Short and long sleeping mutants reveal links between sleep and macroautophagy
Abstract
Sleep is a conserved and essential behavior, but its mechanistic and functional underpinnings remain poorly defined. Through unbiased genetic screening in Drosophila, we discovered a novel short-sleep mutant we named argus. Positional cloning and subsequent complementation, CRISPR/Cas9 knock-out, and RNAi studies identified Argus as a transmembrane protein that acts in adult peptidergic neurons to regulate sleep. argus mutants accumulate undigested Atg8a(+) autophagosomes, and genetic manipulations impeding autophagosome formation suppress argus sleep phenotypes, indicating that autophagosome accumulation drives argus short-sleep. Conversely, a blue cheese neurodegenerative mutant that impairs autophagosome formation was identified independently as a gain-of-sleep mutant, and targeted RNAi screens identified additional genes involved in autophagosome formation whose knockdown increases sleep. Finally, autophagosomes normally accumulate during the daytime and nighttime sleep deprivation extends this accumulation into the following morning, while daytime gaboxadol feeding promotes sleep and reduces autophagosome accumulation at nightfall. In sum, our results paradoxically demonstrate that wakefulness increases and sleep decreases autophagosome levels under unperturbed conditions, yet strong and sustained upregulation of autophagosomes decreases sleep, whereas strong and sustained downregulation of autophagosomes increases sleep. The complex relationship between sleep and autophagy suggested by our findings may have implications for pathological states including chronic sleep disorders and neurodegeneration, as well as for integration of sleep need with other homeostats, such as under conditions of starvation.
Introduction
Sleep is a widespread behavior across animals with nervous systems and occupies a significant proportion of human life. The importance of sleep is evident in the consequences of its disruption, which range from impaired cognitive performance to serious health problems, and even death in some animal models (Mignot, 2008). However, we still have limited understanding of the mechanisms that regulate sleep or the physiological functions served by it.
The fruit fly has been essential to identifying molecular mechanisms regulating sleep. Forward genetic screens in Drosophila melanogaster revealed several molecular sleep regulators and effectors later implicated in mammalian sleep. The first was the voltage-gated potassium channel Shaker; its mammalian homolog (Kcna2) was later shown to have corresponding effects in mice (Cirelli et al., 2005; Douglas et al., 2007). Similarly, we previously identified redeye, a nicotinic acetylcholine receptor (nAchR) alpha subunit gene required for sleep maintenance, although cholinergic signaling is typically thought of as wake-promoting, sleep-promoting cholinergic neurons that act through a related nicotinic receptor subunit were subsequently identified in mammals (Ni et al., 2016; Shi et al., 2014). The power of invertebrate behavioral screening is perhaps best demonstrated by a tour de force sleep mutant screen recently conducted in mice; homologs of the two sleep-regulating genes identified, Nalcn sodium leak channel and Sik3 kinase, were previously linked to sleep in Drosophila and Caenorhabditis elegans, respectively (Flourakis et al., 2015; Funato et al., 2016; van der Linden et al., 2008). Sleep genes originally identified in flies are increasingly also implicated in human sleep. Both voltage-gated potassium channels and nicotinic acetylcholine receptors were top hits in a genome-wide association study for polymorphisms associated with human sleep duration (Allebrandt et al., 2013). And autoantibodies to voltage-gated potassium channels have been found in people with Morvan’s syndrome, a neurological disorder associated with insomnia (Barber et al., 2000).
The fruit fly has already also proven valuable for interrogating functions of sleep. Proposed functions for sleep across organisms include memory consolidation, both synaptic and metabolic homeostasis, and waste clearance from the brain (Mignot, 2008). Sleep promotes memory consolidation in Drosophila (Dag et al., 2019; Donlea et al., 2011), which is also reflected in the deep intertwinement of sleep and memory circuitry (Haynes et al., 2015; Joiner et al., 2006; Pitman et al., 2006; Sakai et al., 2012). Meanwhile clearance effects implicated in mammals are inferred from sleep regulation of endocytosis across the Drosophila blood-brain barrier (Artiushin et al., 2018; Mestre et al., 2020). Crucially, whether the varying functions of sleep represent independent outputs of a sleeping brain, or are linked in some manner, is unknown.
A large proportion of waste clearance in cells is mediated by macroautophagy (hereafter, autophagy), which recycles bulk material including protein aggregates and damaged organelles. Different types of autophagy can be induced by stimuli including accumulation of ubiquitinated protein, unfolded protein response, pro-apoptotic signaling, and metabolic stressors including amino acid starvation (Hale et al., 2013). Factors involved vary by stimulus, but they converge on a core network of essential proteins that mediate nucleation, expansion, and maturation of an Atg8(+) autophagosome; loading of cargo; and ultimately lysosomal fusion, forming an autolysosome whose acidification and protease activity degrades the cargo (Hale et al., 2013). Successful autophagy both remediates toxicity of its cargo and liberates metabolites for reuse by the cell. Autophagy crosstalk with other clearance mechanisms suggest it as a potentially important transducer or effector of sleep. Yet to our knowledge, no direct link between sleep and autophagy has been established.
Here, we report a novel short-sleeping mutant, argus (aus), derived from a screen of chemically mutagenized flies. The gene responsible for the mutant phenotype encodes an integral membrane protein whose loss in neurons, including in peptidergic subpopulations, reduces sleep by increasing accumulation of undigested autophagosomes. Genetic manipulations that block autophagy upstream of Atg8 recruitment to autophagosomes suppress the aus reduced sleep phenotype. Further, in the cases of an independently identified sleep mutant, blue cheese-58, and RNAis for several autophagy genes, prominently including atg1 and atg8a/b, blockade of autophagosome production increases baseline sleep. Finally, we show that autophagosomes accumulate during the day, in a manner that can be acutely extended by sleep deprivation or curtailed by enforced sleep. Together, our data suggest that sleep regulates autophagy in a daily sleep:wake cycle, and sustained and/or strong changes in autophagosome level affects sleep amount. This model suggests that autophagy is a promising candidate for coupling sleep to its known functions in the healthy and neurodegenerative brain.
Results
argus mutants have reduced sleep
As reported previously, we mutagenized newly isogenized iso31 flies with ethyl methane sulfonate (EMS), generated independent lines, and screened F3 generation flies under 12:12 light:dark cycles for sleep phenotypes (Shi et al., 2014). One line that reproducibly showed reduced sleep was named argus (aus: after the mythological Greek giant who never slept) and subjected to further analysis. aus homozygotes showed ~600 fewer minutes of total sleep per 24 hr day compared to iso31 controls (Ryder et al., 2004) controls (p < 0.0001; Figure 1A–B). aus sleep decrease was primarily driven by inability to sustain sleep, as reflected in a significant decrease in aus homozygote sleep bout duration during both day and night (p < 0.0001; Figure 1C), while sleep bout number was unchanged during the day and increased at night (p < 0.05; Figure 1D). Sleep latency analysis showed that aus mutants took a longer time to initiate sleep after lights off at ZT12 than aus heterozygotes or controls (p < 0.01; Figure 1E). Activity index, locomotor activity per waking minute, was comparable between aus mutants and controls (p > 0.05; Figure 1F), indicating that aus is not a hyperactive mutant. aus heterozygotes showed a small decrease in sleep relative to controls (p < 0.001; Figure 1A–B), indicating that the aus mutation is slightly dominant.
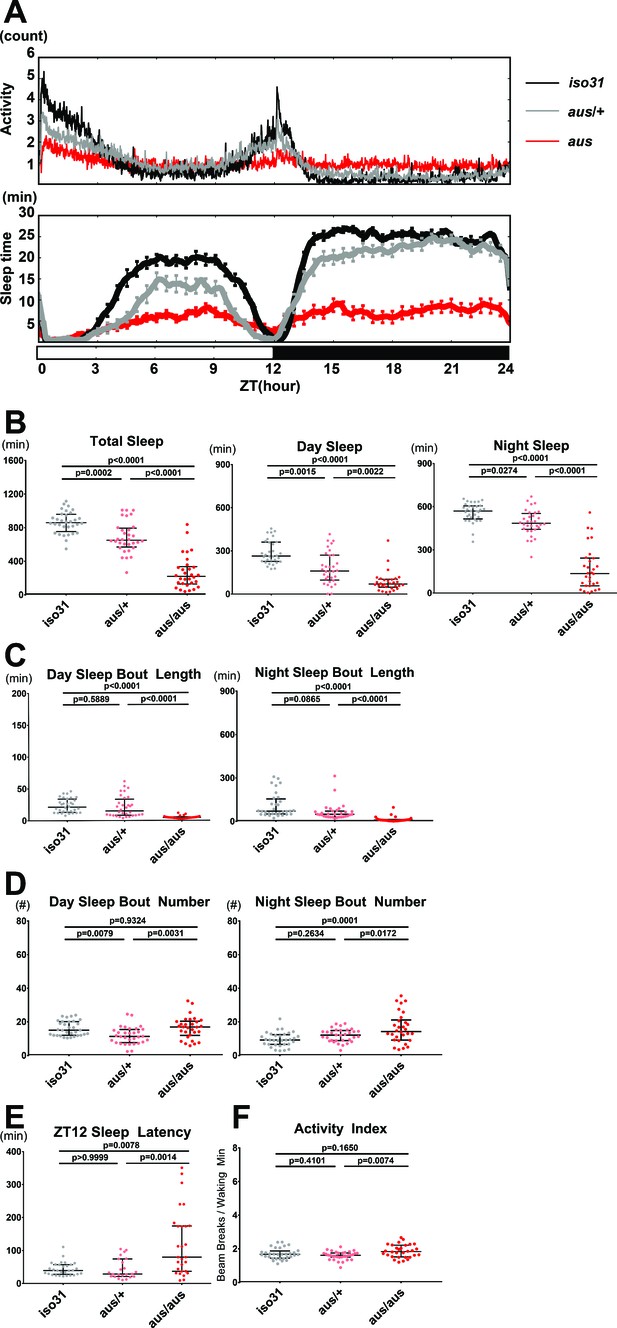
Sleep phenotype of argus mutants.
