.
Immunohistochemistry of thoracic aorta sections
Request a detailed protocolMouse thoracic aortas were dissected and immediately fixed in 10% formalin. The formalin fixed aortas were embedded in paraffin and 5 µm sections were cut by automated microtome and mounted on positively charged slides. Slides were deparaffinized with Histoclear and hydrated with 100% ethanol, 75% ethanol, 50% ethanol, and then distilled water. To achieve the antigen retrieval, slides were steamed in Citrate buffer at pH 6 (DAKO Target retrieval buffer S1699, DAKO) for 30 min and subsequently cooled for 30 min. Furthermore, to inhibit the endogenous peroxides, slides were quenched with 3% H2O2 for 10 min. Slides were washed with distilled water, then 1× PBS and blocked with 1% BSA in 1× PBS for 1 hr. After washing with 1× PBS, slides were incubated with p-eNOS antibody (1:500 dilution) overnight in the humidified chamber at 4°C. Slides were washed two times with 1× PBS and incubated with secondary antibody (AF488, Invitrogen, 1:1000 dilution) for 1 hr. Subsequently slides were washed with 1× PBS three times and mounted with DAPI added mounting media with coverslip. The slides were imaged by Zeiss LSM700 confocal microscope (10× objective).
Ex vivo sprouting angiogenesis
Request a detailed protocolFollowing Avertin injection and cervical dislocation, aortas were dissected and connective tissue removed, and then washed with PBS with 50 µg/ml penicillin and streptomycin. Using iris scissors, the aorta was cut into aortic rings of 1– 2 mm cross-sectional slices; 50 µl of Matrigel was used to coat the center of coverslips in 24-well plates for 2 hr at 37°C in the incubator to solidify the Matrigel. Aorta rings were then seeded and transplanted on Matrigel (BD Biosciences, Cat#356231) on coverslips. After seeding the aortic rings, plates were incubated at 37°C without medium for 10 min to allow the ring to attach to the Matrigel. Complete medium was added to each well and incubated at 37°C with 5% CO2 for 48–72 hr. Phase contrast photos of individual explants were taken using a 10×/0.75 NA objective Olympus IX73 microscope (Olympus, Japan) fitted with camera (Orca flash 4.0+, Hamamatsu, Japan). Cells were incubated with LRRC8A (1:250) and CD31 (1:250) primary antibodies in 0.1% Tween-20% and 1% BSA overnight, and then incubated with secondary antibodies (Invitrogen, Anti-Rabbit 488, A11070; Anti-Mouse 568, A11019). Cells were then incubated for 10 min with Topro 3 (T3605, Thermofisher) at RT.
Retinal imaging
Request a detailed protocolKetamine (Akorn Animal Health, 100 mg/ml) was prepared and mixed with xylazine, then stored at RT. Animals were anesthetized with 87.5/12 mg/kg body weight via i.p. injection. Eyes were topically anesthetized with proparacaine and dilated with tropicamide. Fluorescein (100 mg/ml, Akorn Inc) diluted with sterile saline was administered by i.p. injection (50 µl), and mice were positioned on Micron imaging platform. Images of the eyes were taken from the start of fluorescein infusion with the Micron camera with a 450–650 nm excitation filter and 469–488 nm barrier filter at 30 frame/s using Micron software for 30 s. Data were converted into tiff image for further analysis.
Ang II infusion
Request a detailed protocolInfusion studies were carried out using Azlet osmotic minipumps (Model 1004). Ang II (BACHEM) dissolved in saline was filled in the minipumps and were prepared to maintain infusion rate of 600 ng/kg/min for 4 weeks. The mice were anesthetized under 2% isofluorane and the minipumps were implanted subcutaneously on the dorsal aspect of the mice.
Blood pressure recordings
Request a detailed protocolSystolic tail-cuff blood pressure (BP) measurements were carried out using computerized tail-cuff system BP-2000 (Visitech Systems) at the same time of day. Mice were first acclimated to the device by performing 3 days of measurements (20 sequential measurements/day) and then mean blood pressure readings were obtained by averaging 3–5 days of measurements (not inclusive of the 3 acclimation days).
