The Hippo pathway controls myofibril assembly and muscle fiber growth by regulating sarcomeric gene expression
Abstract
Skeletal muscles are composed of gigantic cells called muscle fibers, packed with force-producing myofibrils. During development, the size of individual muscle fibers must dramatically enlarge to match with skeletal growth. How muscle growth is coordinated with growth of the contractile apparatus is not understood. Here, we use the large Drosophila flight muscles to mechanistically decipher how muscle fiber growth is controlled. We find that regulated activity of core members of the Hippo pathway is required to support flight muscle growth. Interestingly, we identify Dlg5 and Slmap as regulators of the STRIPAK phosphatase, which negatively regulates Hippo to enable post-mitotic muscle growth. Mechanistically, we show that the Hippo pathway controls timing and levels of sarcomeric gene expression during development and thus regulates the key components that physically mediate muscle growth. Since Dlg5, STRIPAK and the Hippo pathway are conserved a similar mechanism may contribute to muscle or cardiomyocyte growth in humans.
Introduction
Mammalian skeletal muscles are built from gigantic cells called muscle fibers, up to several centimetres long, that mechanically link distant skeletal elements. Muscle forces are produced by highly regular molecular arrays of actin, myosin and titin filaments called sarcomeres. Each sarcomere has a length of about 3 µm in relaxed human skeletal muscles (Ehler and Gautel, 2008; Llewellyn et al., 2008; Regev et al., 2011). Thus, hundreds of sarcomeres need to assemble into long chains called myofibrils in order to generate force across the entire muscle fiber (Lemke and Schnorrer, 2017). Large muscle fibers contain many parallel myofibrils, which are laterally aligned to a cross-striated pattern to effectively power animal locomotion (Gautel, 2008; Schiaffino et al., 2013). How muscle fibers grow to these enormous sizes and how their growth is coordinated with the assembly and maturation of the individual myofibrils within the muscle is a challenging biological problem that is not well understood.
Muscle fibers are built during animal development. Initially, many small myoblasts fuse to myotubes, whose long-ends then mechanically connect to tendon cells (Kim et al., 2015; Schnorrer and Dickson, 2004). This enables the build-up of mechanical tension within myotubes, which consecutively triggers myofibril assembly and the transition of myotubes to early myofibers (Weitkunat et al., 2017; Weitkunat et al., 2014). Following myofibril assembly, the immature myofibrils mature and build functional sarcomeres. To do so, each myofibril grows in length and diameter and thereby supports the extensive muscle fiber growth during embryonic and postembryonic development (González-Morales et al., 2019; Orfanos et al., 2015; Reedy and Beall, 1993; Sanger et al., 2017; Sparrow and Schöck, 2009). For the correct developmental sequence of myofibril morphogenesis, the protein concentrations of the various sarcomeric components need to be precisely regulated (Orfanos and Sparrow, 2013; Schönbauer et al., 2011). This is particularly prominent in mammalian muscle fibers, in which sarcomeric proteins transcriptionally switch isoforms from embryonic to neonatal and finally to adult isoforms (Schiaffino, 2018; Schiaffino et al., 2015). In Drosophila indirect flight muscles, transcription of sarcomeric and mitochondrial protein coding genes starts just before myofibril assembly and is then strongly boosted during myofibril maturation, when myofibrils grow in length and width (González-Morales et al., 2019; Shwartz et al., 2016; Spletter et al., 2018). Concomitantly with the growth of the myofibrils, the T-tubule network forms (Peterson and Krasnow, 2015; Sauerwald et al., 2019) and also the mitochondria grow in size (Avellaneda et al., 2020; Spletter et al., 2018). How this precise transcriptional control is achieved and coordinated with muscle fiber growth is unclear.
One central pathway controlling organ size during development and tumorigenesis is the Hippo pathway, which regulates the activity of the growth promoting transcriptional co-activator Yorkie (Yki, YAP and TAZ in mammals) (Pan, 2010; Zanconato et al., 2019). The core of the pathway is composed of a kinase cascade with Hippo (Hpo; Mst1 and Mst2 in mammals) phosphorylating the downstream kinase Warts (Wts; Lats1 and Lats2 in mammals) (Udan et al., 2003; Wu et al., 2003). Phosphorylated Wts is active and in turn phosphorylates Yki (Huang et al., 2005), leading to the cytoplasmic retention of phospho-Yki by 14-3-3 proteins (Dong et al., 2007; Oh and Irvine, 2008; Ren et al., 2010). When the pathway is not active, unphosphorylated Yki enters into the nucleus, binds to the Tead protein Scalloped (Sd), and turns on transcriptional targets (Goulev et al., 2008; Wu et al., 2008; Zhang et al., 2008). The majority of these targets promote organ growth by suppressing apoptosis and stimulating cell growth and cell proliferation (Harvey and Tapon, 2007).
A key control step of the Hippo pathway is the localisation and kinase activity of Hippo. In epithelial cells, the scaffold protein Salvador promotes Hippo kinase activity by localising Hippo to the plasma membrane (Yin et al., 2013) and by inhibiting a large protein complex called the STRIPAK (Striatin-interacting phosphatase and kinase) complex (Bae et al., 2017). The STRIPAK complex contains PP2A as active phosphatase, which dephosphorylates a key Hippo auto-phosphorylation site and thus inhibits Hippo activity (Ribeiro et al., 2010; Zheng et al., 2017). dRassf can promote the recruitment of STRIPAK to Hippo and thus inactivate Hippo (Polesello et al., 2006). Furthermore, the Hippo pathway can also be regulated downstream by membrane localisation of the kinase Warts by Merlin binding, which promotes Warts phosphorylation by Hippo and thus activation of the pathway (Yin et al., 2013). Finally, mechanical stretch of the epithelial cell cortex was shown to directly inhibit the Hippo pathway, likely mediated by the spectrin network at the cortex, promoting nuclear localisation of Yorkie (Fletcher et al., 2018; Fletcher et al., 2015). Despite this detailed knowledge about Hippo regulation in proliferating epithelial cells, little is known about how the Hippo pathway is regulated during post-mitotic muscle development and how it impacts muscle growth.
Here, we employ a systematic in vivo muscle-specific RNAi screen and identify various components of the Hippo pathway as essential post-mitotic regulators of flight muscle morphogenesis. We find that loss of Dlg5 or of the STRIPAK complex member Slmap, which interacts with Dlg5, as well as loss of the transcriptional regulator Yorkie results in too small muscles. These small muscles express lower levels of sarcomeric proteins and as a consequence contain fewer and defective myofibrils. Conversely, over-activation of Yorkie, either by removing the negative regulators Hippo or Warts or by enabling constitutive nuclear entry of Yorkie results in premature and excessive expression of sarcomeric proteins and consequently in chaotic myofibril assembly. Therefore, our findings suggest that the Hippo pathway contributes to the precise timing of sarcomeric gene expression and thus can generate a feedback mechanism for muscles to precisely coordinate sarcomeric protein levels during myofibril assembly and maturation. This provides an attractive mechanism for how regulated transcription can coordinate muscle growth with myofibril morphogenesis.
Results
Growth of Drosophila flight muscles
We chose the Drosophila indirect flight muscles to investigate post-mitotic muscle fiber growth. These muscles consist of two groups, the dorsal-longitudinal flight muscles (DLMs) and the dorso-ventral flight muscles (DVMs). Both groups form in the second thoracic segment during pupal development and despite differences during myoblast fusion and myotube attachment determining their location in the thorax, their development after 24 hr after puparium formation (APF) is very similar (Dutta et al., 2004; Fernandes et al., 1991; Schönbauer et al., 2011). Thus, we focused our studies on the DLMs and for simplicity call them flight muscles in the remainder of the manuscript.
In order to quantify muscle fiber growth we measured fiber length and cross-sectional area of wild-type DLM flight muscles. At 24 hr APF (at 27°C) myoblast fusion is largely finished (Weitkunat et al., 2014), and the fibers have a length of about 270 µm and a cross-sectional area of about 1000 µm2 (Figure 1A,C, Supplementary file 1). Then, flight muscles build-up mechanical tension, compact to about 220 µm in length, while their diameter grows to about 2000 µm2, and assemble the immature myofibrils at 32 hr APF (Lemke et al., 2019; Weitkunat et al., 2014; Figure 1A,C). After 32 hr, flight muscles grow about 2.5 times in length while keeping a similar diameter until 48 hr APF. After 48 hr APF, they grow further to about 800 µm in length while increasing in diameter to almost 4000 µm2 until 90 hr APF, which is shortly before eclosion at 27°C (Figure 1A, Supplementary file 1; Spletter et al., 2018). Thus, in total, the volume of the individual muscle fibers increases more than 10-fold in less than 3 days (Figure 1A, Supplementary file 1). Thus, indirect flight muscles are a good model to study rapid post-mitotic muscle fiber growth.
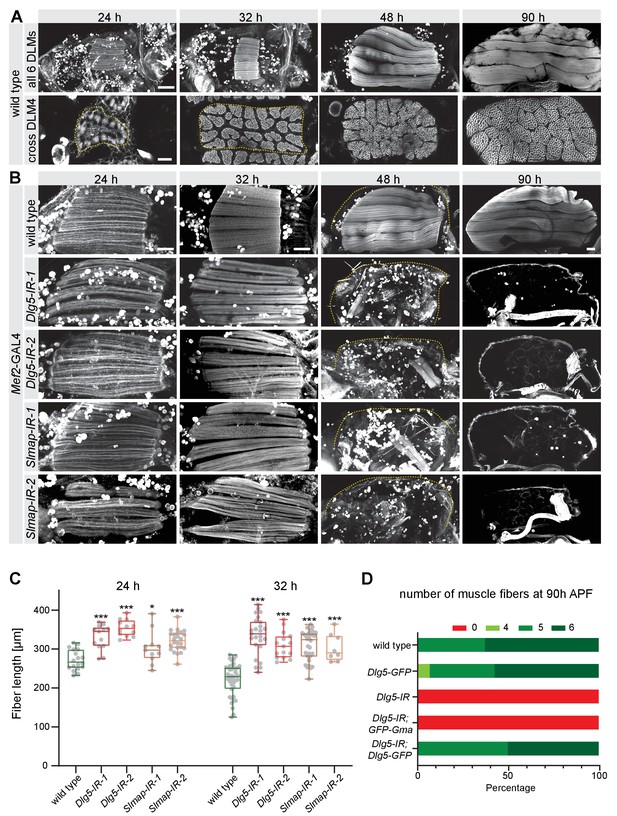
Dlg5 and Slmap are essential for flight muscle morphogenesis.
(A) Time-course of wild-type dorsal longitudinal indirect flight muscle (DLM) development. Longitudinal sections (upper panel) of all DLMs and cryo cross-sections (lower panel) of DLM4 were stained for actin. Note the muscle fiber growth in length and width. Scale bars represent 100 µm for longitudinal and 10 µm for cross-sections. (B) Longitudinal views of developing flight muscles at 24 hr, 32 hr, 48 hr, and 90 hr after puparium formation (APF) of wild-type, Dlg5, or Slmap knockdown genotypes (independent RNAi lines IR-1 and 2) stained for actin. Note that Dlg5-IR and Slmap-IR muscles are too long at 24 hr and 32 hr APF, and are lost after 32 hr APF. The dotted lines highlight the cuticle. Scale bars represent 50 µm. (C) Box plot showing flight muscle fiber length at 24 hr and 32 hr APF of the indicated genotypes. Each dot represents the average flight muscle length from one pupa (see Materials and methods). Box extends from 25% to 75%, line marks median, whiskers extend from max to min, all data points superimposed. Student’s t-test, *** p-value<0.001, *p value < 0.05. All following box plots are plotted the same way. N ≥ 8 pupae for each genotype. (D) Number of flight muscle fibers in half thoraces at 90 hr APF of the indicated genotypes. Note that UAS-Dlg5-GFP but not UAS-GFP-Gma rescues the fiber atrophy phenotype of Dlg5 knockdown (Dlg1-IR-1, in all the following figures IR refers to IR-1).
Dlg5 and Slmap are essential for flight muscle morphogenesis
To identify regulators of muscle growth, we have investigated genes identified in a genome-wide muscle-specific RNAi study that had resulted in flightless or late developmental lethality when knocked-down using muscle-specific Mef2-GAL4 (Schnorrer et al., 2010). Our analysis identified two genes, Dlg5 (Discs large 5, CG6509) and Slmap (Sarcolemma associated protein, CG17494), which are conserved from Drosophila to human and when knocked-down using several independent RNAi constructs result in viable but completely flightless flies (Figure 1—figure supplement 1, Supplementary file 1). Inspection of the thoraces of these animals revealed complete flight muscle atrophy in pupae at 90 hr APF (Figure 1B). Expression of a UAS-Dlg5-GFP but not a UAS-GFP-Gma (globular moesin actin binding domain fused to GFP) control construct, was able to rescue the number of muscle fibers of Dlg5 knock-down (Dlg5-IR-1) flies to wild type providing further strong evidence for the specificity of the knock-down phenotype (Figure 1D). We conclude that Dlg5 and Slmap are two conserved genes essential for flight muscle morphogenesis during pupal stages.