All sleep metrics were measured under a 12 hr:12 hr light:dark cycle in female iso31 (gray), aus/+ (pink) and aus/aus (red) flies. (A) Mean activity (top panel) and sleep (bottom panel) over time during the 24-hr cycle. (B) Total sleep amount during the whole 24-hr cycle (left), day (middle), and night (right). (C) Mean sleep bout duration during the day (left) and night (right). (D) Sleep bout number during the day (left) and night (right). (E) Latency to first sleep bout after ZT12 lights off. (F) Activity index of beam breaks per waking minute over the 24-hr cycle. n = 30–32 (A–D,F) or n = 23–30 (E); individual flies overlaid with median±interquartiles (B–F); Tukey test (B-total+ night,C,F) or Dunn test (B-day,D-E). (A).
-
Figure 1—source data 1
Sleep Phenotype of Argus Mutants.
- https://cdn.elifesciences.org/articles/64140/elife-64140-fig1-data1-v1.xlsx
As the circadian clock regulates sleep timing, and some clock mutants show changes in total sleep (Shaw et al., 2002), we tested aus behavior under constant darkness for a potential circadian phenotype. Most aus homozygotes ( > 60%) showed robust locomotor activity rhythms, indicating an intact circadian clock (Figure 1—figure supplement 1A). Similarly to other short-sleeping mutants, the overt arrhythmia in ~30% of aus homozygotes likely stems from the large reduction in sleep (Figure 1—figure supplement 1B; Shi et al., 2014). Notably, aus homozygotes displayed longer activity episodes than controls in constant darkness (Figure 1—figure supplement 1A), consistent with their short sleep under LD conditions.
Identification of CG16791 as a candidate gene for argus
As the original screen selected for recessive mutations on the third chromosome (Shi et al., 2014), mapping of the aus mutation was initiated by crossing the mutants with a line carrying multiple genetic markers on the third chromosome (ru1 h1 Diap11 st1 cu1 sr1 es ca1). Recombinant progeny were screened for sleep phenotypes and subjected to classical mapping, localizing aus distal to ebony. We then developed single nucleotide polymorphism (SNP) markers through genomic DNA sequencing of aus mutants and the genetic marker line; the aus mutation was localized between SNP markers at ~19 and 24 Mb (Figure 2A). In parallel, we subjected genomic DNAs from aus homozygotes and iso31 controls to whole-genome sequencing. DNA sequences were aligned with the Drosophila Genome Project for SNP calling. While >500,000 polymorphic sites distinguished our stocks from the reference sequence, many SNPs were common to aus and the iso31 control; these were removed from further consideration (Figure 2B). In the ~5 Mb region identified by mapping, we found 622 aus-specific SNPs, of which 10 led to amino acid changes in nine open-reading frames (ORFs) (Figure 2B).
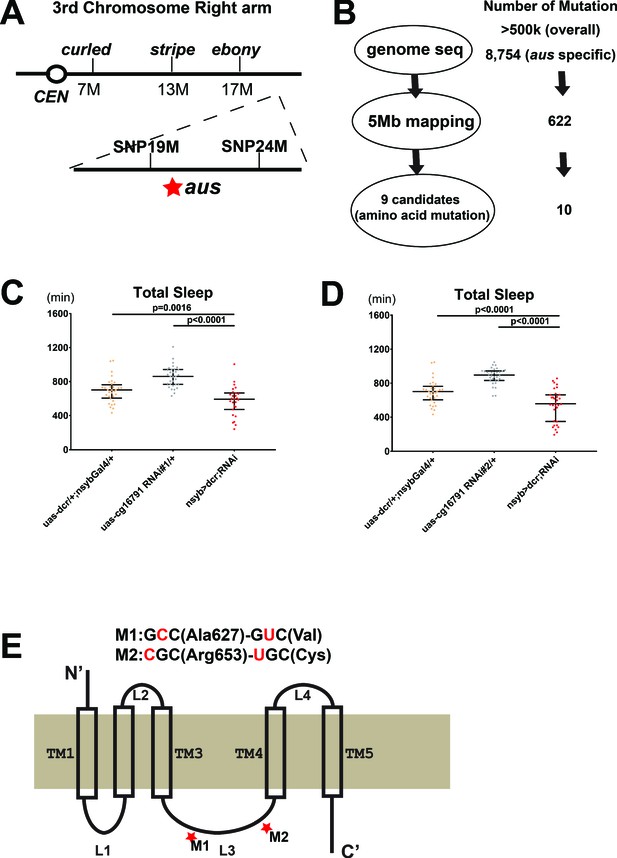
Mapping the argus sleep phenotype to a single gene: cg16791.
(A) The genomic location of argus is indicated as a star within a 5 Mb region on the right arm of the third chromosome, following genetic mapping with visible mutations and SNP markers. (B) Schematic of the genome sequencing procedure of argus homozygotes with the number of mutations identified in each step listed on the right. The initial alignment revealed more than half a million mutations relative to the published Drosophila genome. More than eight thousand mutations remained after removing mutations also found in the iso31 control strain. Factoring in the mapping data (shown in A) and focusing on missense mutations narrowed the number of candidate genes to nine. (C–D) Total sleep with cg16791 RNAi knockdown in females, using pan-neuronal driver nsyb-Gal4, uas-dicer, and either of two independent RNAi lines, compared to RNAi-alone and nsyb-Gal4+ Dcr alone controls. n = 27–32; Fischer’s LSD; individual flies overlaid with median±interquartiles. (E) Predicted protein of CG16791. Two GC-AT transitions that cause missense mutations in the loop3 region were identified by Sanger-sequencing in aus mutants.
-
Figure 2—source data 1
Mapping the argus sleep phenotype to a single gene: cg16791.
- https://cdn.elifesciences.org/articles/64140/elife-64140-fig2-data1-v1.xlsx
We focused on these ORFs, knocking each down in a pan-neuronal RNAi screen using elav-GAL4 driver, and identifying two candidates that produced sleep loss. One was Neurexin 1 (nrx1, cg7050), a synapse assembly molecule that regulates fruit fly sleep (Tong et al., 2016). We ruled this candidate out, as the nrx1 knockout showed no loss of sleep amount compared to control in our hands (Figure 2—figure supplement 1A) and it complemented aus sleep loss in transheterozygotes (Figure 2—figure supplement 1B).
The other candidate gene was cg16791, in which aus mutant flies have two GC→AT transitions that are predicted to translate to A→V and R→C amino acid substitutions. Supporting its identity as the aus locus, pan-neuronal knockdown of cg16791 with elav-Gal4> Dicer and cg16791 RNAi#1 produced a severe sleep reduction comparable to aus/aus mutants (p < 0.0001; Figure 2—figure supplement 2A,B). This also suggested the aus sleep phenotype is neural in origin. To rule out RNAi off-target effects and confirm this neuron-specificity, we assessed sleep behavior in cg16791 RNAi #1 and #2 crossed to Nsyb-Gal4> Dicer2 flies. Both pan-neuronal knockdown manipulations resulted in significant decreases in total sleep compared to RNAi and Nsyb-Gal4> Dicer2 controls (p < 0.01; Figure 2C–D). These results confirm neuron-dependence of the cg16791 RNAi sleep phenotype.
Our studies to this point did not address whether cg16791 acutely regulates adult sleep, or the development of sleep regulatory mechanisms. To address this, we crossed cg16791 RNAi#1 and #2 to Actin-GeneSwitch (GS)> Dicer2, and conducted sleep experiments in the presence of food supplemented with either the gene switch activating drug mifepristone / RU486 (RU+) or ethanol vehicle control (RU-). Actin-GS> Dicer2+ RNAi#2 flies showed a robust decrease in sleep compared to RNAi and Actin-GS> Dicer2 controls on RU+; however, there was no difference between genotypes on RU- (p < 0.0001; Figure 2—figure supplement 2C). Actin-GS> Dicer2+ RNAi#2 flies also showed a within-genotype reduction of sleep on RU+ vs RU- (p < 0.001; Figure 2—figure supplement 2C), while the control genotypes did not. This shows that cg16791 regulates sleep in adulthood. RNAi#1 caused a weak trend toward RU-dependent sleep loss that did not reach significance when crossed with Actin-GS> Dicer2, likely because of weaker knockdown (data not shown); note also that RNAi#2 is predicted to have higher specificity (Figure 2—figure supplement 2D).