RNA sequencing
Request a detailed protocolRNA quality was assessed by Agilent BioAnalyzer 2100 by the University of Iowa Institute of Human Genetics, Genomics Division. RNA integrity numbers greater than eight were accepted for RNAseq library preparation. RNA libraries of 150 bp PolyA-enriched RNA were generated, and sequencing was performed on a HiSeq 4000 genome sequencing platform (Illumina). Sequencing results were uploaded and analyzed with BaseSpace (Illumina). Sequences were trimmed to 125 bp using FASTQ Toolkit (Version 2.2.0) and aligned to Homo sapiens HG19 (refseq) using RNA-Seq Alignment (Version 1.1.0). Transcripts were assembled and differential gene expression was determined using Cufflinks Assembly and DE (Version 2.1.0). Ingenuity Pathway Analysis (QIAGEN) was used to analyze significantly regulated genes which were filtered using cutoffs of >1.5 fragments per kilobase per million reads, >1.5-fold changes in gene expression, and a false discovery rate of <0.05. Heatmaps were generated to visualize significantly regulated genes. Data have been deposited in GEO (accession# TBD).
Metabolic phenotyping
Request a detailed protocolMice were fasted for 6 hr prior to glucose tolerance tests (GTTs). Baseline glucose levels at 0 min timepoint (fasting glucose) were measured from blood samples collected from tail bleeds using a glucometer (Bayer Healthcare LLC). D-Glucose was injected i.p. (0.75 g/kg body weight) and glucose levels were measured at 7, 15, 30, 60, 90, and 120 min timepoints after injection. For insulin tolerance tests (ITTs), the mice were fasted for 4 hr. Similar to GTTs, the baseline blood glucose levels were measured at 0 min timepoint and 15, 30, 60, 90, and 120 min timepoints post-injection (i.p.) of insulin (HumulinR, 1.25 U/kg body weight). Mouse body composition was performed as previously described (Zhang et al., 2017).
Statistics
Data are represented as mean ± s.e.m. Two-tail unpaired Student’s t-tests were used for comparison between two groups. For three or more groups, data were analyzed by one-way analysis of variance (ANOVA) and Tukey’s post hoc test. For time-series data in Figure 5 between two groups, data were analyzed using a two-way ANOVA followed by Bonferroni post hoc test. For GTTs and ITTs, two-way ANOVA was used. A p-value<0.05 was considered statistically significant. *, **, and *** represent a p-value less than 0.05, 0.01, and 0.001, respectively.
Data availability
RNA sequencing data has been deposited in GEO under accession number GSE166989. All other data available as source data files.
-
NCBI Gene Expression OmnibusID GSE166989. The SWELL1-LRRC8 complex regulates endothelial AKT-eNOS- mTOR signaling and vascular function.
References
-
Body fat distribution predicts the degree of endothelial dysfunction in uncomplicated obesityInternational Journal of Obesity 23:936–942.https://doi.org/10.1038/sj.ijo.0801022
-
A flow-activated chloride-selective membrane current in vascular endothelial cellsCirculation Research 85:820–828.https://doi.org/10.1161/01.RES.85.9.820
-
Responsiveness of vascular endothelium to shear stress: potential role of ion channels and cellular cytoskeleton (review)International Journal of Molecular Medicine 4:323–332.https://doi.org/10.3892/ijmm.4.4.323
-
Reduced severity of oxygen-induced retinopathy in eNOS-deficient miceInvestigative Ophthalmology & Visual Science 42:222–228.
-
Stretch of beta 1 integrin activates an outwardly rectifying chloride current via FAK and src in rabbit ventricular myocytesJournal of General Physiology 122:689–702.https://doi.org/10.1085/jgp.200308899
-
Vascular endothelium - Gatekeeper of vessel healthAtherosclerosis 248:97–109.https://doi.org/10.1016/j.atherosclerosis.2016.03.007
-
DEPTOR Deficiency-Mediated mTORc1 hyperactivation in vascular endothelial cells promotes angiogenesisCellular Physiology and Biochemistry 46:520–531.https://doi.org/10.1159/000488619
-
TRPV4-dependent dilation of peripheral resistance arteries influences arterial pressureAmerican Journal of Physiology-Heart and Circulatory Physiology 297:H1096–H1102.https://doi.org/10.1152/ajpheart.00241.2009
-
Caveolae-Mediated activation of mechanosensitive chloride channels in pulmonary veins triggers atrial arrhythmogenesisJournal of the American Heart Association 8:e012748.https://doi.org/10.1161/JAHA.119.012748