To identify the developmental time point when Dlg5 and Slmap are required, we analysed pupal stages and found that at 24 hr APF all flight muscles are present after Dlg5 or Slmap knockdown. However, the fibers are more than 20% longer than wild type and fail to compact at 32 hr APF when myofibrils normally assemble (Figure 1B,C, Supplementary file 1). Interestingly, after 32 hr APF, when wild-type myofibers strongly grow in length, Dlg5 and Slmap knock-down fibers undergo complete flight muscle atrophy until 48 hr APF (Figure 1B). Taken together, these data demonstrate that Dlg5 and Slmap play an essential role during stages of myofibril assembly and muscle fiber growth.
Dlg5 and Slmap partially co-localise with Dlg1 during T-tubule morphogenesis
We aimed to determine the subcellular localisation of Dlg5 and Slmap proteins in developing flight muscles and thus raised polyclonal antibodies against both proteins. Both antibodies are specific as the Dlg5 or Slmap intensities in the flight muscles, but not in motor neurons or tendons, are strongly reduced in the respective muscle-specific Dlg5 or Slmap knock-down at 32 hr APF (Figure 2—figure supplement 1A,B). This also demonstrates the efficiency of the muscle-specific Dlg5 or Slmap knock-down at protein level.
Detailed immunostainings revealed that both Dlg5 and Slmap proteins largely localise to membranous structures called T-tubules at 90 hr APF (Figure 2A,B), which are marked by the Dlg5-related protein Dlg1 and the membrane-binding protein Amphiphysin (Figure 2C; Razzaq et al., 2001). The mature T-tubules are in intimate contact with the mature myofibrils at their sarcomeric A-bands (Figure 2D; Razzaq et al., 2001). Interestingly, at 32 hr and 48 hr APF when first myofibrils and then T-tubules assemble, Dlg5 and Slmap proteins partially co-localise with Dlg1 (Figure 2A,B), however, both proteins show a more vesicular pattern compared to Dlg1 or Amphiphysin at these stages (Figure 2C). Together, these data show that Dlg5 and Slmap are expressed during muscle development and localise at or close to membranous structures forming the T-tubules that will closely interact with the maturing myofibrils.
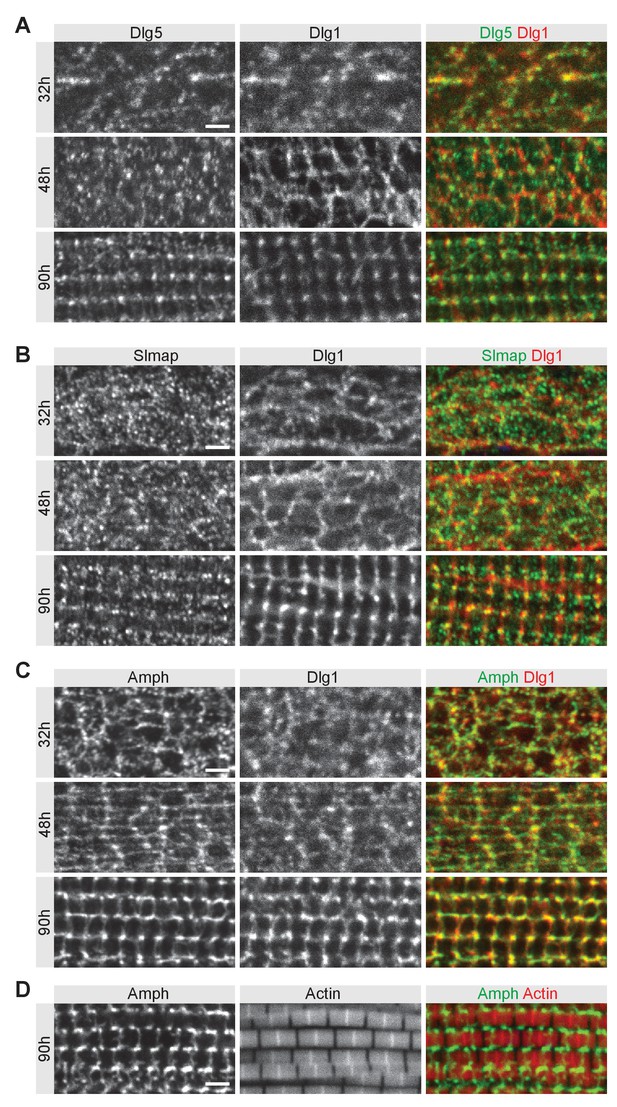
Dlg5 and Slmap partially co-localise with Dlg1 during T-tubule morphogenesis.
(A–C) Wild-type developing flight muscles at 32 hr, 48 hr, and 90 hr after puparium formation (APF) co-stained for T-tubule marker Dlg1 with Dlg5 (A) or Slmap (B) or with another T-tubule marker Amphiphysin (C). (D) Wild-type flight muscles at 90 hr APF co-stained for Amphiphysin and actin with phalloidin. Scale bars represent 2 µm.
Dlg5 interacts with Slmap, a STRIPAK complex member, in Drosophila muscle
To define a molecular mechanism for how Dlg5 regulates muscle morphogenesis, we performed GFP immunoprecipitation followed by mass-spectrometry, using the functional UAS-Dlg5-GFP, which was expressed in pupal muscles with Mef2-GAL4. Interestingly, we not only identified Slmap as a binding partner of Dlg5 in muscle, but also Fgop2, GckIII, Striatin (Cka), and the catalytic subunit of PP2A phosphatase (Mts) (Figure 3A,B, Supplementary file 1). All these proteins are members of the STRIPAK complex and have been described to interact closely in mammals (Hwang and Pallas, 2014). This suggests that the composition of the STRIPAK complex in fly muscles is similar to mammals (Figure 3C), and Dlg5 is either a core member or closely interacts with this complex in muscle.
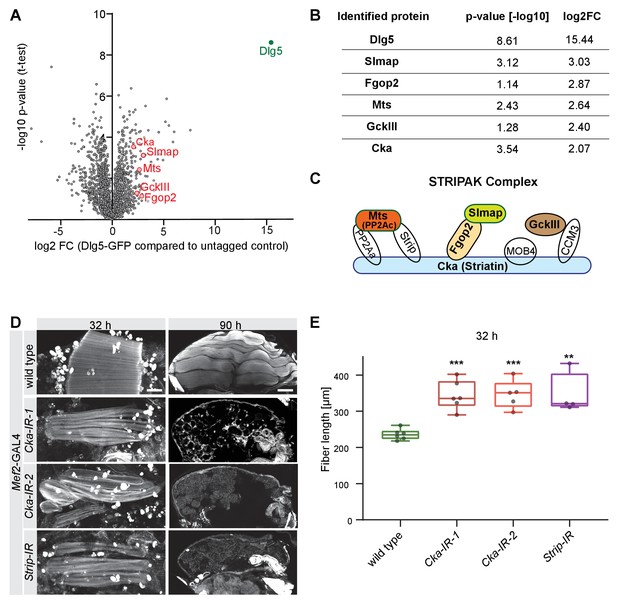
Dlg5 binds to the STRIPAK complex.
(A) Volcano plot showing proteins enriched in GFP pull-down from Mef2-GAL4, UAS-Dlg5-GFP 24 hr - 48 hr after puparium formation (APF) pupae. Y-axis shows statistical significance (-log10) and x-axis the log2fold change (FC) compared to Mef2-GAL4 control (see Supplementary file 1). STRIPAK complex components are highlighted in red. (B) Table showing the log2fold change and p-values of the selected STRIPAK complex components. (C) Simplified schematic of the STRIPAK complex (adapted from Duhart and Raftery, 2020). Proteins identified in the Dlg5-GFP pull-down are coloured. (D) Flight muscles at 32 hr and 90 hr APF of wild type, Cka (independent RNAi lines IR-1 and 2) or Strip knockdown genotypes stained for actin. Note the compaction defect at 32 hr APF followed by muscle atrophy. Scale bars represent 50 µm for 32 hr and 100 µm for 90 hr APF. (E) Box plot showing dorsal-longitudinal flight muscle (DLM) fiber length at 32 hr APF of the indicated genotypes. Each dot is the average from one pupa. Student’s t-test, *** p-value<0.001, ** p-value<0.01.
To functionally test if members of the STRIPAK complex other than Dlg5 and Slmap play a similarly important role in flight muscles, we knocked-down various component members and found that knock-down of Striatin (Cka) and Strip indeed result in very similar phenotypes to Dlg5 and Slmap knock-down: flight muscles at 32 hr APF are longer than wild type, suggesting a fiber compaction defect, and undergo muscle atrophy after 32 hr APF (Figure 3D,E). Together, these data show that Dlg5 interacts with the STRIPAK complex in flight muscles, of which several members including Slmap are important for flight muscle morphogenesis.
The Hippo pathway regulates the developmental timing of muscle morphogenesis
As the STRIPAK complex was shown to dephosphorylate and thus inactivate Hippo (Ribeiro et al., 2010; Zheng et al., 2017), we wondered if the muscle morphogenesis phenotype we observed could be linked to a function of the Hippo pathway in growing flight muscles. When knocking-down the Hippo pathway transcriptional co-activator yorkie in muscles, we observed a failure of muscle compaction at 32 hr APF, flight muscle atrophy at 48 hr APF and consequently flightless adults (Figure 4A,B, Figure 4—figure supplement 1A, Supplementary file 1). The myofiber compaction defect of yorkie knock-down muscles is corroborated by fiber cross-sections at 24 hr and 32 hr APF revealing much thinner muscles and phenocopying the Dlg5 and Slmap loss of function (Figure 4—figure supplement 1B).
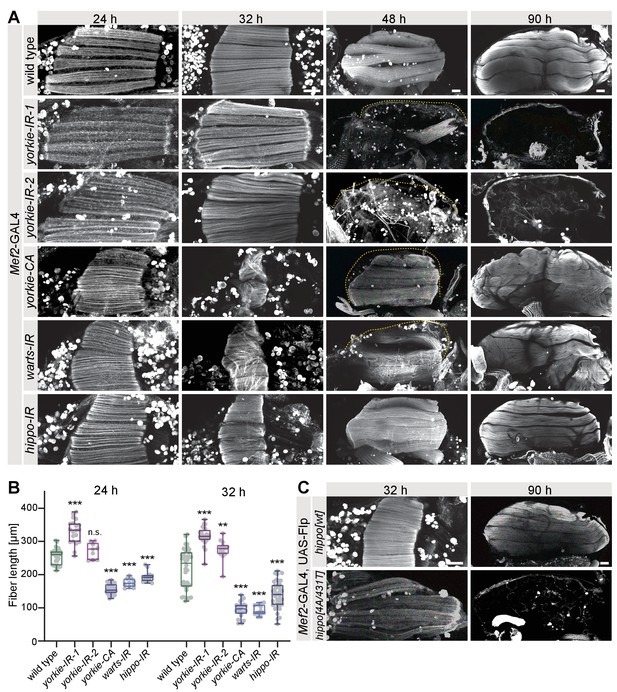
The Hippo pathway regulates muscle morphogenesis.
(A) Flight muscles at 24 hr, 32 hr, 48 hr, and 90 hr after puparium formation (APF) from wild type, yorkie knock-down (independent RNAi lines IR-1 and 2), yorkie-CA, as well as warts and hippo knockdown genotypes stained for actin. The dotted lines highlight the cuticle. Note the too long yorkie-IR muscles but too short yorkie-CA, warts-IR, and hippo-IR muscles at 24 hr and 32 hr APF. (B) Box plot showing muscle fiber length at 24 hr and 32 hr APF of the indicated genotypes. Student’s t-test, *** p-value<0.001, **p value<0.01. (C) Flight muscles at 32 hr and 90 hr APF from pupae expressing either wild-type hippo or Slmap-binding-deficient hippo[4A/431T] (under control of the milder tubulin promoter). The FRT stop cassette is removed by muscle-specific Mef2-GAL4-driven Flp recombinase. All scale bars represent 50 µm.