Having putatively identified the aus locus, we took a bioinformatic approach to hypothesize probable structure and function of the largely uncharacterized CG16791 protein isoforms. An unbiased ProDom search of the full-length CG16791 isoform-A reference sequence identified a number of possible transmembrane motifs (Supplementary file 2). A more targeted TMPred assessment of known CG16791 isoforms predicted their best-fit membrane topology with a 5-transmembrane structure, and placed the aus mutations in an internal loop region between transmembrane helices 3 and 4 (Figure 2D; Supplementary file 2). This same loop contains a variable region that distinguishes the four known isoforms of CG16791. A Deep-Loc-1.0 analysis predicted that all CG16791 isoforms are targeted to the cell membrane, and perhaps to some extent the ER/Golgi network, driven predominantly by signal sequences in the C-terminus (Supplementary file 2; Almagro Armenteros et al., 2017). Our bioinformatic analyses are experimentally supported by the isolation of CG16791 isoform-A from membrane fractions of fly head (Aradska et al., 2015). Based on these analyses, we speculated that the aus substitutions in CG16791’s internal loop cause a loss-of-function that underlies its sleep loss phenotype.
Mutations in CG16791 underlie the argus sleep phenotype
To confirm that mutated CG16791 leads to the aus sleep phenotype, we performed additional mutant analysis, as well as rescue assays. First, we obtained a P-element insertion allele of cg16791 that breaks the open reading frame of the gene (hereafter, P1). The P1 allele failed to complement the aus mutant. Thus, P1/aus trans-heterozygotes had severely reduced total sleep comparable to aus homozygotes (p < 0.01; Figure 3A), supporting the idea that the cg16791 mutations in aus are causal for the sleep phenotype.
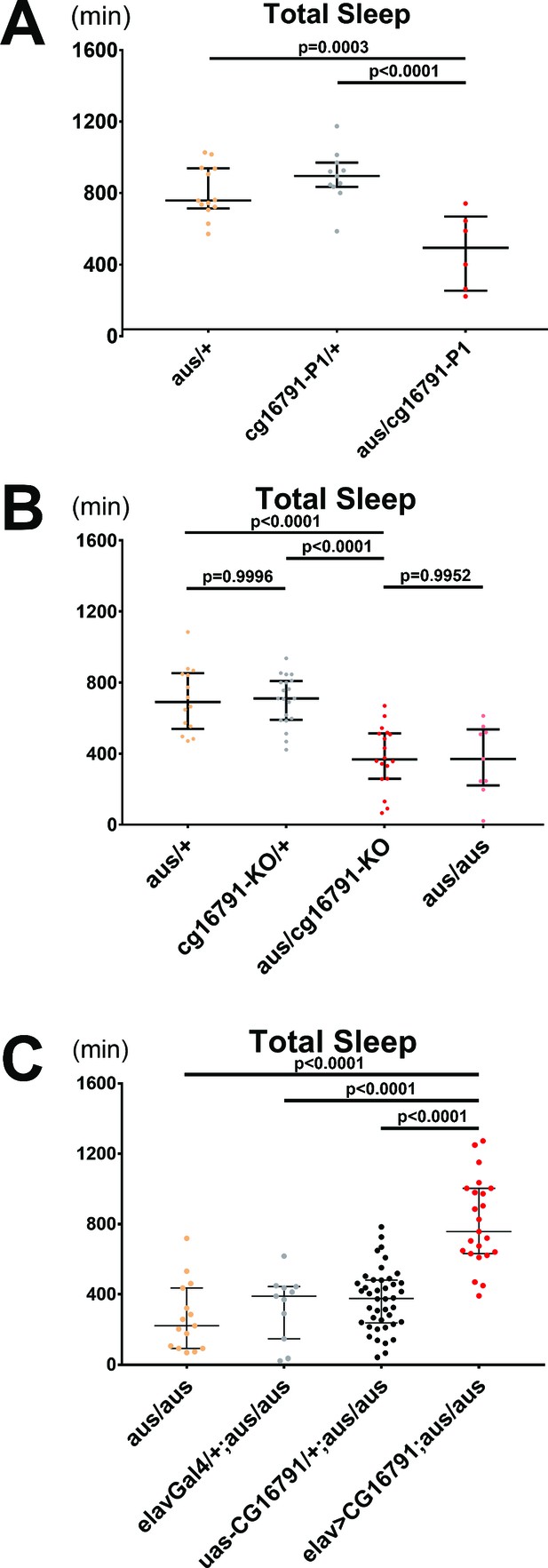
CG16791 underlies the argus sleep phenotype.
(A) Transheterozygotes of male aus and cg16791 insertional mutant (P1) have reduced total sleep compared to aus/+ and cg16791-P1/+ controls. n = 6–13; individual flies overlaid with median±interquartiles; Fischer’s LSD. (B) Female cg16791-KO and aus (EMS) transheterozygotes have reduced total sleep compared to aus (EMS) or cg16791-KO heterozygotes. Transheterozygote total sleep is comparable to aus homozygotes. n = 9–20; individual flies overlaid with median±interquartiles; Tukey test. (C) Pan-neuronal expression of uas-cg16791 with elav-Gal4 partially rescues female aus homozygote short-sleep, to significantly above aus-homozygous Gal4 and UAS controls. n = 11–42; individual flies overlaid with median±interquartiles; Fischer’s LSD.
-
Figure 3—source data 1
CG16791 underlies the argus sleep phenotype.
- https://cdn.elifesciences.org/articles/64140/elife-64140-fig3-data1-v1.xlsx
We then used CRISPR/Cas9 to generate a cg16791 knockout, in which the first exon containing the initiating methionine was replaced with a selectable marker, Dsred (Gratz et al., 2013; Figure 3—figure supplement 1A). As homozygous knockouts were semi-lethal (0.59% survival rate; 2 / 339 flies tested), we could only reliably obtain cg16791KO heterozygotes. Southern blot analysis confirmed a single integration of DsRed at the aus locus (Figure 3—figure supplement 1B). Behavioral analysis showed that total sleep in cg16791KO heterozygotes is comparable to aus heterozygotes, while trans-heterozygotes of cg16791KO and aus showed a severe reduction in total sleep, similar to aus homozygotes (p < 0.0001; Figure 3B, Figure 3—figure supplement 1C). These cg16791KO results further support our mapping of the aus EMS allele to loss-of-function of CG16791. However, the aus EMS allele maintains function required for survival, as EMS homozygotes are viable while knockout homozygotes are semi-lethal.
The gold standard to confirm that a specific mutation drives a mutant phenotype is through a rescue experiment. We cloned two cDNA forms of cg16791 under control of a UAS (Upstream Activation Sequence): a UAS-cg16791 short form beginning with the second methionine, which lacks 51 amino acids at the N-terminus, and a full-length UAS-cg16791FL form. Pan-neuronal (elav-Gal4) induction of either form effectively rescued sleep in aus mutants (p < 0.0001; Figure 3C, Figure 3—figure supplement 1D), proving that mutations in cg16791 indeed cause aus sleep loss. This result also suggests that the CG16791FL N-terminus is dispensable for its sleep function. For simplicity, only UAS-cg16791 was used for later experiments.
Argus functions in dimmed-positive peptidergic neurons to regulate sleep
We next sought to identify the brain region through which aus regulates sleep. We first cloned ~2 kb of the aus promoter upstream of Gal4 and used the resulting fly line, ausP2k-Gal4, to drive expression of membrane-bound GFP. GFP was expressed broadly in the fly brain, in many cell types including peptidergic neurons of the pars intercerebralis (PI), Kenyon cells of the mushroom body, optic lobe neurons and some lateral neurons (Figure 4A). Importantly, P2k-driven aus expression rescued aus/aus short sleep, indicating that P2k-Gal4 recapitulates the sleep-relevant aus expression pattern (p < 0.05; Figure 4A).
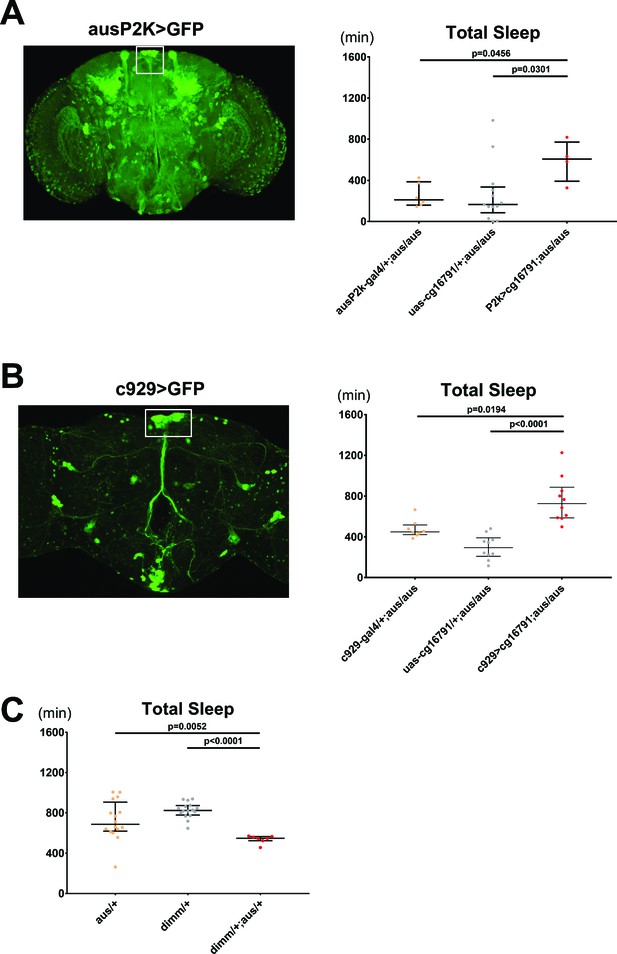
Argus functions in dimmed positive neurons to regulate sleep.