-
VEGF induces nuclear translocation of Flk-1/KDR, endothelial nitric oxide synthase, and caveolin-1 in vascular endothelial cellsBiochemical and Biophysical Research Communications 256:192–197.https://doi.org/10.1006/bbrc.1998.9790
-
Nitric oxide signaling via nuclearized endothelial nitric-oxide synthase modulates expression of the immediate early genes iNOS and mPGES-1Journal of Biological Chemistry 281:16058–16067.https://doi.org/10.1074/jbc.M602219200
-
Long-term cyclic strain downregulates endothelial Nox4Antioxidants & Redox Signaling 11:2385–2397.https://doi.org/10.1089/ars.2009.2561
-
Relationships between caveolae and eNOS: everything in proximity and the proximity of everythingAmerican Journal of Physiology-Renal Physiology 283:F1–F10.https://doi.org/10.1152/ajprenal.00377.2001
-
Direct interaction of endothelial nitric-oxide synthase and caveolin-1 inhibits synthase activityJournal of Biological Chemistry 272:18522–18525.https://doi.org/10.1074/jbc.272.30.18522
-
Insulin resistance and endothelial cell dysfunction: studies in mammalian modelsExperimental Physiology 93:158–163.https://doi.org/10.1113/expphysiol.2007.039172
-
Dietary cholesterol plays a role in CD36-mediated atherogenesis in LDLR-knockout miceArteriosclerosis, Thrombosis, and Vascular Biology 29:1481–1487.https://doi.org/10.1161/ATVBAHA.109.191940
-
Knockout of insulin and IGF-1 receptors on vascular endothelial cells protects against retinal neovascularizationJournal of Clinical Investigation 111:1835–1842.https://doi.org/10.1172/JCI200317455
-
Leucine-rich repeat containing 8A (LRRC8A) is essential for T lymphocyte development and functionJournal of Experimental Medicine 211:929–942.https://doi.org/10.1084/jem.20131379
-
LRRC8/VRAC anion channels are required for late stages of spermatid development in miceJournal of Biological Chemistry 293:11796–11808.https://doi.org/10.1074/jbc.RA118.003853
-
TRPV4-mediated endothelial Ca2+ influx and vasodilation in response to shear stressAmerican Journal of Physiology. Heart and Circulatory Physiology 298:466–476.https://doi.org/10.1152/ajpheart.00854.2009
-
Local control of TRPV4 channels by AKAP150-targeted PKC in arterial smooth muscleJournal of General Physiology 143:559–575.https://doi.org/10.1085/jgp.201311050
-
Phosphoinositide 3-kinase signalling in the vascular systemCardiovascular Research 82:261–271.https://doi.org/10.1093/cvr/cvn325
-
Role of insulin resistance in endothelial dysfunctionReviews in Endocrine and Metabolic Disorders 14:5–12.https://doi.org/10.1007/s11154-012-9229-1
-
Vascular dysfunction and reduced circulating endothelial progenitor cells in young healthy UK south asian menArteriosclerosis, Thrombosis, and Vascular Biology 27:936–942.https://doi.org/10.1161/01.ATV.0000258788.11372.d0
-
Mechanical stress-induced Ca2+ entry and cl- current in cultured human aortic endothelial cellsThe American Journal of Physiology 276:238–249.https://doi.org/10.1152/ajpcell.1999.276.1.C238
-
Stressing caveolae new role in cell mechanicsTrends in Cell Biology 22:381–389.https://doi.org/10.1016/j.tcb.2012.04.007
-
Ion channels and their functional role in vascular endotheliumPhysiological Reviews 81:1415–1459.https://doi.org/10.1152/physrev.2001.81.4.1415
-
CD36, a scavenger receptor implicated in atherosclerosisExperimental & Molecular Medicine 46:e99.https://doi.org/10.1038/emm.2014.38
-
The role of nitric oxide and cGMP in platelet adhesion to vascular endotheliumBiochemical and Biophysical Research Communications 148:1482–1489.https://doi.org/10.1016/S0006-291X(87)80299-1
-
Dual effect of fluid shear stress on volume-regulated anion current in bovine aortic endothelial cellsAmerican Journal of Physiology-Cell Physiology 282:C708–C718.https://doi.org/10.1152/ajpcell.00247.2001
-
A congenital mutation of the novel gene LRRC8 causes agammaglobulinemia in humansJournal of Clinical Investigation 112:1707–1713.https://doi.org/10.1172/JCI18937
-
Reduced basal nitric oxide bioavailability and platelet activation in young spontaneously hypertensive ratsBiochemical Pharmacology 67:2273–2279.https://doi.org/10.1016/j.bcp.2004.02.034
-