In contrast to the myofiber compaction defect of yorkie knock-down muscles, expression of an activated form of Yorkie (yorkie-CA), which cannot be phosphorylated by Warts, results in premature muscle fiber compaction already at 24 hr APF and strongly hyper-compacted muscle fibers at 32 hr APF, and later in disorganised myofibers (Figure 4A,B). The increased cross-sectional area is particularly obvious in cross-sections of yorkie-CA fibers (Figure 4—figure supplement 1B). Importantly, we observed the same phenotypes after knock-down of each of the two kinases hippo and warts, both negative regulators of Yorkie nuclear entry (Figure 4A,B). Consistently, a transcriptomics time-course of dissected developing flight muscles (Spletter et al., 2018) showed that Dlg5, Slmap, hippo, warts, yorkie and also the transcriptional activator scalloped are all expressed in developing flight muscles (Figure 4—figure supplement 1C). This strongly suggests that the Hippo pathway, by regulating phosphorylation of the transcriptional co-activator Yorkie, is essential for the correct developmental timing of flight muscle morphogenesis: too much active Yorkie accelerates myofiber compaction, while too little active Yorkie blocks it.
To further investigate how the STRIPAK complex may regulate the Hippo pathway in flight muscles, we investigated Hippo protein localisation in relation to Slmap protein. We find that the available Hippo antibody does give specific staining in flight muscles at 32 hr APF, which is strongly reduced in the hippo knock-down flight muscle (Figure 4—figure supplement 1D). Detailed imaging revealed that Hippo localises in a dotted pattern in flight muscles at 32 hr APF, with the dots often being in proximity to Slmap-positive structures; however, we found little overlap of both (Figure 4—figure supplement 1E).
Hence, we wanted to corroborate that the STRIPAK complex directly regulates the Hippo pathway in flight muscles with additional genetic evidence and used a recently characterised hippo construct (hippo[4A/431T]), which lacks the four auto-phosphorylation sites in Hippo required to bind to the STRIPAK phosphatase complex via Slmap. This Hippo[4A/431T] protein cannot be dephosphorylated on regulatory T195 by STRIPAK and thus is constitutively active (Zheng et al., 2017). Interestingly, expression of hippo[4A/431T] in muscle after Mef2-GAL4 driven flip-out also resulted in a muscle fiber compaction defect at 32 hr APF and muscle atrophy thereafter, phenocopying the STRIPAK and yorkie loss-of-function phenotypes (Figure 4C). Taken all these data together, we conclude that Dlg5 and members of the STRIPAK complex are key regulators of the Hippo pathway, which controls the developmental timing of flight muscle morphogenesis in Drosophila.
The Hippo pathway is required post-mitotically in flight muscle fibers
Indirect flight muscles are formed by fusion of several hundred myoblasts until 24 hr APF (Weitkunat et al., 2014). These myoblasts emerge during embryonic development and proliferate extensively during larval stages (Bate et al., 1991; Gunage et al., 2014; Roy and VijayRaghavan, 1998). As knock-down of Dlg5, STRIPAK complex, and Hippo pathway members with Mef2-GAL4 results in flightless animals and not in lethality (except for warts, see Figure 4—figure supplement 1A), it is unlikely that general myoblast proliferation during larval stages is affected, which would result in defects of all adult muscles. However, since Mef2-GAL4 is already active during larval stages, we wanted to exclude that the observed muscle phenotypes are caused by myoblast proliferation defects during larval stages. Hence, we conditionally activated GAL4 only during pupal stages using temperature sensitive GAL80 (GAL80ts, see Materials and methods) (McGuire et al., 2003) and quantified myoblast fusion rates by counting the nuclei of DLM4. We found comparable numbers of nuclei at 24 hr APF ruling out a major contribution of myoblast proliferation or fusion defect to the phenotype (Figure 5—figure supplement 1A,B).
Importantly, these GAL80ts Mef2-GAL4 Dlg5 and yorkie knock-down muscles do display the same fiber compaction defect as observed with Mef2-GAL4 resulting in longer but thinner fibers with grossly comparable volumes at 24 hr APF (yorkie-IR is slightly smaller) (Figure 5A,B). These fibers do not compact at 32 hr APF and undergo atrophy leading to no remaining fibers at 48 hr or 90 hr APF (Figure 5C,D), phenocopying the constitutive knock-down of Dlg5 or yorkie. Conversely, conditional expression of yorkie-CA during pupal stages results in premature compaction at 24 hr APF and very short fibers at 32 hr APF that grow to disorganised fibers at 90 hr APF (Figure 5A–D). These phenotypes resemble the constitutive Mef2-GAL4-driven phenotypes demonstrating a role for Dlg5 and yorkie in muscle fibers during pupal stages.
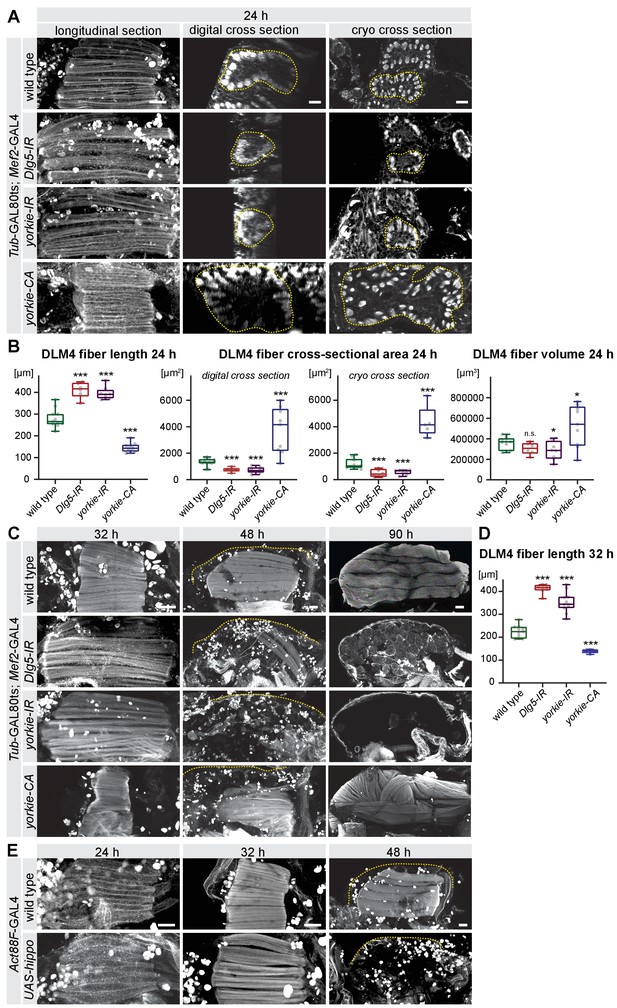
The Hippo pathway is important for post-mitotic muscle development.
(A–D) Developing flight muscles at 24 hr, 32 hr, 48 hr, and 90 hr after puparium formation (APF) from wild type, Dlg5-IR, yorkie-IR, and yorkie-CA TubGAL80ts Mef2-GAL4 pupae, in which GAL4 activity was restricted to pupal stages (shift to 31°C at 0 hr APF). (A) Longitudinal sections of all flight muscles, as well as digital and cryo cross-sections of dorsal-longitudinal flight muscle 4 (DLM4) at 24 hr APF. Dotted lines highlight the fiber area. (B) Box plot displaying DLM4 muscle fiber length, fiber cross-sectional area from digital and cryo cross-sections as well as the calculated DLM4 fiber volume at 24 hr APF (calculated by multiplying length with digital cross-sectional area for each pupa). Student’s t-test, *** p-value<0.001, * p-value<0.05. (C) Flight muscles at 32 hr, 48 hr, and 90 hr APF. Note the too long muscles in Dlg5-IR, yorkie-IR at 32 hr APF followed by muscle atrophy and the too short yorkie-CA fibers at 32 hr that develop disorganised fibers at 90 hr APF. (D) Box plot illustrating the average muscle fiber length at 32 hr APF. Student’s t-test, *** p-value<0.001. (E) Control and Act88F-GAL4 UAS-hippo flight muscles at 24 hr, 32 hr and 48 hr APF. Note the induced fiber compaction defect followed by the muscle atrophy. All scale bars represent 50 µm for longitudinal sections and 10 µm for cross-sections.
To further corroborate a post-mitotic role of the Hippo pathway in muscle fibers, we over-expressed hippo with the strong, strictly post-mitotic flight muscle specific driver Act88F-GAL4, which is only active after myoblast fusion (Bryantsev et al., 2012; Spletter et al., 2018). This post-mitotic over-expression of hippo resulted in flight muscle compaction defects at 32 hr APF and muscle atrophy at 48 hr APF (Figure 5E). Together, these data demonstrate that the Hippo pathway and its regulator Dlg5 are required post-mitotically in flight muscle fibers for the correct timing of morphogenesis.
The Hippo pathway regulates post-mitotic muscle fiber growth
The wild-type flight muscle fibers grow in volume from 24 hr to 48 APF (see Figure 1), while yorkie or Dlg5 knock-down fibers undergo atrophy after 32 hr APF. As the Yorkie activity is known to suppress apoptosis in epithelial tissues (Harvey and Tapon, 2007) we asked if we could rescue fiber atrophy by over-expressing the apoptosis inhibitor Diap1. Indeed, over-expression of Diap1 using UAS-Diap1 but not of a control construct (UAS-GFP-Gma) during pupal stages in GAL80ts Mef2-GAL4 (hereafter abbreviated as GAL80ts) yorkie or Dlg5 knock-down fibers substantially rescues fiber atrophy, often resulting in the normal number of six muscle fibers at 48 hr APF (Figure 6A, Figure 6—figure supplement 1A). Furthermore, over-expression of the exogenous apoptosis inhibitor p35 (Clem et al., 1991) also results in six muscle fibers at 48 hr APF (Figure 6—figure supplement 1B,C). This demonstrates that apoptosis contributes to flight muscle fiber atrophy in yorkie and Dlg5 knock-down muscles.
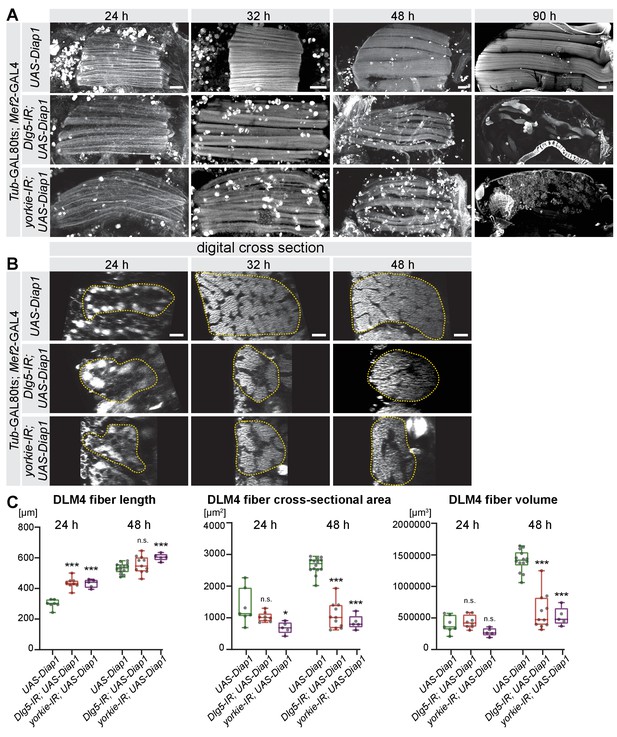
The Hippo pathway is essential for post-mitotic muscle fiber growth.
(A, B) Longitudinal sections of all dorsal-longitudinal flight muscles (DLM) at 24 hr, 32 hr, 48 hr, and 90 hr after puparium formation (APF) (A) and digital cross-sections of DLM4 at 24 hr, 32 hr, and 48 hr APF (B) from wild type, Dlg5-IR and yorkie-IR TubGAL80ts Mef2-GAL4 UAS-Diap1 (shifted to 31°C at 0 hr APF) stained for actin. Scale bars represent 50 µm for longitudinal sections and 10 µm for cross-sections. In B dotted lines highlight the fiber areas. (C) Box plots showing DLM4 fiber length, digital cross-sectional area, and volume (calculated by multiplying length with digital cross-sectional area for each pupa) at 24 hr and 48 hr APF. Student’s t test, *** p-value<0.001, * p-value<0.05.
Presence of muscle fibers at 48 hr APF enabled us to quantitatively investigate the role of the Hippo pathway during the post-mitotic muscle fiber growth. As in Figure 5, we used digital cross-sections of large confocal stacks to quantify the cross-sectional area of DLM4 and together with the fiber length calculated the fiber volume (Figure 6A–C). Similar to what we showed in Figure 5, GAL80ts Diap1-expressing control fibers have a comparable volume to GAL80ts Diap1-expressing yorkie or Dlg5 knock-down fibers at 24 hr APF (Figure 6A–C), showing that they start into the muscle growth phase with comparable sizes.