(A) The aus promoter region was subcloned, and a ~ 2000 bp sequence was inserted upstream of Gal4 and used to drive GFP (left). aus2kGal4 driving uas-cg16791 partially rescues the short sleep phenotype in female fruit flies (ausP2K, UAS-cg16791, or ausP2K > UAS-cg16791 in aus/aus mutant background). n = 4–13; individual flies overlaid with median±interquartiles; Fischer’s LSD. (B) C929-Gal4 (a peptidergic Gal4 line representing Dimmed expression) driving GFP (left). C929 driving uas-cg16791 expression rescues the short sleep phenotype in male aus flies. (c929, UAS-cg16791, or c929> UAS-cg16791 in aus/aus mutant background). n = 8–10; individual flies overlaid with median±interquartiles; uncorrected Dunn’s test. (C) aus and dimm interact genetically in female transheterozygotes to reduce sleep (aus/+, dimm/+, and aus dim transheterozygotes). n = 7–16; individual flies overlaid with median±interquartiles; uncorrected Dunn’s test.
-
Figure 4—source data 1
Argus Functions in Dimmed Positive Neurons to Regulate Sleep.
- https://cdn.elifesciences.org/articles/64140/elife-64140-fig4-data1-v1.xlsx
Based on the prominent representation of neuropeptidergic populations labeled by the Aus2k driver, we suspected that aus functions in peptidergic pathways to regulate sleep/arousal behavior. To test this, we assayed for rescue using the peptidergic neuron specific c929-Gal4 driver, which is inserted near the dimmed gene, a bHLH transcription factor essential for neuroendocrine cell differentiation, and which appears to express in overlapping cell populations with Aus2k-Gal4 (Figure 4A–B, white boxes) (Hewes, 2003). c929-Gal4-driven aus expression in an aus mutant homozygous background partially rescued the short sleep phenotype (p < 0.05; Figure 4B), demonstrating that aus expression in peptidergic cells contributes to sleep behavior. Furthermore, transheterozygotes for dimmed (which have impaired neuropeptidergic neuron function, including in the PI) (Hewes, 2003) and aus show a synergistic loss of sleep compared to the respective single heterozygotes, suggesting that loss of neuropeptide signaling contributes to the aus sleep phenotype (p < 0.01; Figure 4C).
Aus mutants show an accumulation of autophagosomes
In investigating the mechanism by which aus regulates sleep, we noted that CG16791 was previously identified as a protein that interacts with the cell engulfment receptor Draper (Fullard and Baker, 2015). Draper is involved in cell death associated with autophagy, the primary disposal pathway for large-scale cellular waste such as protein aggregates and damaged organelles, and an emergency nutrient source (McPhee et al., 2010). Thus, we considered the possibility that AUS plays a role in waste disposal, such as autophagy, within cells. To determine if autophagy is regulated by AUS, we conducted live-imaging experiments in aus mutants and iso31 control flies pan-neuronally by expressing a GFP-mcherry-atg8a fusion protein driven by elav-Gal4 (Figure 5A). mCherry red fluorescence, but not GFP green fluorescence, persists under low pH; thus, autophagosomes retain both GFP and mCherry fluorescence, while acidified autolysosomes (autophagosomes that have fused with lysosomes to degrade their cargoes) selectively quench GFP, leaving only mCherry fluorescence (Mauvezin et al., 2014).
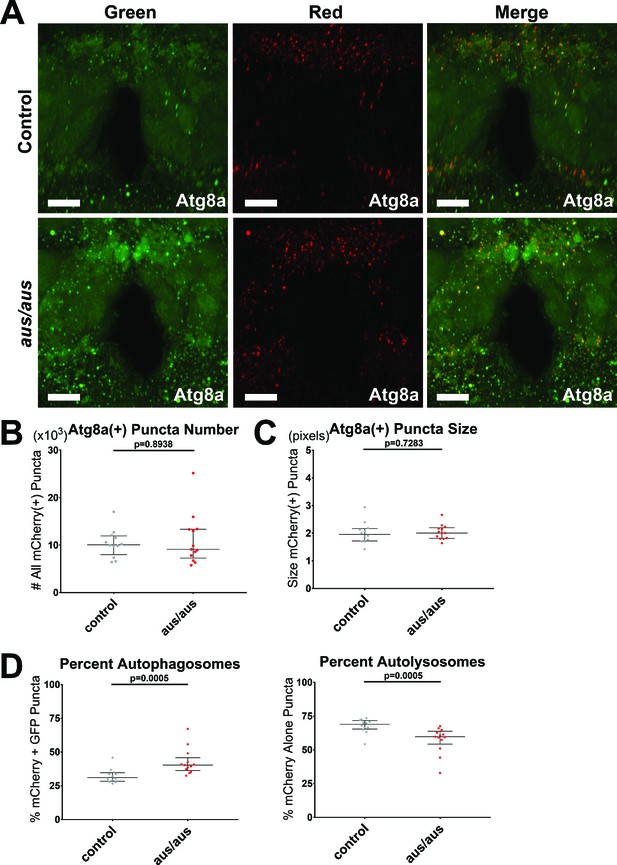
The argus mutant displays accumulation of autophagosomes.
Female iso31 control and aus/aus brains with elav-Gal4> UAS-GFP-mCherry-Atg8a driving pan-neuronal autophagy sensor were live imaged from ZT0-2. mCherry fluoresces in all Atg8a(+) puncta, while GFP fluoresces in autophagosomes and is quenched in autolysosomes. (A) Max-projected z-stacks of representative brains showing GFP (left), mCherry (middle), and merged (right) fluorescence. Scale bar = 25 um. (B) The number of all neuronal mCherry(+) puncta was similar in both genotypes. (C) The size of all neuronal mCherry(+) puncta was similar in both genotypes. (D) aus neuronal mCherry(+) puncta were significantly skewed toward % mCherry+ GFP(+) autophagosomes (left) and away from % mCherry-only(+) autolysosomes (right) compared to control. n = 12–13; individual brains overlaid with median±interquartiles; Mann-Whitney tests.
-
Figure 5—source data 1
The argus mutant displays accumulation of autophagosomes.
- https://cdn.elifesciences.org/articles/64140/elife-64140-fig5-data1-v1.xlsx
First, we validated a machine learning protocol for identifying neuronal Atg8(+) puncta by comparing autophagy flux in a small cohort of elav-Gal4> UAS-GFP-mCherry-atg8a brains dissected from ZT0-2 and incubated in either 2 uM rapamycin or ethanol vehicle in AHL for 2 hr prior to imaging. As expected, given its well-characterized role as a TOR inhibitor and inducer of starvation-dependent autophagy, rapamycin pre-treatment increased total mCherry(+) puncta compared to vehicle control, with no significant difference in either the size of these puncta or the ratio of mCherry+ GFP autophagosomes to mCherry-only autolysosomes (Figure 8—figure supplement 1A–D).
We then tested aus/aus flies pan-neuronally expressing the same sensor. Neither the number nor the size of all mCherry(+) puncta was significantly different in aus mutants compared to controls (p > 0.05; Figure 5B–C), but the distribution was significantly skewed toward double-labeled puncta with a reduction in the number of mCherry-alone puncta, suggesting that inefficient lysosomal digestion drives autophagosome accumulation in aus mutants (p < 0.001; Figure 5D). It is surprising that overall mCherry(+) puncta number is not increased by aus blockade of autophagosome degradation; the most likely explanation is a compensatory reduction of autophagy initiation upstream.
Autophagosome accumulation regulates sleep in aus and blue cheese mutants
Through an independent project targeted toward identifying links between sleep and neurodegeneration, we discovered a sleep phenotype in the blue cheese 58 loss-of-function allele (bchs). The bchs mutant is best known for an autophagy defect that decreases the accumulation of autophagosomes and drives neurodegeneration (Finley et al., 2003; Simonsen et al., 2007). We found that bchs increases sleep compared to iso31 control (p < 0.01), primarily by lengthening night sleep bouts (p < 0.001) (Figure 6A, and Figure 6—figure supplement 1A–C). bchs also decreases latency to sleep at nightfall (p < 0.0001), suggesting that the sleep gain reflects increased sleep need (Figure 6—figure supplement 1D). Activity index was unaffected by either dosage of bchs, ruling out defective locomotion as a confound of the sleep gain phenotype (p > 0.05) (Figure 6—figure supplement 1E). Many bchs sleep phenotypes were recessive in females and dominant in males, suggesting sexual dimorphism.