Fiji: an open-source platform for biological-image analysisNature Methods 9:676–682.https://doi.org/10.1038/nmeth.2019
-
Obesity/insulin resistance is associated with endothelial dysfunction. Implications for the syndrome of insulin resistanceJournal of Clinical Investigation 97:2601–2610.https://doi.org/10.1172/JCI118709
-
Caveolin-1 modulates the activity of the volume-regulated chloride channelThe Journal of Physiology 520 Pt 1:113–119.https://doi.org/10.1111/j.1469-7793.1999.t01-1-00113.x
-
Inhibition of volume-regulated anion channels by dominant-negative caveolin-1Biochemical and Biophysical Research Communications 284:461–465.https://doi.org/10.1006/bbrc.2001.4995
-
Caveolin-1 detergent solubility and association with endothelial nitric oxide synthase is modulated by tyrosine phosphorylationBiochemical and Biophysical Research Communications 236:155–161.https://doi.org/10.1006/bbrc.1997.6921
-
eNOS S-nitrosylation mediated OxLDL-induced endothelial dysfunction via increasing the interaction of eNOS with ß-cateninBiochimica Et Biophysica Acta (BBA) - Molecular Basis of Disease 1865:1793–1801.https://doi.org/10.1016/j.bbadis.2018.02.009
-
Effect of fat distribution on endothelial-dependent and endothelial-independent vasodilatation in healthy humansDiabetes, Obesity and Metabolism 8:296–301.https://doi.org/10.1111/j.1463-1326.2005.00505.x
-
Regulation of endothelial nitric oxide synthase activity and gene expressionAnnals of the New York Academy of Sciences 962:122–130.https://doi.org/10.1111/j.1749-6632.2002.tb04062.x
-
Insulin-stimulated production of nitric oxide is inhibited by wortmannin. Direct measurement in vascular endothelial cellsJournal of Clinical Investigation 98:894–898.https://doi.org/10.1172/JCI118871
-
SWELL1 is a regulator of adipocyte size, insulin signalling and glucose homeostasisNature Cell Biology 19:504–517.https://doi.org/10.1038/ncb3514
-
Endothelial Grb2-associated binder 1 is crucial for postnatal angiogenesisArteriosclerosis, Thrombosis, and Vascular Biology 31:1016–1023.https://doi.org/10.1161/ATVBAHA.111.224493
Article and author information
Author details
Funding
National Institute of Diabetes and Digestive and Kidney Diseases (R01DK106009)
- Rajan Sah
National Heart, Lung, and Blood Institute (5R00HL125683)
- Amber N Stratman
National Heart, Lung, and Blood Institute (R01 HL125436)
- Chad E Grueter
National Heart, Lung, and Blood Institute (R35 GM137976)
- Amber N Stratman
VA Merit Award (I01BX005072)
- Rajan Sah
Cancer Research Foundation (Young Investigator Award)
- Amber N Stratman
Roy J. Carver Charitable Trust
- Rajan Sah
UIHC Center (I01B 005072)
- Rajan Sah
The funders had no role in study design, data collection and interpretation, or the decision to submit the work for publication.
Acknowledgements
RNA-Seq data presented herein were obtained at the Genomics Division of the Iowa Institute of Human Genetics. This work was supported by grants from the NIH/NHLBI R01 HL125436 (CEG), NIH/NHLBI 5R00HL125683, NIH/NIGMS R35 GM137976, Cancer Research Foundation Young Investigator Award (ANS), NIH NIDDK 1R01DK106009, the Roy J Carver Trust (RS), UIHC Center for Hypertension Research Pilot and Feasibility Grant, VA Merit Award I01BX005072 (RS), andKing Abdullah International Medical Research Center (KAIMRC) grant RA17-014-A (AA). We thank Dr. Rithwick Rajagopal for insightful reading of the manuscript.
Ethics
Animal experimentation: This study was performed in accordance with the recommendations in the Guide for the Care and Use of Laboratory Animals of the National Institutes of Health. All of the animals were handled according to the approved Institutional Animal Care and Use Committee (IACUC) protocols of Washington University in St. Louis (20180217) and the University of Iowa (1308148).
Copyright
© 2021, Alghanem et al.
This article is distributed under the terms of the Creative Commons Attribution License, which permits unrestricted use and redistribution provided that the original author and source are credited.
Metrics
-
- 2,737
- views
-
- 394
- downloads
-
- 51
- citations
Views, downloads and citations are aggregated across all versions of this paper published by eLife.
Citations by DOI
-
- 51
- citations for umbrella DOI https://doi.org/10.7554/eLife.61313