However, until 32 hr APF, Diap1 control muscles increase their cross-sectional area followed by growth in length until 48 hr APF to increase their volume about fourfold within 24 hr (Figure 6A–C). Interestingly, GAL80ts Diap1-expressing yorkie or Dlg5 knock-down muscles fail to normally increase their cross-sectional area at 32 hr and 48 hr APF, thus resulting in smaller volumes at 48 hr APF (Figure 6A–C). Not all these thin muscles do survive until 90 hr APF despite over-expression of the apoptosis inhibitors Diap1 or p35 (Figure 6A and Figure 6—figure supplement 1B). Taken together, these data provide strong evidence that the Hippo pathway and its transcriptional co-activator Yorkie are required to enable normal post-mitotic growth of flight muscle fibers, likely by regulating the developmental timing of muscle morphogenesis.
The Hippo pathway is essential for myofibrillogenesis triggering muscle growth
To investigate the molecular mechanism of the muscle growth defect in detail, we quantified myofibrillogenesis. Control Diap1-expressing muscles have assembled immature myofibrils at 32 hr APF (Weitkunat et al., 2014). These immature myofibrils are continuous and thus can be easily traced throughout the entire field of view (Figure 7A,B, Figure 7—figure supplement 1A). In contrast, GAL80ts Diap1-expressing yorkie or Dlg5 knock-down muscles fail to properly assemble their myofibrils at 32 hr APF resulting in only short myofibril traces (Figure 7A,B, Figure 7—figure supplement 1A). Concomitant with the myofibril assembly defect, we also found that the spacing of the nuclei is defective. In control muscle fibers the nuclei are present mainly as single rows located between myofibril bundles, whereas in yorkie and Dlg5 knock-down muscles they form large centrally located clusters (Figure 7—figure supplement 1B). This indicates that at 32 hr APF, Hippo signalling is required within the muscle fibers to trigger proper myofibril assembly and nuclear positioning.
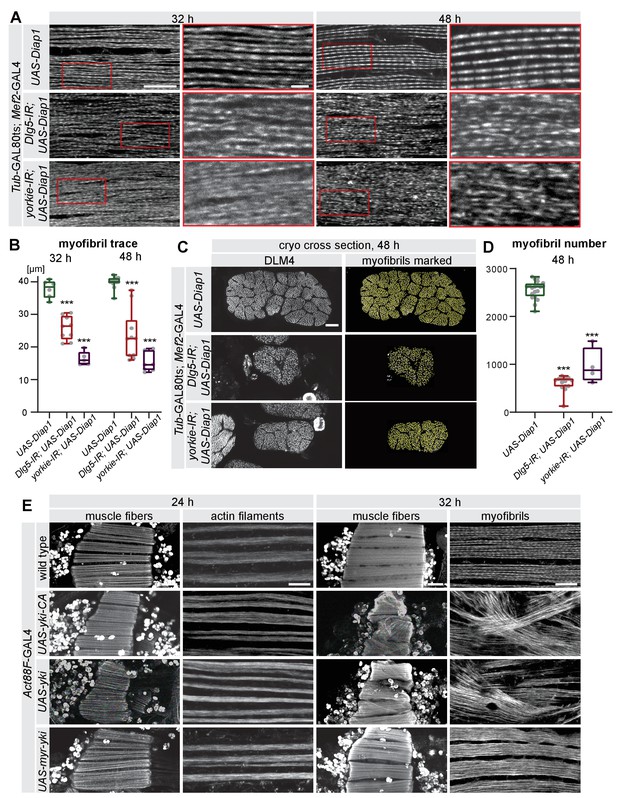
The Hippo pathway is essential for myofibrillogenesis.
(A) Myofibrils visualised by phalloidin from control, Dlg5-IR, and yorkie-IR UAS-Diap1 TubGAL80ts Mef2-GAL4 muscles at 32 hr and 48 hr after puparium formation (APF; shifted to 31°C at 0 hr APF). The red-boxed areas are magnified. Note the less regular myofibril pattern of Dlg5-IR and yorkie-IR muscles at 32 hr APF that makes it hard to trace an individual myofibril (see Figure 7—figure supplement 1A). Even at 48 hr APF, myofibrils from Dlg5-IR and yorkie-IR are hard to trace continuously. Scale bars represent 10 µm in the overviews and 2 µm in the zoomed red boxes. (B) Box plot of traced myofibril length in a 40 × 20 × 2.5 µm volume (see Figure 7—figure supplement 1A). Student’s t test, *** p-value<0.001. (C) Cryo cross-sections of dorsal-longitudinal flight muscle 4 (DLM4) from control, Dlg5-IR and yorkie-IR UAS-Diap1 TubGAL80ts Mef2-GAL4 muscles at 48 hr APF (shifted to 31°C at 0 hr APF). Yellow dots represent the myofibrils recognised by the MyofibrilJ plug-in to automatically count the number of myofibrils per DLM4 fiber (Spletter et al., 2018). Scale bar represents 10 µm. (D) Box plot of myofibril number in DLM4 of indicated genotypes at 48 hr APF. Student’s t test, *** p-value<0.001. (E) Flight muscle and myofibril morphologies of muscles expressing yorkie-CA, yorkie, or myr-yorkie under the control of post-mitotic Act88F-GAL4 at 24 hr and 32 hr APF. Scale bars represent 50 µm in muscle fiber images and 10 µm in myofibril images.
The myofibril defect becomes even more pronounced at 48 hr APF when control myofibrils have matured and sarcomeres are easily discernible (Figure 7A), while no organised sarcomeres are present in GAL80ts yorkie and Dlg5 knock-down muscles at 48 hr APF and myofibril traces remain short (Figure 7A,B, Figure 7—figure supplement 1A). Furthermore, cryo cross-sections revealed that not only the cross-sectional area but also the total number of myofibrils is strongly reduced in GAL80ts yorkie and Dlg5 knock-down muscles compared to control (Figure 7C,D, Figure 7—figure supplement 1C). As myofibril number is determined at the assembly stage at 32 hr APF in wild type (Spletter et al., 2018) these data demonstrate that the Hippo pathway controls both the morphological quality of the myofibrils at the assembly and maturation stages as well as their quantity. As myofibrils occupy most of the muscle fiber space, their reduced amount likely causes the reduced muscle size in Dlg5 or yorkie knock-down fibers.
To further substantiate that the amount and quality of myofibrils are important for normal muscle growth, we aimed for an independent way of changing myofibril number and assessing muscle fiber size. It had been shown that mechanical tension in flight muscles is a prerequisite for normal myofibrillogenesis (Weitkunat et al., 2014). Upon hypomorphic knock-down of the muscle attachment protein Kon-tiki (kon), muscle-tendon attachment is compromised, hence mechanical tension build-up is perturbed and only fewer myofibrils can assemble (Weitkunat et al., 2014). Consistent with our results after yorkie or Dlg5 knock-down we find that kon knock-down also results in a fiber compaction defect, caused by reduced mechanical tension at 32 hr APF (Figure 7—figure supplement 1D), and as a consequence in a strongly reduced fiber cross-sectional area and volume at 48 hr APF (Figure 7—figure supplement 1D–F). Taken together, these data strongly suggest that both mechanical tension and regulated Yorkie activity are required for normal myofibril morphogenesis to support muscle fiber growth.
Yorkie is a transcriptional co-regulator in muscle fibers
It was recently shown that the transcription of most sarcomere key components is tightly regulated starting shortly before myofibril assembly and being strongly boosted during myofibril maturation (Spletter et al., 2018). Thus, we reasoned that Yorkie activity may be involved in this transcriptional regulation step to control the timing of myofibrillogenesis. However, it had also been recently shown that Yorkie can regulate myosin contractility directly at the cell membrane without entering into the nucleus (Xu et al., 2018). As we have thus far failed to unambiguously locate Yorkie protein in muscle fibers during development, we used genetic tools to address this important point. To test whether Yorkie may play a role outside of the nucleus, we manipulated Yorkie levels by over-expressing different Yorkie variants post-mitotically using Act88F-GAL4 and investigated the consequences at 24 hr and 32 hr APF. Over-expression of either Yorkie-CA, whose import into the nucleus is uncoupled from the Hippo pathway, or wild-type Yorkie, whose nuclear import is regulated by Hippo, both result in premature muscle fiber compaction at 24 hr APF, with seemingly normal actin filaments, phenocopying the Gal80ts condition (Figure 7E). Strikingly, the muscle fiber hyper-compaction at 32 hr APF coincides with a chaotic organisation of the myofibrils, with many myofibrils not running in parallel but in various directions (Figure 7E, Figure 7—figure supplement 2A). This suggests that the hyper-compaction phenotype upon Yorkie over-expression is likely caused by uncontrolled and premature force production of the chaotically assembling myofibrils.
In contrast, over-expression of a membrane-anchored myristoylated form of Yorkie, which has been shown to activate myosin contractility at the epithelial cell cortex without going into the nucleus (Xu et al., 2018), does not result in premature muscle fiber compaction at 24 hr APF. Furthermore, these muscles display normally oriented parallel myofibrils at 32 hr APF (Figure 7E, Figure 7—figure supplement 2A). These results indicate that the observed myofibril and fiber compaction defects are caused by a transcriptional response of Yorkie in the nucleus.
This interpretation is corroborated by loss-of-function data of the transcriptional activator scalloped (sd), which is the essential transcriptional co-factor of Yorkie in the nucleus. Knock-down of scalloped results in severe muscle atrophy and no remaining muscles at 90 hr APF (Figure 7—figure supplement 2B). Together, these genetic data suggest that Hippo signalling regulates Yorkie phosphorylation and thus its nuclear entry to trigger a transcriptional response that controls myofibril development and muscle fiber growth.
The Hippo pathway controls expression of key sarcomere components
To investigate the transcriptional role of the Hippo pathway during flight muscle development, we performed muscle-specific transcriptomics of wild-type flight muscles compared to different yorkie ‘loss-of-function’ (Dlg5-IR, Slmap-IR, and yorkie-IR) and yorkie ‘gain-of-function’ (yorkie-CA and hippo-IR) conditions. We dissected flight muscles from 24 hr and 32 hr APF, isolated RNA and applied a sensitive 3-prime end mRNA sequencing method (BRB-seq) (Alpern et al., 2019), which handles small amounts of mRNA (see Materials and methods). We found a clustering of biological replicates and similar genotypes using principal component analysis and comparable read count distributions across all samples (Figure 8—figure supplement 1). This verifies BRB-seq as a reliable method to quantitatively compare gene expression from small amounts of developing muscle tissue across multiple samples.
We applied the selection criteria log2FC > 1 and adjusted p-value<0.05 to identify differentially expressed genes compared to wild type (Supplementary file 2). Applying FlyEnrichr (Kuleshov et al., 2016) on the differential data sets, we found a strong enrichment for muscle and, in particular, for sarcomere and myofibril Gene Ontology terms (GO-terms) in the differentially expressed genes of all three yorkie ‘loss-of-function’ muscle genotypes (Dlg5-IR, Slmap-IR, and yorkie-IR) at 24 hr APF (Figure 8A, Supplementary file 3). Importantly, expression of many core sarcomeric components, including both titin homologs sallimus (sls) and bent (bt), Myosin heavy chain (Mhc), Myofilin (Mf), Paramyosin (Prm), tropomyosins (Tm1, Tm2), flight-muscle-specific actin (Act88F) and Obscurin (Unc-89), as well as sarcomere dynamics regulators, including myosin phosphatase (Mbs), a flight muscle formin (Fhos) and the spektraplakin shortstop (shot) are consistently reduced in yorkie ‘loss-of-function’ muscle genotypes at 24 hr APF (Figure 8B). Furthermore, expression of mRNAs coding for proteins linking the nuclei to the cytoskeleton, such as the Nesprin family members klar and Msp300, are also strongly reduced (Figure 8B), which may explain the observed nuclei positioning defect in yorkie and Dlg5 knock-down muscles (Figure 7—figure supplement 1B). Msp300 and Prm are amongst the only six genes that are significantly downregulated in all three loss-of-function conditions at 24 hr APF (Supplementary file 2). This strongly suggests that nuclear entry of Yorkie contributes to the transcriptional induction of sarcomeric protein coding genes as well as genes important to link the nuclei to the sarcomeres. This transcriptional induction was shown to precede sarcomere assembly (Spletter et al., 2018) and thus may provide a molecular explanation of the observed flight muscle compaction and myofibril assembly defects of Dlg5-IR, Slmap-IR, and yorkie-IR muscles.