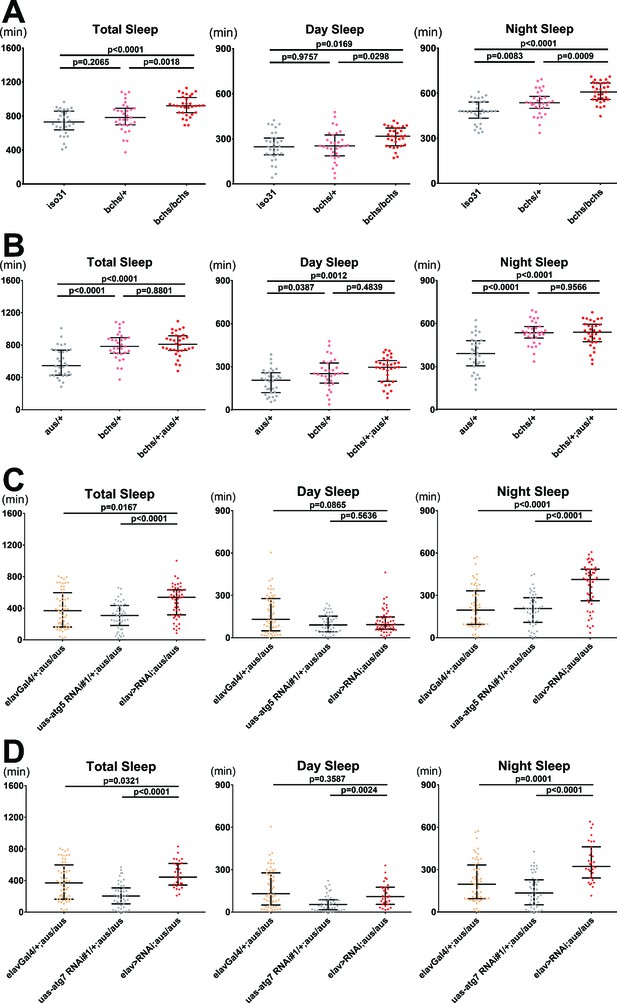
Blocking autophagosome production rescues the short sleep phenotype of the argus mutant.
(A) Total, day, and night sleep were measured under 12 hr:12 hr light:dark in iso31 control, bchs/+, and bchs/bchs female flies. n = 31–32; individual flies overlaid with median±interquartiles; Tukey tests. (B) Total, day, and night sleep were measured under 12 hr:12 hr light:dark in aus/+, bchs/+, and bchs/+; aus/+ transheterozygous female flies. n = 31–32; individual flies overlaid with median±interquartiles; Tukey tests. (C–D) Total, day, and night sleep were measured under 12 hr:12 hr light:dark in elav-Gal4/+, UAS-RNAi/+ and elav-Gal4> UAS RNAi female flies in aus/aus mutant background. RNAi’s used were atg5 RNAi#1 (C) and atg7 RNAi#1 (D). n = 46–54 (C) or n = 31–54 (D); individual flies overlaid with median±interquartiles; uncorrected Dunn’s tests.
-
Figure 6—source data 1
Blocking autophagosome production rescues the short sleep phenotype of the argus mutant.
- https://cdn.elifesciences.org/articles/64140/elife-64140-fig6-data1-v1.xlsx
Overall autophagy is impaired in both aus and bchs mutants, and yet they have opposing effects on sleep. However, we noticed that while the aus mutant increases the accumulation of autophagosomes, likely by blocking their clearance (Figure 5), the bchs mutant has been shown to decrease the accumulation of autophagosomes, by blocking the maturation of immature Atg5(+) autophagosomes and recruitment of Atg8 (Sim et al., 2019). We hypothesized that the opposite changes in the level of Atg8(+) autophagosomes in aus and bchs mutants drive their respective sleep phenotypes. If true, the bchs sleep phenotype should be epistatic to that of aus, since the bchs blockade of autophagosome maturation is expected to be upstream of aus autophagosome accumulation.
To test this, we generated transheterozygous bchs/+; aus/+ female flies and tested their sleep behavior compared to each single heterozygote. While a single allele of bchs had no effect on total sleep amount compared to control iso31 (Figure 6A), it robustly and non-additively suppressed sleep phenotypes of aus, rendering the bchs/+; aus/+ transheterozygotes statistically indistinguishable from bchs/+ for total, day, and night sleep amount, all of which were higher than aus/+ (p < 0.05; Figure 6B). Activity index was significantly higher in transheterozygotes and bchs/+ flies compared to aus/+ flies, suggesting an improvement in aus mobility with the addition of bchs that does not confound bchs suppression of aus short-sleep (Figure 6—figure supplement 1F). Our findings suggest that the bchs sleep phenotype is indeed epistatic to that of aus, consistent with their respective effects on the autophagy pathway. This epistatic relationship could not be assessed in trans-homozygous bchs/bchs; aus/aus flies because of a lethal interaction.
To confirm that aus short-sleep suppression by bchs is not due to Bchs roles in other cellular pathways including lysosome trafficking (Lim and Kraut, 2009), we assessed whether neuron-specific impairment of autophagosome maturation could rescue short-sleep in aus heterozygotes and homozygotes. Thus, we generated homozygous aus flies with elav-Gal4-driven pan-neuronal RNAi knockdown of atg5 or atg7, both of which are involved in autophagosome maturation and Atg8 recruitment/activation (Hale et al., 2013). Both pan-neuronal atg RNAis increased sleep on an aus mutant background (p < 0.05), driven selectively by increases in night sleep (Figure 6C–D). Importantly, neither RNAi increased sleep in flies lacking the aus mutation—in fact, atg7 RNAi decreased sleep in control flies—indicating that the rescue of aus did not result from an additive interaction (p < 0.001; Figure 6—figure supplement 2A,B). Impaired locomotion cannot explain either sleep rescue phenotype on the aus/aus background, as pan-neuronal atg5 RNAi flies had similar activity index to controls, while the activity index of pan-neuronal atg7 RNAi flies was intermediate between its controls (p < 0.05; Figure 6—figure supplement 2C,D). Much like the bchs mutant, atg5 and atg7 RNAi also rescue the milder sleep defect of aus/+ flies (p < 0.001; Figure 6—figure supplement 2E,F).
Blocking autophagosome formation in adulthood increases sleep in wild-type Drosophila
While the rescue of aus by neuronal knockdown of atg5 and atg7 RNAi implicated impaired autophagosome clearance as a mechanism underlying the short-sleep phenotype, we asked why these neuronal knockdowns did not produce a phenotype on their own. The bchs sleep gain could be driven by its roles in pathways aside from autophagy, so to rigorously test whether autophagy affects sleep, we conducted a targeted RNAi screen of genes with known links to various steps of autophagy for sleep behavior (Supplementary file 3, Tab 1). The use of drug-inducible geneswitch drivers allowed us to restrict manipulations to adulthood.
We first screened with pan-neuronal nsyb-geneswitch+ uas-dicer on RU+ food, and identified five RNAis for four genes that significantly increased sleep (p < 0.05; Figure 7A). These included upstream regulators that couple autophagy to starvation (Atg1, 2 RNAi’s), unfolded protein response (Bip), and ecdysone signaling (Daor) (Figure 7—figure supplement 1A,B,D,E; Hale et al., 2013). The remaining gain-of-sleep hit was Atg10, an E2 ligase-like enzyme involved in autophagosome vesicle expansion (Figure 7—figure supplement 1C; Hale et al., 2013).
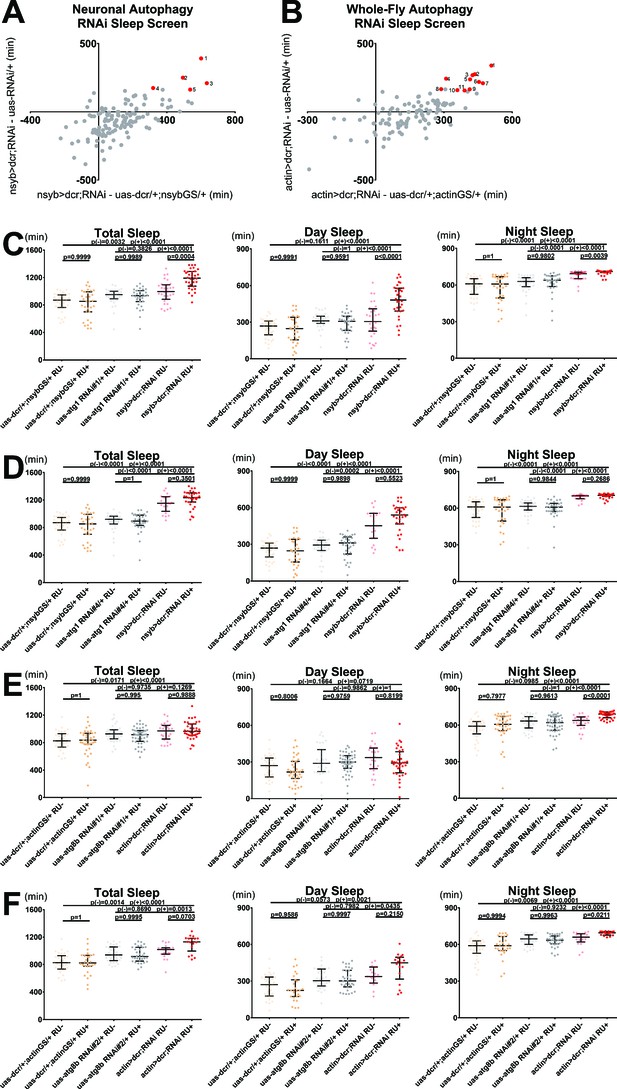
Blocking neuronal or whole-fly autophagosome formation increases sleep.