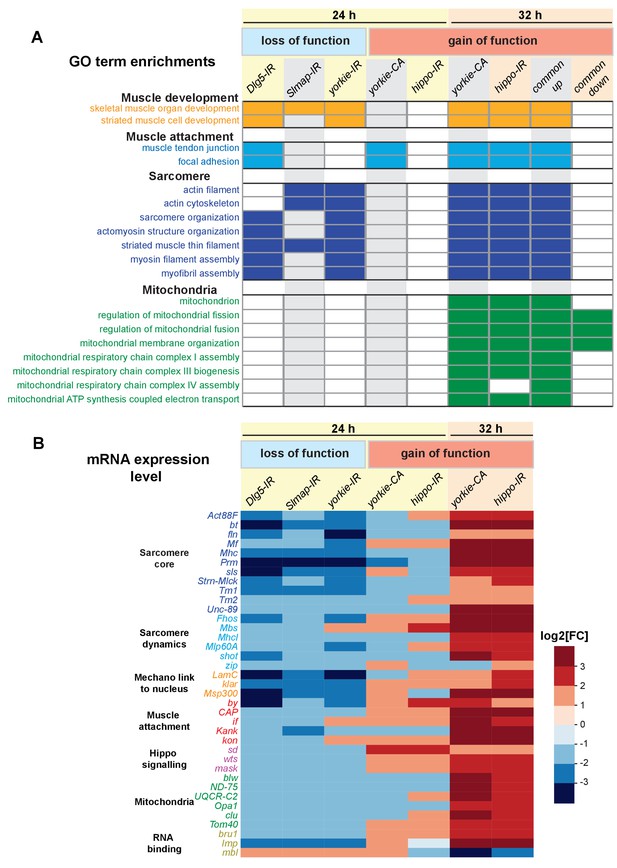
Yorkie transcriptionally controls sarcomeric and mitochondrial genes expression.
(A) Gene ontology (GO)-term enrichments in genes lists that are significantly changed in the various loss (Dlg5-IR, Slmap-IR, yorkie-IR) and gain-of-function (yorkie-CA, hippo-IR) yorkie conditions at 24 hr and 32 hr after puparium formation (APF) compared to wild-type controls (see Supplementary file 3). Note the strong enrichment of sarcomere related GO-terms in the 24 hr APF loss-of-function and the 32 hr APF gain-of-function condition. Thirty-two hr APF gain of function is also strongly enriched for mitochondrial GO-terms. (B) Plot displaying the log2-fold change of transcript levels for individual genes of the above genotypes compared to control (up in red, down in blue). Note the strong downregulation of the sarcomeric genes in the 24 hr APF loss-of-function conditions, in particular the titin homologs bt and sls, as well as Mhc, Prm and Act88F.
To complement the yorkie loss-of-function conditions, we also performed BRB-seq transcriptomics comparing yorkie ‘gain-of-function’ conditions (yorkie-CA and hippo-IR) to control. While we found few significantly differentially expressed genes at 24 hr APF (including an induction of the transcriptional activator scalloped) (Supplementary file 2), we identified many significant changes in mRNA expression in yorkie-CA and hippo-IR myofibers at 32 hr APF (Supplementary file 2). Strikingly, sarcomeric core components and their regulators are amongst the top upregulated genes on both lists (Figure 8B, Supplementary file 2). Consequently, GO-term analysis of the differentially expressed genes identified a strong enrichment for sarcomere and myofibril GO-terms (Figure 8A, Supplementary file 3). In addition to sarcomeric genes, genes important for myofibril attachment at muscle-tendon junctions, including the integrin attachment complex members kon, if, CAP, by, and Kank are upregulated in both gain-of-function genotypes (Figure 8B). Furthermore, we found an upregulation of Hippo signalling regulators mask, which is required for efficient nuclear import of Yki (Sidor et al., 2019), and of the negative regulator wts (Figure 8B). This demonstrates that regulated Hippo activity is required to control Yorkie in order to tune expression of mRNAs coding for sarcomeric and myofibril attachment proteins.
During the stage of myofibril assembly, the mitochondria morphology changes and the expression of mitochondrial genes increase (Avellaneda et al., 2020; Spletter et al., 2018). Consistently, we found an induction of mitochondria dynamics and protein import regulators (Opa1, Tom40) in hippo-IR and yorkie-CA myofibers at 32 hr APF as well as a consistent upregulation of mRNAs coding for respiratory chain components, including the F1F0 ATP synthase complex (complex V) subunit blw, the NADH dehydrogenase (ubiquinone) subunit ND-75 and the Ubiquinol-cytochrome c reductase subunit UQCR-C2, which are all required to boost ATP production during muscle fiber growth (Figure 8A,B, Supplementary file 2). Taken together, these data strongly suggest that the Hippo pathway regulates the correct expression dynamics of many key muscle components, most prominently mRNAs coding for core sarcomeric and mitochondrial proteins to enable myofibril assembly and mitochondrial maturation.
Yorkie controls sarcomeric protein dynamics
The most prominent phenotypes of the yorkie ‘loss-of-function’ group (Dlg5-IR, Slmap-IR and yorkie-IR) are defective muscle fiber compaction and severe myofibril assembly defects at 32 hr APF. As the core sarcomeric proteins actin and myosin are required to assemble myofibrils and build-up mechanical tension (Loison et al., 2018; Weitkunat et al., 2014), we chose to quantify protein levels of the major actin isoform in flight muscles Actin88F (Act88F) as well as the only Drosophila muscle Myosin heavy chain (Mhc). For both, we used GFP fusion proteins expressed under endogenous control (Sarov et al., 2016) and thus avoiding the variations often seen in antibody stainings (see Materials and methods). Consistent with the transcriptomics data, we found a mild reduction of Mhc and Act88F protein levels in yorkie knock-down muscles at 24 hr APF, which became more pronounced at 32 hr APF (Figure 9A–C). Furthermore, we have investigated protein levels for the myosin-binding protein Paramyosin (Prm) and the titin homolog Sls also using GFP fusion proteins. We found a reduction of Sls-GFP at 24 hr APF and a severe reduction of Prm-GFP at 32 hr APF in yorkie knock-down muscles (Figure 9—figure supplement 1A–C). Together, these data are consistent with the myofibril assembly defects found in yorkie knock-down muscles at 32 hr APF.
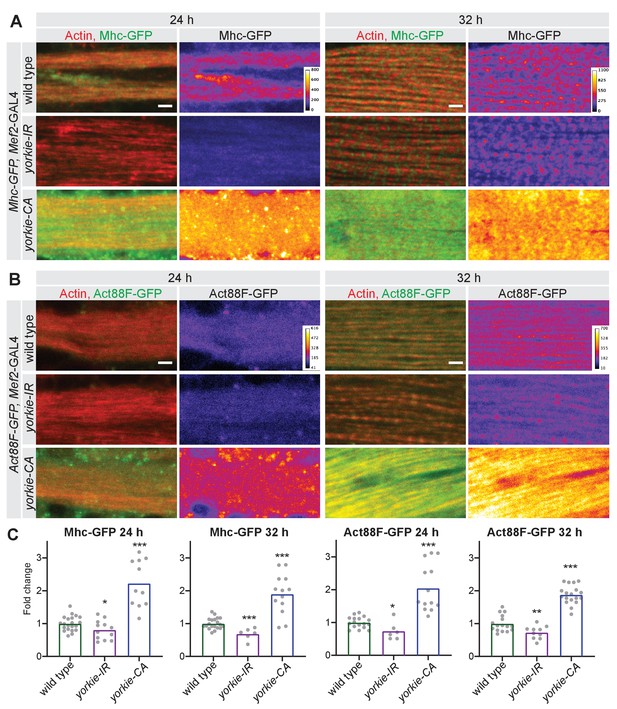
Yorkie regulates sarcomere protein expression.
(A, B) Mhc-GFP (A) and Act88F-GFP (B) proteins levels visualised with a GFP nanobody together with actin comparing wild-type control with yorkie-IR and yorkie-CA at 24 hr and 32 hr after puparium formation (APF). Scale bars represent 2 µm. GFP channel is displayed using the ‘fire’ look-up table. (C) Quantification of relative GFP protein levels compared to wild type (wild-type mean set to one for each individual experiment). Bar plots display average normalised value and each dot represents one hemithorax. Student’s t test, *** p-value<0.001, ** p-value<0.01 * p-value<0.05.
Surprisingly, constitutive activation of yorkie (yorkie-CA) results in a boost of Mhc-GFP, Act88F-GFP, Sls-GFP, and Prm-GFP protein expression already at 24 hr APF, which is maintained at 32 hr APF (Figure 9A–C, Figure 9—figure supplement 1A–C). This increased acto-myosin and titin expression may provide the molecular explanation of the premature compaction phenotype seen in yorkie-CA muscles at 24 hr APF. Despite being expressed at high levels already at 24 hr APF, the proteins fail to prematurely assemble into well-organised periodic myofibrils in yorkie-CA (Figure 9A,B, Figure 9—figure supplement 1A,B). Furthermore, the myofibrils present in yorkie-CA at 32 hr APF are less regularly organised compared to control, with actin showing less pronounced periodicity (Figure 7—figure supplement 2A). These ectopic localisation patterns of actin, myosin, and titin may explain muscle hyper-compaction at 32 hr APF and the chaotic arrangement of myofibrils in yorkie-CA 90 hr APF (see Figure 5C). Taken together, these data provide strong evidence that the Hippo pathway and its transcriptional regulator Yorkie contribute to the timing of myofibril assembly by regulating correct timing and levels of sarcomeric protein expression.
Discussion
The Hippo pathway and post-mitotic muscle fiber growth
Muscle fibers are often enormously large cells, which are densely packed with force producing contractile filaments and ATP-producing mitochondria (Willingham et al., 2020). Thus, it appears logical that myofibril and mitochondria content primarily contribute to the size of an individual muscle fiber. To explore the link between myofibrillogenesis and muscle fiber growth, we used the largest Drosophila muscle cells, the indirect flight muscles, which are formed by fusion of several hundred myoblasts per muscle fiber and grow more than 10 times in volume after fusion in less than 72 hr. We provide strong genetic evidence that the regulation of Yorkie nuclear activity by the Hippo pathway is essential to allow post-mitotic flight muscle growth. Loss of function of Yorkie or one of the upstream STRIPAK complex members Slmap, Strip, and Cka, as well as Dlg5, that regulate Hippo activity, all show the same phenotype: muscle atrophy during the post-mitotic muscle growth phase. Atrophy can be partially suppressed by over-expression of the apoptosis inhibitors Diap1 or p35, which then results in small flight muscle fibers, emphasizing the essential role of Yorkie in promoting flight muscle growth.
How general is this role of Yorkie and the Hippo pathway in muscle fiber growth? Interestingly, muscle-specific loss (Dlg5-IR, Slmap-IR and yorkie-IR) and gain of function of Yorkie (hippo-IR, yorkie-CA) results in viable but flightless animals. This suggests that the larval muscles and the other adult Drosophila muscle fibers such as leg and abdominal muscles can form and function normally in the absence of Yorkie and the Hippo pathway. One reason might be their slower growth rates and their more limited sizes compared to indirect flight muscles. A second reason may relate to the particular myofibrillogenesis mechanism in flight muscles. Flight muscles display individual distinct myofibrils, which all form simultaneously at about 32 hr APF (Weitkunat et al., 2014) and then grow in length and diameter to match the volume increase of the muscle fiber (Spletter et al., 2018).
The closest mammalian homolog to insect flight muscles is the mammalian heart. Similar to flight muscles, the heart is a very stiff muscle using a stretch-modulated contraction mechanism (Shiels and White, 2008). After birth, mammalian cardiomyocytes stop dividing and all organ growth is achieved post-mitotically by size increase of the individual contractile cells. Interestingly, it was shown that mammalian YAP1 can promote cardiomyocyte survival and growth, as post-mitotic deletion of YAP1 results in increased fibrosis and cardiomyocyte apoptosis (Del Re et al., 2013). However, a role for the Hippo pathway in mammalian muscle is not limited to the heart. Constitutive expression of active YAP in adult mouse muscle fibers induces muscle fiber atrophy and deterioration of muscle function (Judson et al., 2013). Furthermore, it has been shown that the mammalian Hippo homolog Mst1 is a key regulator in fast skeletal muscle atrophy (Wei et al., 2013), and more importantly YAP promotes muscle fiber growth by its transcriptional activity requiring Tead cofactors (Watt et al., 2015). This suggests that the Hippo pathway via its transcriptional regulators Yorkie/YAP/TAZ is a general regulator of muscle fiber growth and survival in animals.