(A) Difference in first-pass population median sleep on RU+ food for a range of female nsybGS> dcr;autophagy-RNAi crosses compared with nsybGS> dcr control (x-axis) and RNAi control (y-axis). Red, numbered dots indicate significant hits that passed all validation steps: (1) bip RNAi#3; (2) atg1 RNAi#4; (3) daor RNAi#1; (4) atg1 RNAi#1; (5) atg10 RNAi#3. N = 133 viable crosses shown; n = 3–16 flies per group for each first-pass experiment. (B) Difference in first-pass population median sleep on RU+ food for a range of female actinGS> dcr;autophagy-RNAi crosses compared with actinGS> dcr control (x-axis) and RNAi control (y-axis). Red, numbered dots indicate significant hits that passed all validation steps: (1) dor RNAi#2; (2) atf6 RNAi#1; (3) atg8b RNAi#2; (4) wacky RNAi#2; (5) atg8b RNAi#1; (6) atg7 RNAi#1; (7) daor RNAi#1; (8) atg14 RNAi#3; (9) dram RNAi#2; (10) aduk RNAi#3; (11) atg12 RNAi#2. N = 106 viable crosses shown; n = 3–16 flies per group for each first-pass experiment. See Supplementary file 3 for details on first-pass screen and Figure 7—figure supplement 1 for combined first/second pass sleep data for significant hits, for the screens shown in both 7A and 7B. (C–F) Total (left), day (middle), and night (right) sleep in GS> dcr;RNAi, GS> dcr control, and RNAi control female flies on both RU+ and RU- food. All data shown as individual flies overlaid with median±interquartiles; p(-) indicates RU- p-values and p(+) indicates RU+ p-values. (C) nsybGS> dcr;atg1-RNAi#1: n = 31–32; Steel-Dwass test (total,night) and Tukey test. (day). (D) nsybGS> dcr;atg1-RNAi#4: n = 21–32; Steel-Dwass tests. (E) actinGS> dcr;atg8b-RNAi#1: n = 25–47; Steel-Dwass test (total,night) and Tukey test. (day). (F) actinGS> dcr;atg8b-RNAi#2: n = 17–32; Steel-Dwass tests.
-
Figure 7—source data 1
Blocking Neuronal or Whole-Fly Autophagosome Formation Increases Sleep.
- https://cdn.elifesciences.org/articles/64140/elife-64140-fig7-data1-v1.xlsx
To accomplish broad and strong knockdown of autophagy genes, we repeated the same screen with actin-geneswitch. As expected, this approach yielded more gain-of-sleep hits (P < 0.05; Figure 7B), including several autophagosome maturation proteins: two distinct RNAis encoding Atg8b (one of two ubiquitin-like homologs that label mature autophagosomes), and single RNAis for Atg12 and Atg7 (Figure 7—figure supplement 1H,I,J,K; Hale et al., 2013). Notably, the atg7 hit was the same allele used to rescue aus, suggesting that sleep loss from its knockdown with elav-Gal4 in the absence of aus reflects dosage and/or developmental compensation effects (Figure 6 and Figure 6—figure supplement 1B). Additional RNAi hits encoded proteins involved in autophagy initiation by multiple pathways (Aduk, Atf6, Daor, Dram, Wacky); autophagosome nucleation (Atg14, Dor); and facilitating autophagosome-autolysosome fusion (also Dor) (Figure 7—figure supplement 1F,G,L,M,N,O,P; Hale et al., 2013; Lindmo et al., 2006; Montagne, 2016). All of these hits consistently increased sleep, in the case of Dor likely because of an epistatic effect on autophagosome nucleation (Lindmo et al., 2006).
To validate these results, we back-crossed our highest confidence hits (atg1 RNAi’s #1,4 and atg8b RNAi’s #1,2) to iso31 and closely assessed sleep with crosses to nsybGS and actinGS (respectively). Both atg1 RNAi crosses increased total sleep (p < 0.0001; Figure 7C–D). The nsybGS> dcr,atg1 RNAi#1 increased sleep largely RU-dependently, while nsybGS> dcr,atg1 RNAi#4 increased sleep largely RU-independently, suggesting a leaky GS/RNAi combination (Figure 7C–D). Neither total mean bout length nor total bout number was significantly increased in either cross (Figure 7—figure supplement 2A,B,G,H). But both atg1 RNAi crosses had many flies with massive single night-time sleep bouts, and on RU+ food we found consistently longer night (p < 0.01) and longest (p < 0.0001) sleep bout lengths, with significantly lower night bout number (p < 0.05), suggesting that consolidation of night sleep drives overall sleep gain in these flies (Figure 7—figure supplement 2A,B,C,G,H,I). Sleep latency at nightfall was consistently decreased in both atg1 RNAi crosses on both foods (p < 0.01), with an even stronger decrease on RU+ vs RU- (p < 0.001; Figure 7—figure supplement 2D,J). Food-independent increased waking activity in atg1 knockdowns excludes the possibility that sleep increases are derived from sickness (p < 0.05; Figure 7—figure supplement 2E,K). Finally, qPCR quantification confirmed knockdown of atg1 by our RNAi alleles in actinGS> dcr,atg1 RNAi flies (p < 0.05; Figure 7—figure supplement 2F,L).
Both actinGS> dcr,atg8b RNAis robustly and RU-dependently increased night sleep, but only RNAi#2 increased total sleep and day sleep after back-crossing (Figure 7E–F). Both atg8b RNAis RU-dependently increased mean sleep bout length (p < 0.05), driven disproportionately by longest bout (p < 0.05; Figure 7—figure supplement 2M-O,S-U). Sleep latency at nightfall was marginally decreased on RU+ food compared to RU- in actinGS> dcr,atg8b RNAi flies, but not control genotypes (p < 0.05; Figure 7—figure supplement 2P,V). Neither atg8b RNAi cross had significantly different waking activity compared to both controls on either RU+ or RU- food (Figure 7—figure supplement 2Q,W). As qPCR did not consistently detect atg8b even in control fly extracts, suggesting very low expression, atg8b RNAis may effect their sleep gain by knockdown of atg8a through conserved sequences. This was supported by qPCR quantification of atg8a cDNA in actinGS> dcr,atg8b RNAi flies (p < 0.05; Figure 7—figure supplement 2R,X).
In sum, pan-neuronal atg1 and whole-fly atg8 knockdown phenotypes largely recapitulate the key hallmarks of bchs phenotypes: (1) sleep gain disproportionately driven by night sleep, (2) sleep consolidation, and (3) decreased sleep latency at nightfall (Figure 6 and Figure 6—figure supplement 1). This supports our attribution of the bchs sleep phenotype to its autophagy effects and, more generally, the sleep promoting effects of blocking autophagosome formation. RU-dependence of many phenotypes demonstrates that perturbing autophagosome formation in adulthood is sufficient to drive changes in sleep.
Sleep negatively regulates autophagosome formation in Drosophila
Our findings above indicated that autophagy, in particular autophagosome levels, regulate sleep amount. To determine whether sleep, in turn, regulates autophagosome accumulation, we first live-imaged neuronal autophagy flux in brains of flies carrying elav-Gal4> UAS-GFP-mCherry-atg8a at ZT0-2 and ZT12-14 (Figure 8A). In the early night, there were more total mCherry(+) puncta than in the early day, with no significant difference in the size of mCherry(+) puncta or the ratio of mCherry+ GFP autophagosomes to mCherry-only autolysosomes, suggesting a potential role for sleep:wake state in regulating the production of autophagosomes (p < 0.05; Figure 8B–D). However, this experiment left ambiguous whether sleep contributed to the observed day/night effect. To address this, we mechanically sleep-deprived (SD) flies of the same genotype overnight for at least 12 hr, and compared autophagy flux in SD vs control flies at ZT0-2 (Figure 8E). SD flies had significantly more total mCherry(+) puncta compared to controls, with no significant difference in the size of mCherry(+) puncta or the ratio of mCherry+ GFP autophagosomes to mCherry-only autolysosomes (Figure 8F–H).