Yorkie targets
How does Yorkie mediate flight muscle growth? Our transcriptomics analysis revealed that mRNAs coding for sarcomeric and mitochondrial components are major transcriptional targets of Yorkie in flight muscle. In flies and mammals, Yorkie/YAP/TAZ require transcriptional cofactors of the Tead family (TEA domain containing) to bind to DNA. Interestingly, it has been shown that mammalian homologs Tead1-4 bind to a DNA motif called ‘muscle CAT’ (MCAT), a motif well-known to regulate cardiac skeletal sarcomeric protein expression, including cardiac Troponin T or cardiac actin (Carson et al., 1996; Farrance and Ordahl, 1996; Farrance et al., 1992; Wackerhage et al., 2014). The only Drosophila Tead family member Scalloped binds to a very similar MCAT motif (Wu et al., 2008), and we found loss of scalloped shows the same phenotype as loss-of-function of yorkie. This strongly suggests that Yorkie and Scalloped cooperate in Drosophila muscle to transcriptionally boost sarcomeric gene expression to enable myofibril assembly and flight muscle fiber growth.
It has been shown that flight muscle fate is determined by the zinc finger transcriptional regulator Spalt major (Schönbauer et al., 2011). This includes the regulation of all flight muscle-specific sarcomeric components. How Yorkie cooperates with Spalt is to date an open question. One interesting link is that Yorkie and the Hippo pathway are required for the normal expression of bruno1 (bru1, see Figure 8B). Bruno is the major splice regulator of flight muscle alternative splicing of sarcomeric proteins, downstream of Spalt (Spletter et al., 2015). Additionally, Bruno was shown to bind to 3’-UTRs of mRNA to regulate their translation efficiencies (Webster et al., 1997). Another RNA-binding protein that requires the Hippo pathway for normal expression is Imp (IGF-II mRNA-binding protein, see Figure 8B). Imp has been shown to regulate the stability and translation of a number of F-actin regulators (Medioni et al., 2014), suggesting that post-transcriptional effects of Hippo signalling can play an important role, too. This may explain the strong upregulation of Mhc, Act88F, Sls, and Prm proteins levels in yorkie-CA muscle at 24 hr APF despite little changes at the mRNA level. A similar crosstalk between the Hippo pathway and translational regulation via the mTORC1 complex has been suggested in mammals (Hansen et al., 2015).
Regulation of the Hippo pathway
How is the Hippo pathway regulated to induce sarcomeric protein expression at the correct time during muscle development? Salvador, Expanded, Merlin, and Kibra, which regulate activity or plasma membrane localisation of Hippo in epithelial cells, all appear not to be required in flight muscles (Schnorrer et al., 2010; and data not shown). However, we find that the core members of the STRIPAK complex Slmap, Strip and Cka as well as Dlg5 are required to regulate Hippo in muscle. Interestingly, Strip and Cka have been shown to bind to each other and regulate either Hippo or other signalling pathways in other Drosophila tissues including eye, testis or motor neurons (La Marca et al., 2019; Neal et al., 2020; Neisch et al., 2017). Slmap appears to be the specific adaptor to link STRIPAK to Hippo in muscle (our work) and epithelia in flies (Neal et al., 2020; Zheng et al., 2017) as well as in mammalian cell culture (Bae et al., 2017; Kwan et al., 2016). As Slmap has a single transmembrane domain, it is likely localised to vesicles and our antibody stainings are consistent with at least a partial co-localisation to the assembling T-tubules, membrane invaginations that form during myofibrillogenesis and link the plasma membrane to the forming sarcomeres and the sarcoplasmic reticulum (Peterson and Krasnow, 2015; Razzaq et al., 2001; Sauerwald et al., 2019). Slmap may either bind or recruit Dlg5 to membranes, both were interestingly found in a complex with Mst1 (Hippo homolog) in mammalian cell culture (Kwan et al., 2016), which may boost the interaction of STRIPAK with Hippo, resulting in its effective de-phosphorylation. A detailed molecular model of Hippo signalling (Figure 10) remains speculative to date until the regulation of the precise physical interactions between the key components, Hippo, Slmap, Dlg5, and Yorkie have been resolved in developing muscle. Interaction proteomics using dissected flight muscles at different stages may reveal interesting dynamics.
A speculative model how myofibrillogenesis may coordinate with transcription
We have shown that mechanical tension in flight muscles increases during the early phases of myofiber development and identified it as a key regulator of myofibril assembly in flight muscle (Weitkunat et al., 2014). Concomitantly, transcription of sarcomeric components needs to be upregulated to allow myofibril assembly and myofibril maturation. An attractive although speculative model places the Hippo pathway central to coordinate the assembly status of the myofibrils with the transcriptional status of the muscle fiber nuclei (Figure 10). In analogy to the epithelial cell cortex, we hypothesise that mechanical stretch produced by the actin cytoskeleton potentially via membranes, STRIPAK and Dlg5 may inhibit Hippo and thus promote the nuclear localisation of Yorkie, which in turn will boost transcription of mRNAs coding for sarcomeric components. This would be an analogous mechanism to the inhibition of Hippo by high tension produced by the cortical actomyosin and spectrin networks at the epithelial cortex resulting in epithelial tissue growth (Deng et al., 2015; Fletcher et al., 2015; Fletcher et al., 2018; Rauskolb et al., 2014). Interestingly, it was shown that filamentous actin (F-actin) levels can directly regulate Hippo pathway activity; more F-actin blocks Hippo signalling, resulting in epithelial tissue over-growth (Fernández et al., 2011; Sansores-Garcia et al., 2011; Yin et al., 2013). A similar feedback loop may enable the induction of more actin and myosin once the myofibrils have started to assemble and produce high levels of mechanical tension (Figure 10). This feedback model implies that only the successful completion of the myofibril assembly step then allows to fully boost sarcomeric mRNA transcription and translation to enable myofibril maturation and muscle fiber growth. This is consistent with the temporal separation of myofibril assembly and maturation in flight muscles (González-Morales et al., 2019; Shwartz et al., 2016; Spletter et al., 2018) and with the fiber growth defects found here when tension generation and hence myofibrillogenesis are compromised upon kon knock-down. Mechanical control of the Hippo pathway and YAP/TAZ localisation by the actomyosin cytoskeleton is also prominent in mammalian cells (Dupont et al., 2011; Wada et al., 2011). A similar mechanical feedback model as proposed here may therefore also be a relevant mechanism to coordinate post-mitotic cardiomyocyte and skeletal muscle growth or regeneration in mammals.
Materials and methods
Reagent type (species) or resource | Designation | Source or reference | Identifiers | Additional information |
---|---|---|---|---|
Genetic reagent (D. melanogaster) | w- | BDSC: 3605 | ||
Genetic reagent (D. melanogaster) | Dlg5-IR-1 | VDRC: 22496 | Pruned from the VDRC collection | |
Genetic reagent (D. melanogaster) | Dlg5-IR-2 | VDRC: 101596 | ||
Genetic reagent (D. melanogaster) | Dlg5-IR-3 | BDSC: 30925 | w[*]; P{w[+mC]=UAS-Dlg5.RNAi}3, source: Helena Richardson | |
Genetic reagent (D. melanogaster) | Slmap-IR-1 | VDRC: 8199 | ||
Genetic reagent (D. melanogaster) | Slmap-IR-2 | BDSC: 32509 | y[1] sc[*] v[1] sev[21]; P{y[+t7.7] v[+t1.8]=TRiP.HMS00513}attP2 | |
Genetic reagent (D. melanogaster) | Cka-IR-1 | BDSC: 28927 | y[1] v[1]; P{y[+t7.7] v[+t1.8]=TRiP.HM05138}attP2 | |
Genetic reagent (D. melanogaster) | Cka-IR-2 | VDRC: 35234 | ||
Genetic reagent (D. melanogaster) | Strip-IR | BDSC: 34657 | y[1] sc[*] v[1] sev[21]; P{y[+t7.7] v[+t1.8]=TRiP.HMS01134}attP2 | |
Genetic reagent (D. melanogaster) | yorkie-IR-1 | VDRC: 111001 | ||
Genetic reagent (D. melanogaster) | yorkie-IR-2 | VDRC: 40497 | ||
Genetic reagent (D. melanogaster) | warts-IR-1 | VDRC: 111002 | ||
Genetic reagent (D. melanogaster) | warts-IR-2 | BDSC: 41899 | y[1] sc[*] v[1] sev[21]; P{y[+t7.7] v[+t1.8]=TRiP.GL01331}attP2 | |
Genetic reagent (D. melanogaster) | Hippo-IR | VDRC: 104169 | ||
Genetic reagent (D. melanogaster) | sd-IR | VDRC: 101497 | ||
Genetic reagent (D. melanogaster) | kon-IR | VDRC: 106680 PMID:24631244 | ||
Genetic reagent (D. melanogaster) | UAS-Dlg5-GFP | BDSC: 30927 | w[*]; P{w[+mC]=UAS-Dlg5.RNAi}2, source: Helena Richardson | |
Genetic reagent (D. melanogaster) | Mef2-GAL4 | BDSC: 27390 | y[1] w[*]; P{w[+mC]=GAL4-Mef2.R}3 | |
Genetic reagent (D. melanogaster) | UAS-Flp; Mef2-GAL4 | this study | Mef2GAL4 on third combined with UAS-Flp on X | |
Genetic reagent (D. melanogaster) | UAS-GFP-Gma | BDSC: 31776 PMID:11784030 | ||
Genetic reagent (D. melanogaster) | Tub-GAL80-ts | BDSC: 7016 PMID:14657498 | ||
Genetic reagent (D. melanogaster) | hippo[wt] | Duojia Pan, UT Soutwestern, USA PMID:29262338 | tub-FRT-y+-FRT-FLAG-hpo | |
Genetic reagent (D. melanogaster) | hippo[4A/431T] | Duojia Pan, UT Soutwestern, USA PMID:29262338 | tub-FRT-y+-FRT-FLAG-hpo[4A/431T] | |
Genetic reagent (D. melanogaster) | Act88F-GAL4 | Richard Cripps, San Diego State University, USA PMID:22008792 | ||
Genetic reagent (D. melanogaster) | UAS-Hippo | Barry Thompson, John Curtin School of Medical Research, Australia PMID:14502295 | ||
Genetic reagent (D. melanogaster) | UAS-Diap1 | BDSC: 6657; Barry Thompson, John Curtin School of Medical Research, Australia | w[*]; P{w[+mC]=UAS-DIAP1.H}3 | |
Genetic reagent (D. melanogaster) | UAS-p35 | BDSC:5072; Barry Thompson, John Curtin School of Medical Research, Australia | ||
Genetic reagent (D. melanogaster) | yorkie-CA | BDSC: 28836; Barry Thompson, John Curtin School of Medical Research, Australia PMID:18256197 | y[1] w[*]; P{w[+mC]=UAS-yki.S168A.GFP.HA}10-12-1 | |
Genetic reagent (D. melanogaster) | UAS-Yki | Richard G. Fehon, University of Chicago, USA PMID:30032991 | wild type Yorkie tagged with FLAG | |
Genetic reagent (D. melanogaster) | UAS-myr-Yki | Richard G. Fehon, University of Chicago, USA PMID:30032991 | membrane tethered wild type Yorkie tagged with FLAG | |
Genetic reagent (D. melanogaster) | Mhc-GFP | VDRC: 318471 PMID:26896675 | fTRG500 | |
Genetic reagent (D. melanogaster) | Act88F-GFP | VDRC: 318362 PMID:26896675 | fTRG10028 | |
Genetic reagent (D. melanogaster) | Prm-GFP | VDRC: 318114 PMID:26896675 | fTRG475 | |
Genetic reagent (D. melanogaster) | Sls-GFP [CA06744] | Allan Spradling, Carnegie Institution for Science, Maryland, USA PMID:17194782 | ||
Chemical compound, drug | GFP-Booster Atto488 | ChromoTek GmbH gba488 | ||
Chemical compound, drug | Rhodamine Phalloidin | Thermo Fischer Scientific R415 | ||
Chemical compound, drug | Vectashield with DAPI | Biozol VEC-H-1200 | ||
Chemical compound, drug | Fluoroshield with DAPI | Sigma F6057 | ||
Chemical compound, drug | anti-Dlg5, rabbit | this study | ||
Chemical compound, drug | anti-Slmap, rabbit | this study | ||
Chemical compound, drug | anti-Dlg1, mouse | DSHB: 4F3 PMID:11709153 | ||
Chemical compound, drug | anti-Hippo, guinea pig | Georg Halder, VIB-KU Leuven, Belgium PMID:16341207 | ||
Chemical compound, drug | anti-Amphiphysin, rabbit | Jörg Großhans, Georg-August-Universität, Göttingen, Germany PMID:11711432 | ||
Chemical compound, drug | Tissue-Tek O.C.T. | Weckert Labotechnik 4583; Sakura Finetek | ||
Chemical compound, drug | FEATHER Microtome Blades, C35 | pfmmedical, Cologne, Germany 207500003 | ||
Chemical compound, drug | TRIzol | Thermo Fischer Scientific 15596026 |
Fly strains and genetics
Request a detailed protocolFly strains were maintained using standard conditions. Unless otherwise stated, all experiments were performed at 27°C to improve RNAi efficiency. When applying temperature-sensitive Tub-GAL80ts, fly crosses were kept at 18°C to suppress GAL4 activity and the white pre-pupae (0–30 min APF) were shifted to 31°C to allow GAL4 activity only at pupal stages. The pupae were then raised at 31°C until the desired age. Considering that pupae develop faster at 31°C compared to 27°C, timing was corrected by growing pupae at 31°C for 30 hr age matching 32 hr APF at 27°C, for 44 hr matching 48 hr APF and for 84 hr matching 90 hr APF at 27°C. The Act88F-GAL4 UAS-Hippo pupae were raised for 66 hr at 18°C age matching 32 hr APF at 27°C, and for 96 hr at 18°C age matching 48 hr APF at 27°C. RNAi stocks used were from the Vienna (Dietzl et al., 2007) or Harvard collections (Ni et al., 2011) and obtained from VDRC or Bloomington stock centers. All used fly strains are listed in Key Resource Table.
Flight
Request a detailed protocolFlight tests were performed as previously described (Schnorrer et al., 2010). One- to three-day-old male flies were dropped into a 1 m long plexiglass cylinder with 8 cm diameter and five marked sections. Wild-type flies land in the upper two sections, whereas flies with defective flight muscles fall to the bottom of the tube immediately. For each genotype, flight assays were performed two or three times with minimum seven males each (for yorkie-IR females were used) (Supplementary file 1).
Pupal dissection and flight muscle stainings
Request a detailed protocolFor 24 hr, 32 hr, and 48 hr APF pupal dissections, the pupa was stabilised on a slide by sticking to double-sticky tape. The pupal case was removed with fine forceps. Using insect pins two or threeholes were made in the abdomen to allow penetration of the fixative and pupae were fixed in 4% PFA (Paraformaldehyde) in PBST (PBS with 0.3% Triton-X) in black embryo glass dishes for 15 min at room temperature (RT). After one wash with PBST, the pupae were immobilised using insect pins in a silicone dish filled with PBST and dissected similarly as described previously (Weitkunat and Schnorrer, 2014). Using fine scissors, the ventral part of each pupa was removed, then the thorax was cut sagittally and the thorax halves were freed from the abdomen, leaving half thoraces with the flight muscles on them. These half thoraces were transferred to black embryo dishes and blocked for 30 min at room temperature (1/30 normal goat serum in PBST). Primary antibodies (anti-Amph: rabbit, 1/500 (Razzaq et al., 2001); anti-Dlg1: mouse, 1/500, DSHB; anti-Hippo: guinea pig, 1/1000 (Hamaratoglu et al., 2006); anti-Dlg5: rabbit, animal 2125, 1/1000, pre-absorbed; anti-Slmap: rabbit, animal 2151, 1/1000, pre-absorbed) were incubated either at room temperature for 2 hr or overnight at 4°C. Half thoraces were then washed three times 10 min in PBST at room temperature and incubated with secondary antibodies coupled to Alexa dyes (Alexa488 or Alexa568, all from Molecular Probes, 1/500) for 2 hr at room temperature in the dark. To visualise F-actin rhodamine-phalloidin was added to the secondary antibody solution (Molecular Probes, 1/500 in PBST). To visualise GFP fusion proteins, GFP-booster conjugated with Atto488 (ChromoTek, 1/200 in PBST) in combination with rhodamine-phalloidin was used. Half thoraces were incubated 2 hr at room temperature. After three washes with PBST, half thoraces were mounted using Vectashield including DAPI (Biozol).
For comparing protein levels in wild type versus knock-down conditions, pupae from wild type and knock-down genotypes were dissected, stained, and imaged on the same day in parallel under identical settings (master mixes for staining reagents, identical laser and scanner settings for imaging).
For 90 hr APF pupal dissections, the head, wings, legs, and abdomen were cut off the thorax with fine scissors, and the thoraxes were fixed for 20 min in 4% PFA in PBST at RT. After washing once with PBST, the thoraxes were placed on a slide with double-sticky tape and cut sagittally (dorsal to ventral) with a microtome blade (Pfm Medical Feather C35). These half thoraces were stained similarly to the early pupa half thoraces and mounted in Vectashield with DAPI using two spacer coverslips on each side.
Production of polyclonal antibodies against Dlg5 and Slmap
Request a detailed protocolDlg5 cDNA (Gold clone LD32687) corresponding to amino acids 74–379 and Slmap cDNA (Gold clone LD47843) for the full protein sequence were PCR amplified, cloned into pCoofy4 (Scholz et al., 2013), expressed in E. coli to generate His6-MBP fusion proteins; the tags were cleaved and peptides were injected into rabbits using standard protocols (Max Planck Institute of Biochemistry service facilities).
Image acquisition and processing
Request a detailed protocolImage acquisition was performed with Zeiss LSM780-I-NLO, LSM880-I-NLO and LSM880-U-NLO confocal microscopes using Plan Neofluar 10x/0.30 NA air, Plan-Apo 40x/1.4 NA oil and Plan-Apo 63x/1.4 NA oil objectives. For all samples z-stacks were acquired. Image processing was done using Fiji (Schindelin et al., 2012). Digital cross-sections were created from z-stacks that covered the entire width of the flight muscle DLM4 by drawing a straight line in the central part of the fiber and re-slicing. For displaying the thick digital cross-sections, ‘Bleach correction’ plug-in of Fiji was used with Histogram Matching Method. Fiber length and fiber cross-sectional area were measured with freehand drawing tools in Fiji based on phalloidin staining. To determine the average muscle fiber length per hemithorax, the length of all flight muscles for which both fiber ends were visible were measured and averaged.
Myofibril length quantification and intensity profile plots
Request a detailed protocolMyofibrils stained with phalloidin from a 40 µm x 20 µm x 2.5 µm confocal microscope stack were traced semi-automatically using Simple Neurite Tracer plug-in in Fiji (Longair et al., 2011). In each pupa, 10 myofibrils were traced and their average length was calculated. To visualise sarcomere periodicity, intensity profiles were plotted along a line based on actin labelling (phalloidin) using Fiji.
Nuclei count
Request a detailed protocolTo count nuclei numbers of flight muscle fibers, half thoraces were stained with phalloidin (actin) and DAPI (nuclei) and imaged first using 10x objective to quantify the entire length of the fiber and then with 63x oil objective to visualise details. The acquired 63x z-stacks contain the entire muscle depth and about half of the muscle fiber length. Using Fiji’s multi-point tool all the nuclei in each z-stack were counted manually, using actin labelling as a landmark to visualise the borders of the fiber. Nuclei number for the entire fiber was calculated using the length of the entire fiber from the 10x image.
Cryo cross-sections
Request a detailed protocolCryo cross-sections were performed as described previously (Spletter et al., 2018). Briefly, the pupal case was removed by fine forceps. Using insect pins, two or three holes were made in the abdomen to allow penetration of the 4% PFA in PBST (PBS with 0.5% Triton-X) overnight at 4°C. Fixed pupae were sunk in 30% sucrose solution in PBST on a nutator at 4°C. Pupae were embedded in O.C.T. compound in plastic moulds (#4566, Sakura Finetek) and frozen on dry ice. Blocks were sectioned at 20 µm thickness on a microtome cryostat. Sections were adhered on glass slides coated with 1% gelatin, 0.44 mM chromium potassium sulfate dodecahydrate to improve tissue adherence. Sections on the slide were fixed for 1 min in 4% PFA with PBS at room temperature, washed once for 5 min in PBS, incubated with rhodamine-phalloidin (1/500 in PBST) for 2 hr at RT in a wet chamber, washed three times with PBST and mounted in Fluoroshield with DAPI.
Quantifying GFP protein levels
Request a detailed protocolGFP-tagged genomic fosmid fly lines (fTRG500 for Mhc-GFP, fTRG10028 for Act88F-GFP, fTRG475 for Prm-GFP) (Sarov et al., 2016) and GFP-trap fly line Sls-GFP [CA06744] (Buszczak et al., 2007) were used for comparison of protein levels in Mef2-GAL4 driven knockdown of yorkie or gain-of-function yorkie-CA to Mef2-GAL4 control. Pupae of the different genotypes were dissected, stained, and imaged on the same day in parallel under identical settings (master mixes for staining reagents, identical laser and scanner settings for imaging). Per hemi-thorax one or two different areas were imaged using 63x oil objective, zoom factor 2. In Fiji, five z planes at comparable positions in the muscles were selected for an average intensity projection of the volume into a 2D plane. In the 2D plane, two or three regions of 50 µm2 occupied by myofibrils (based on actin labelling) were selected. Mean intensities of each of these regions were averaged to calculate one value per hemi-thorax used for the quantification graphs. For each experimental day, the mean intensity of all wild-type samples was set to one to calculate the relative intensity of the other genotypes. Data from a minimum of two independent experiments were plotted.
RNA-isolation from developing flight muscle
Request a detailed protocolFor each replicate, flight muscles from seven Mef2-GAL4, UAS-GFP-Gma pupae at 24 hr or 32 hr APF were dissected in ice-cold PBS treated with DEPC using a fluorescent binocular. Flight muscles were collected in an Eppendorf tube and centrifuged at 2000 g for 5 min. The flight muscle pellet was re-suspended in TRIzol, shock-frozen in liquid nitrogen and kept at −80°C.
RNA was isolated directly from the TRIzol muscle samples using a 96-well plate extraction kit (Direct-zol−96 RNA, Zymo Research, #R2054): after thawing to room temperature in 1.5 ml Eppendorf tubes, the tissue samples were homogenised using a small pestle, followed by nucleic acid precipitation with 100% ethanol. This suspension was then transferred to the 96-well plate containing the purification columns. DNA digestion was performed ‘in column’ according to the kit instructions. Total RNA was eluted with 25 μl of RNase-free water and was quantified using the Quantifluor RNA System (Promega, #E3310).
BRB-sequencing library preparation
Request a detailed protocolRNA sequencing libraries were prepared using 20 ng of total RNA following the BRB-sequencing protocol (Alpern et al., 2019). Briefly, each RNA sample was reverse transcribed in a 96-well plate using SuperScriptTM II Reverse Transcriptase (Lifetech 18064014) with individual barcoded oligo-dT primers (Microsynth, Switzerland. For primer sequences see Alpern et al., 2019). Next, the samples were split into three pools, purified using the DNA Clean and Concentrator kit (Zymo Research #D4014), and treated with exonuclease I (New England BioLabs, NEB #M0293S). Double-stranded cDNA was generated by the second strand synthesis via the nick translation method. For that, a mix containing 2 μl of RNAse H (NEB, #M0297S), 1 μl of E. coli DNA ligase (NEB, #M0205 L), 5 μl of E. coli DNA Polymerase (NEB, #M0209 L), 1 μl of dNTP (0 .2 mM), 10 μl of 5x Second Strand Buffer (100 mM Tris, pH 6.9, AppliChem, #A3452); 25 mM MgCl2 (Sigma, #M2670); 450 mM KCl (AppliChem, #A2939); 0.8 mM (β-NAD Sigma, N1511); 60 mM (NH4)2SO4 (Fisher Scientific Acros, #AC20587); and 11 μl of water was added to 20 μl of ExoI-treated first-strand reaction on ice. The reaction was incubated at 16°C for 2.5 hr. Full-length double-stranded cDNA was purified with 30 μl (0.6x) of AMPure XP magnetic beads (Beckman Coulter, #A63881) and eluted in 20 μl of water.