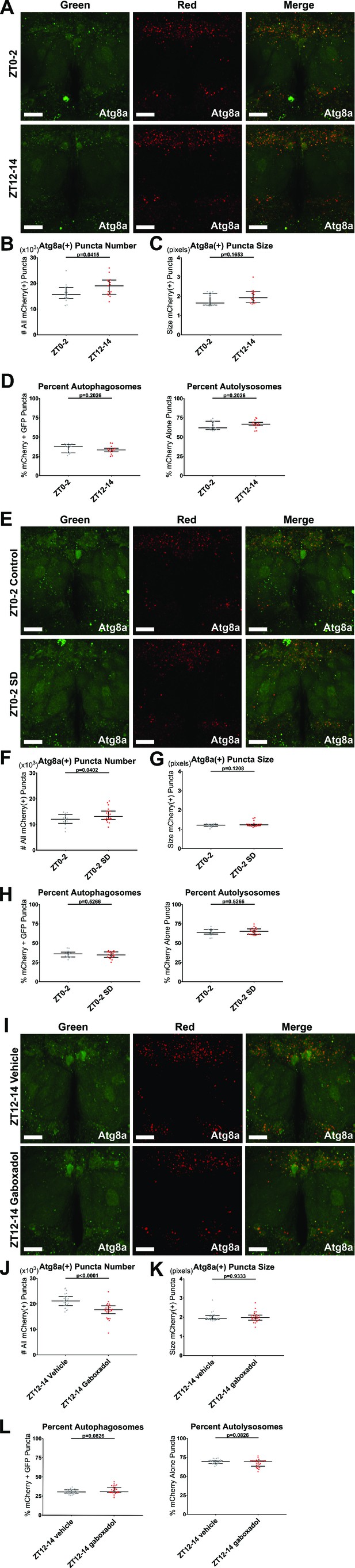
Sleep regulates autophagosome production.
elav-Gal4> UAS-GFP-mCherry-Atg8a flies expressing pan-neuronal autophagy sensor were live imaged as follows. All quantification shows individual brain values overlaid with population median±interquartiles. (A–D) ZT0-2 or ZT12-14. n = 15; Student’s t-tests. (E–H) ZT0-2 after either a control night of unchallenged sleep or at least 12 hr of mechanical sleep deprivation (SD) beginning at the prior ZT12. n = 13–20; Student’s t-tests. (I–L) ZT12-14 after either a control day of feeding with vehicle or at least 11 hr of feeding with 0.1 mg/mL gaboxadol that verifiably and markedly increased daytime sleep, beginning at the prior ZT0-1. n = 25–26. (A,E,I) Max-projected z-stacks of representative brains showing GFP (left), mCherry (middle), and merged (right) fluorescence for ZT time comparison (A), control vs SD (E), or vehicle vs gaboxadol (I). Scale bars = 25 µm. (B,F,J) The number of all neuronal mCherry(+) puncta was higher at nightfall than daybreak (B), elevated at daybreak by 12 hr overnight SD (F), and depressed at nightfall by 12 hr daytime of gaboxadol-induced sleep (J). (C,G,K) The size of all neuronal mCherry(+) puncta was unaffected by ZT time, SD, and gaboxadol. (D,H,L) The percentage of neuronal mCherry(+) puncta that are mCherry+ GFP(+) autophagosomes (left) and mCherry-only(+) autolysosomes (right) was unaffected by ZT time, SD, and gaboxadol.
-
Figure 8—source data 1
Sleep regulates autophagosome production.
- https://cdn.elifesciences.org/articles/64140/elife-64140-fig8-data1-v1.xlsx
To complement our SD data and mitigate possible confounding effects from the stress of mechanical perturbation, we also assayed effects of increased sleep on autophagy. We flipped flies onto food laced with either gaboxadol or water vehicle at ZT0-1, and compared ZT1-12 sleep and ZT12-14 autophagy flux in the same flies (Figure 8I). As previously reported, gaboxadol treatment markedly increased sleep (Figure 8—figure supplement 1E; Berry et al., 2015). Gaboxadol flies had significantly fewer total mCherry(+) puncta compared to controls, with no significant difference in either the size of mCherry(+) puncta or the ratio of mCherry+ GFP autophagosomes to mCherry-only autolysosomes (Figure 8J–L).
These data showing that wake increases and sleep decreases autophagosome number in wild-type fly neurons (Figure 8) were unexpected because they could be interpreted as sleep-promotion by autophagosomes, while our mutant and RNAi data indicate that high neuronal autophagosome number decreases sleep and low neuronal autophagosome number promotes sleep (Figures 1 and 5—7). As discussed below, we believe that the phenotypes of the mutants/RNAis reflect sustained high or low levels of autophagosomes not seen during a normal daily cycle (Figure 9).
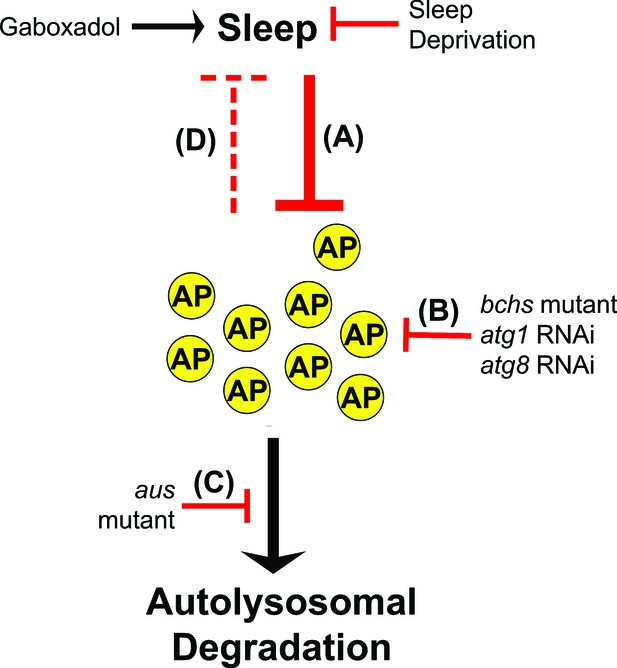
Model for sleep-autophagy interaction.
This schematic details our model for how sleep and macroautophagy interact, based on our results. (A) Sleep decreases autophagosome number under normal conditions, in a manner that is sensitive to both gaboxadol gain or SD loss of sleep lasting between 11 and 14 hr. (B) The mutant blue cheese, pan-neuronal RNAi for atg1, and whole-fly RNAi for atg8b (suppressing both 8a and 8b homologs) are all known to inhibit autophagosome formation, and all increase sleep. (C) Neuronal loss-of-function in the aus mutant inhibits autophagosome degradation, and decreases sleep in a manner that is rescued by blocking autophagosome formation upstream. (D) The wake-promoting / sleep-inhibiting effects of autophagosome number are able to drive sleep behavior when strongly and sustainably adjusted by our genetic manipulations, but are unable to drive sustained waking after a single night of SD, as acute rebound sleep is well established to occur after sleep deprivation on this timescale. Together, this suggests that autophagosome inhibition of sleep is considerably weaker than sleep inhibition of autophagosome accumulation, with autophagosome number only becoming a strong enough signal to control sleep behavioral output with a very strong and/or sustained stimulus.
Discussion
Using a forward genetic screen, we identified a novel neural regulator of both sleep and autophagosomal clearance: argus (cg16791) (Figures 1—3 and 5). Autophagosomes accumulate in aus mutants, likely due to impaired lysosomal clearance, and multiple genetic manipulations that disrupt whole-fly or neuronal autophagosome formation rescue aus mutant sleep (Figures 5 and 6). A link between sleep and autophagy is further supported by our finding of additional sleep phenotypes upon downregulation of components of autophagic pathways (Figures 6 and 7).
Drosophila is a powerful model for the use of unbiased approaches to identify the molecular basis of a physiological process of interest. Indeed, the molecular basis of the circadian clock was determined largely through forward genetic screens of the type used to isolate aus (Dubowy and Sehgal, 2017). We and others have employed a similar forward genetics toolkit to discover sleep-regulating genes (Dubowy and Sehgal, 2017). These genes have provided insight into mechanisms that control daily sleep amount, but to date, they have not suggested functions of sleep. For the most part, the genes identified encode neuromodulators or regulators of neural excitability, which are likely required to modulate brain activity in response to homeostatic sleep need (Cirelli et al., 2005; Koh et al., 2008; Shi et al., 2014). The generation of sleep need is presumably linked to sleep function, but the nature of this remains elusive. The aus sleep mutant is unique, in that the mechanisms underlying its loss of sleep are likely relevant for sleep function (discussed below).
Our finding that aus regulates autophagy is consistent with its expression pattern spatially, temporally, and even intracellularly. aus is temporally elevated during the embryo cellularization and late larval / early pupal stages of fruit fly development, times of enhanced developmental autophagy. Indeed, high autophagy during the latter stage provided the first observation of the pathway in Drosophila melanogaster (Kuhn et al., 2015; Thurmond et al., 2019; Gaudecker, 1963). aus expression is also spatially enriched in tissues with high levels of developmental and adult autophagy, including brain, gut, and fat body (Thurmond et al., 2019). Finally, this hypothesis is consistent with our bioinformatic predictions, as the cell membrane, endoplasmic reticulum, and Golgi apparatus cellular compartments are all proposed donors of autophagosome membranes (Supplementary file 2; Nishimura and Tooze, 2020). We propose that Aus is required for transition of autophagosomes to autolysosomes, and so in its absence, autophagosomes accumulate.