The Illumina compatible libraries were prepared by tagmentation of 5 ng of full-length double-stranded cDNA with 1 µl of in-house produced Tn5 enzyme (11 μM). After tagmentation the libraries were purified with DNA Clean and Concentrator kit (Zymo Research #D4014), eluted in 20 µl of water and PCR amplified using 25 μl NEB Next High-Fidelity 2x PCR Master Mix (NEB, #M0541 L), 2.5 μl of P5_BRB primer (5 μM, Microsynth), and 2.5 μl of Illumina index adapter (Idx7N5 5 μM, IDT) following program: incubation 72°C—3 min, denaturation 98°C—30 s; 15 cycles: 98°C—10 s, 63°C—30 s, 72°C—30 s; final elongation at 72°C—5 min. The fragments ranging 200–1000 bp were size-selected using AMPure beads (Beckman Coulter, #A63881) (first round 0.5x beads, second 0.7x). The libraries were profiled with High Sensitivity NGS Fragment Analysis Kit (Advanced Analytical, #DNF-474) and measured with Qubit dsDNA HS Assay Kit (Invitrogen, #Q32851) prior to pooling and sequencing using the Illumina NextSeq 500 platform using a custom primer and the High Output v2 kit (75 cycles) (Illumina, #FC-404–2005). The library loading concentration was 2.2 pM and sequencing configuration as following: R1 6 c / index 8 c / R2 78 c.
Pre-processing of the data—de-multiplexing and alignment
Request a detailed protocolThe sample reads de-multiplexing was done using BRB-seqTools (http://github.com/DeplanckeLab/BRB-seqTools) as described before (Alpern et al., 2019). The sequencing reads were aligned to the Ensembl gene annotation of the Drosophila melanogaster BDGP6.23 genome using STAR (version 020201) (Dobin et al., 2013), and count matrices were generated with HTSeq (version 0.9.1) (Love et al., 2014).
Bioinformatics analysis of BRB-Seq data
Request a detailed protocolBRB-seq data quality was assessed in several ways. First, we excluded five samples from further analysis due to low numbers of aligned reads (<500K; removed were 1 × 24 hr APF wild type, 1x Dlg5-IR 24 hr, 1x yorkie-CA 24 hr and 2 × 32 hr APF wild type). Using raw read counts, we performed PCA analysis, calculated heatmaps and Pearson’s correlation in R (Version 3.3.1, https://cran.r-project.org/). One additional replicate from 32 hr APF wild type was removed from further analysis as it represented a clear outlier. For the remaining samples, we performed library normalisation (RLE) as well as differential expression analysis using DESeq2 (version 1.22.2, Love et al., 2014). Genes were considered differentially expressed with a log2FC ≥ |1| and an FDR ≤ 0.05. Functional enrichment analysis was performed on the differentially expressed genes using FlyEnrichr (Kuleshov et al., 2019). Data are available at the Gene Expression Omnibus database (Clough and Barrett, 2016), accession number GSE158957.
Affinity enrichment mass spectrometry
Request a detailed protocolFor each replicate, about 100 pupae staged from 24 hr - 48 hr APF of the genotypes Mef2-GAL4 (control) or Mef2-GAL4, UAS-Dlg5-GFP were collected and processed as described previously (Sarov et al., 2016). For each genotype, four replicates (100 pupae each) were snap-frozen in liquid nitrogen and ground to powder while frozen. The powder was processed as described (Hubner et al., 2010). Briefly, the cleared lysate was mixed with magnetic beads pre-coupled to a GFP antibody matrix to perform single step affinity enrichment and mass-spec analysis using an Orbitrap mass spectrometer (Thermo Fisher) and the QUBIC protocol (Hein et al., 2015). Raw data were analysed in MaxQuant version 1.4.3.22 (Cox and Mann, 2008) using the MaxLFQ algorithm for label-free quantification (Cox et al., 2014). The volcano plot was generated with Graphpad.
Data availability
Sequencing data have been deposited in GEO under accession code GSE158957.
-
NCBI Gene Expression OmnibusID GSE158957. The Hippo pathway controls myofibril assembly and muscle fiber growth by regulating sarcomeric gene expression.
References
-
Cells with persistent twist expression are the embryonic precursors of adult muscles in DrosophilaDevelopment 113:79–89.
-
The carnegie protein trap library: a versatile tool for DrosophilaDevelopmental Studies 175:1505–1531.https://doi.org/10.1534/genetics.106.065961
-
SRF and TEF-1 control of chicken skeletal alpha-actin gene during slow-muscle hypertrophyAmerican Journal of Physiology-Cell Physiology 270:C1624–C1633.https://doi.org/10.1152/ajpcell.1996.270.6.C1624
-
The gene expression omnibus databaseMethods in Molecular Biology 1418:93–110.https://doi.org/10.1007/978-1-4939-3578-9_5
-
Accurate proteome-wide label-free quantification by delayed normalization and maximal peptide ratio extraction, termed MaxLFQMolecular & Cellular Proteomics 13:2513–2526.https://doi.org/10.1074/mcp.M113.031591
-
Yes-associated protein isoform 1 (Yap1) Promotes cardiomyocyte survival and growth to protect against myocardial ischemic injuryJournal of Biological Chemistry 288:3977–3988.https://doi.org/10.1074/jbc.M112.436311
-
STAR: ultrafast universal RNA-seq alignerBioinformatics 29:15–21.https://doi.org/10.1093/bioinformatics/bts635
-
Mob family proteins: regulatory partners in hippo and Hippo-Like intracellular signaling pathwaysFrontiers in Cell and Developmental Biology 8:161.https://doi.org/10.3389/fcell.2020.00161
-
The sarcomere and sarcomerogenesisAdvances in Experimental Medicine and Biology 642:1–14.https://doi.org/10.1007/978-0-387-84847-1_1
-
M-CAT binding factor is related to the SV40 enhancer binding factor, TEF-1Journal of Biological Chemistry 267:17234–17240.https://doi.org/10.1016/S0021-9258(18)41917-5
-
Development of the indirect flight muscles of DrosophilaDevelopment 113:67–77.
-
The spectrin cytoskeleton regulates the hippo signalling pathwayThe EMBO Journal 34:940–954.https://doi.org/10.15252/embj.201489642
-
Mechanical strain regulates the hippo pathway in DrosophilaDevelopment 145:dev159467.https://doi.org/10.1242/dev.159467
-
The sarcomere and the nucleus: functional links to hypertrophy, atrophy and SarcopeniaAdvances in Experimental Medicine and Biology 642:176–191.https://doi.org/10.1007/978-0-387-84847-1_13
-
The Salvador-Warts-Hippo pathway - an emerging tumour-suppressor networkNature Reviews Cancer 7:182–191.https://doi.org/10.1038/nrc2070
-
Quantitative proteomics combined with BAC TransgeneOmics reveals in vivo protein interactionsJournal of Cell Biology 189:739–754.https://doi.org/10.1083/jcb.200911091
-
STRIPAK complexes: structure, biological function, and involvement in human diseasesThe International Journal of Biochemistry & Cell Biology 47:118–148.https://doi.org/10.1016/j.biocel.2013.11.021
-
Mechanisms of myoblast fusion during muscle developmentCurrent Opinion in Genetics & Development 32:162–170.https://doi.org/10.1016/j.gde.2015.03.006
-
Enrichr: a comprehensive gene set enrichment analysis web server 2016 updateNucleic Acids Research 44:W90–W97.https://doi.org/10.1093/nar/gkw377
-
modEnrichr: a suite of gene set enrichment analysis tools for model organismsNucleic Acids Research 47:W183–W190.https://doi.org/10.1093/nar/gkz347
-
Mechanical forces during muscle developmentMechanisms of Development 144:92–101.https://doi.org/10.1016/j.mod.2016.11.003
-
In vivo regulation of yorkie phosphorylation and localizationDevelopment 135:1081–1088.https://doi.org/10.1242/dev.015255
-
Sallimus and the dynamics of sarcomere assembly in Drosophila flight musclesJournal of Molecular Biology 427:2151–2158.https://doi.org/10.1016/j.jmb.2015.04.003
-
Myosin isoform switching during assembly of the Drosophila flight muscle thick filament latticeJournal of Cell Science 126:139–148.https://doi.org/10.1242/jcs.110361
-
The hippo signaling pathway in development and CancerDevelopmental Cell 19:491–505.https://doi.org/10.1016/j.devcel.2010.09.011
-
The Drosophila RASSF homolog antagonizes the hippo pathwayCurrent Biology 16:2459–2465.https://doi.org/10.1016/j.cub.2006.10.060
-
Ultrastructure of developing flight muscle in Drosophila. I. assembly of myofibrilsDevelopmental Biology 160:443–465.https://doi.org/10.1006/dbio.1993.1320
-
BookAssembly and maintenance of myofibrils in striated muscleIn: James B. E, editors. Handbook of Experimental Pharmacology. Springer. pp. 39–75.
-
Mechanisms regulating skeletal muscle growth and atrophyFEBS Journal 280:4294–4314.https://doi.org/10.1111/febs.12253
-
Muscle fiber type diversity revealed by anti-myosin heavy chain antibodiesThe FEBS Journal 285:3688–3694.https://doi.org/10.1111/febs.14502
-
Fiji: an open-source platform for biological-image analysisNature Methods 9:676–682.https://doi.org/10.1038/nmeth.2019
-
The Frank-Starling mechanism in vertebrate cardiac myocytesJournal of Experimental Biology 211:2005–2013.https://doi.org/10.1242/jeb.003145
-
The initial steps of myofibril assembly: integrins pave the wayNature Reviews Molecular Cell Biology 10:293–298.https://doi.org/10.1038/nrm2634
-
Hippo promotes proliferation arrest and apoptosis in the Salvador/Warts pathwayNature Cell Biology 5:914–920.https://doi.org/10.1038/ncb1050
-
Hippo pathway regulation by cell morphology and stress fibersDevelopment 138:3907–3914.https://doi.org/10.1242/dev.070987
-
The hippo pathway effector YAP is a critical regulator of skeletal muscle fibre sizeNature Communications 6:ncomms7048.https://doi.org/10.1038/ncomms7048
-
Translational repressor Bruno plays multiple roles in development and is widely conservedGenes & Development 11:2510–2521.https://doi.org/10.1101/gad.11.19.2510
-
The unified myofibrillar matrix for force generation in muscleNature Communications 11:6.https://doi.org/10.1038/s41467-020-17579-6
-
YAP and TAZ: a signalling hub of the tumour microenvironmentNature Reviews Cancer 19:454–464.https://doi.org/10.1038/s41568-019-0168-y
Article and author information
Author details
Funding
European Research Council (FP/2007-2013)
- Frank Schnorrer
Centre National de la Recherche Scientifique
- Frank Schnorrer
Aix-Marseille Université (ANR-11-IDEX-0001-02)
- Frank Schnorrer
Agence Nationale de la Recherche (ANR-ACHN MUSCLE-FORCES)
- Frank Schnorrer
Agence Nationale de la Recherche (ANR-18-CE45-0016-01)
- Bianca H Habermann
Human Frontier Science Program (RGP0052/2018)
- Frank Schnorrer
Agence Nationale de la Recherche (ANR-10-INBS-04-01)
- Frank Schnorrer
Humboldt Foundation
- Aynur Kaya-Çopur
EMBO
- Aynur Kaya-Çopur
Fondation Bettencourt Schueller
- Frank Schnorrer
Turing Center for Living Systems
- Frank Schnorrer
Max Planck Society
- Frank Schnorrer
The funders had no role in study design, data collection and interpretation, or the decision to submit the work for publication.
Acknowledgements
The authors are indebted to Reinhard Fässler for hosting part of this work in his department, and to Jürg Müller and Anne-Kathrin Classen for hosting AK-Ç during parts of this study. We are grateful to the IBDM and LIC (Albert-Ludwigs University of Freiburg) imaging facilities for help with image acquisition and maintenance of the microscopes and the Max Planck service facilities for protein expression and antibody production. We acknowledge the France-BioImaging infrastructure supported by the French National Research Agency (ANR–10–INBS-04–01, Investments for the future). Fly stocks obtained from the Bloomington Drosophila Stock Center (NIH P40OD018537) and the Vienna Drosophila Resource Center (VDRC) were used in this study. The authors thank Barry Thompson, Richard Fehon, Jörg Großhans, Georg Halder and Duojia Pan for fly stocks and antibodies, as well as Pierre Mangeol and Clara Sidor for helpful discussions and critical comments for this manuscript.
Copyright
© 2021, Kaya-Çopur et al.
This article is distributed under the terms of the Creative Commons Attribution License, which permits unrestricted use and redistribution provided that the original author and source are credited.
Metrics
-
- 3,351
- views
-
- 504
- downloads
-
- 43
- citations
Views, downloads and citations are aggregated across all versions of this paper published by eLife.
Citations by DOI
-
- 43
- citations for umbrella DOI https://doi.org/10.7554/eLife.63726