To better understand the effect of autophagy disruptions on sleep, we exploited the vast existing mutant and RNAi resources available in Drosophila to conduct directed screening. This demonstrated sleep gain in multiple scenarios that impede the production of autophagosomes, including homozygotes for bchs58 (Figure 6A), a loss-of-function mutant known to impair autophagosome maturation (Sim et al., 2019), and RNAi knockdown of a number of genes involved in autophagy initiation, autophagosome nucleation, and autophagosome maturation, in particular atg1 and atg8a/b (Figure 7 and Figure 7—figure supplement 1, Figure 7—figure supplement 2). Using a subset of these tools that were too weak to drive sleep gain in wild-type flies, we find complete suppression of aus/+ sleep loss by bchs/+, and rescue of aus/+ and aus/aus sleep loss by pan-neuronal RNAi for either atg5 or atg7 (Figure 6B–D). While the individual genes each have roles in additional pathways, the simplest explanation of consistent aus sleep rescue by three distinct autophagy gene loss-of-functions is that the observed autophagosome accumulation in aus mutants is a contributor to their short-sleeping phenotype (Figures 5 and 6). Together, our findings of abnormal sleep in both the aus and bchs autophagy mutants, as well as knockdown effects of functionally related clusters of canonical autophagy genes, demonstrate that strongly and sustainably disrupting autophagy in adulthood perturbs sleep, such that high autophagosome levels decrease sleep, while low autophagosome levels increase sleep (Figure 9B–D).
We then set out to determine whether this relationship reflects changes normally seen over the sleep-wake cycle. We found that Atg8a(+) autophagosomes accumulate during the waking hours and decrease during sleep, with autophagosome levels at daybreak increased by SD the preceding night, and autophagosome levels at nightfall decreased by gaboxadol-induced sleep the prior day (Figure 8). This demonstrated that at least one of two possibilities must be true in the absence of perturbations of autophagy: (i) sleep increases clearance of autophagosomes, and/or (ii) sleep decreases production of autophagosomes (Figure 8). Given the lack of effect on autophagosome/autolysosome ratio in our daybreak/nightfall, SD, and gaboxadol experiments, our data are most consistent with sleep reducing autophagosome production (Figure 9A). An attractive etiological explanation for this observation is elevated metabolic activity during wake generating waste that could enhance the production of autophagosomes, leading to autophagosome accumulation that is then run down over extended sleep. That said, we cannot fully rule out autophagosome clearance changes that occur as a gradual or late-onset feature of sleep. Sleep enhancement of the degradation of debris and damaged cells (Singh and Donlea, 2020) and the flushing of degraded wastes from the brain (Artiushin et al., 2018; Xie et al., 2013) seem to hint at a role for sleep in autophagosome clearance, and we believe that this possibility deserves further study. Finally, our findings in wild-type brains likely generalize to mammals, as various endosome-autophagosome-lysosome axis puncta accumulate in mice under chronic sleep fragmentation (Xie et al., 2020).
Regardless of whether autophagosome production, clearance, or both are affected during the normal sleep-wake cycle, our imaging data clearly show that waking increases and sleeping decreases autophagosome number (Figure 8). This is surprising given our mutant and RNAi data demonstrating that autophagosome level inhibits sleep (Figures 5—7). The most parsimonious synthesis of these results is a model in which sleep inhibits autophagosome formation on a 24 hr timescale (Figure 9A), while strong and/or sustained upregulation or downregulation of autophagosome levels is required to meaningfully modify sleep (Figure 9B–D). At present, it is unclear whether the small, transient fluctuations we observed in autophagosome number during a typical single day:night cycle are able to feed back on sleep regulation, or whether sleep unidirectionally regulates autophagy when their relationship is not perturbed by other factors.
This relationship between sleep and autophagy nonetheless has interesting implications for pathology. For instance, maladaptive autophagy flux provides a potential mechanism that could reinforce sleep loss in chronic sleep loss disorders. While a single night of SD causes only a modest elevation of neuronal autophagosome number (Figure 8E–H), over many daily cycles chronic sleep loss could establish a deleterious positive feedback loop, with cumulative accumulation of autophagosomes becoming a strong enough wake-promoting cue to further suppress sleep.
This could also represent a mechanism coupling sleep loss disorders to increased incidence of neurodegeneration, and a driver of progressively worsening sleep disturbance noted over the course of many neurodegenerative disorders (Winer and Mander, 2018). Neurodegenerative disorders such as Alzheimer’s disease are characterized by aggregating pathological proteins that strongly inhibit the autolysosomal clearance of autophagosomes (Lee et al., 2010; Ling et al., 2009; Nixon et al., 2005). Chronically elevated autophagosome levels in this context may disrupt sleep much like aus, and chronic sleep loss may in turn exacerbate autophagosome accumulation and further depress sleep, again forming a deleterious feedback loop that in this case begins with extended perturbation of autophagy rather than sleep.
This then begs the etiological question of why the sleep system would evolve such a potentially disastrous feedback loop with the autophagy pathway, one of its regulated outputs. The particularly strong sleep-promoting effects of neuronal atg1 knockdown provide a potential clue (Figure 7A, C and D). Starvation is a well-known inducer of Atg1-dependent autophagy, and food scarcity is a common situation in nature that calls for both high levels of autophagy and suppression of sleep to allow for foraging (Chang et al., 2009; Erion et al., 2012; Hale et al., 2013). Thus, under starvation, the relationship we report for sleep and autophagosome levels would be adaptive. Given that the aus sleep-loss phenotype traces at least in part to neuropeptidergic populations, which are implicated in autophagy and also in behaviors such as feeding and sleep in Drosophila (Bhukel et al., 2019; Dubowy and Sehgal, 2017; Dus et al., 2015; Lieberman et al., 2020; Melcher et al., 2007), these populations may be particularly important for integrating homeostatic phenomena via changes in autophagosome levels. Examples of homeostatic integration are provided by findings that food-motivated learning in fruit flies is disrupted on a high-calorie diet, and that sleep is uncoupled from Drosophila memory consolidation by starvation (Chouhan et al., 2021; Zhang et al., 2015).
Alterations in autophagosome level, or perhaps contents, could integrate external and internal nutritive cues and differentially promote coupling of learning and memory to the food and/or sleep homeostats based on the fly’s needs in a given situation. Indeed, it is tempting to speculate that under conditions of low sleep need and autophagosome level, autophagosomes may be important for clearing waste and maintaining overall cellular health, while very strong or prolonged disruptions to sleep or autophagy constitute a stress response able to modify behavior to adapt to environmental conditions. While we focus on the sleep homeostat in this manuscript, sleep’s link to autophagy may be important for integrating sleep with not just the feeding homeostat, but also circadian rhythms and other biological drives more generally. Indeed, the well-documented involvement of autophagy in a range of nutritive, maintenance, stress-response, developmental, and other cellular functions could potentially position it as a cell-autonomous integrator of homeostatic needs writ large.
Materials and methods
Fly stocks
Request a detailed protocolThe argus mutant line was obtained in a chemical mutagenesis screen as described previously (Shi et al., 2014). Several Drosophila lines used to interrogate the argus allele, including aus2k-Gal4, both cg16791 over-expression lines, and the cg16791 Crispr mutant, were developed by our laboratory (see below). Mapping stocks, insertion mutants, some RNAi, and Gal4 lines were acquired from the Bloomington Drosophila Stock Center at Indiana. Other RNAi lines were acquired from the Vienna Drosophila Resource Center in Austria or the Kyoto Stock Center in Japan. See Supplementary file 1, Tab1 and Supplementary file 3, Tab 1 for details including stock center ID, genetic background, and figure-by-figure breakdown of all Drosophila lines used in this manuscript.
Behavioral analysis
Request a detailed protocolFlies were housed individually in glass tubes in Percival incubators. Beam-break activity was recorded with the Trikinetics DAM system (http://www.trikinetics.com/). Pysolo (http://www.pysolo.net) and custom Matlab software were used to analyze and plot sleep patterns (Gilestro and Cirelli, 2009; Hsu et al., 2020). All flies were entrained prior to and maintained on a 12 hr:12 hr light:dark cycle for all behavior experiments, except where otherwise noted. Most behavior experiments examined behavior in flies that were ~3–5 days old at the start of recording for durations of up to 6 days, except where otherwise stated. Behavior experiments including homozygous aus groups examined sleep in flies of all groups that were ~3–7 days old at the start of recording for durations of up to 6 days. We expanded the acceptable age range for experiments including aus to allow us to maximize collections from a number of crosses with the aus allele that yielded few progeny.
Mapping the argus locus
Request a detailed protocolClassical genetic mapping with phenotypic markers was conducted for the argus allele very similarly to how we previously isolated redeye (Shi et al., 2014). The minimal overlap narrowed down the location of argus to the region distal of ebony.
SNP mapping: Genomic DNA of homozygous recombinants was subject to SNP analysis. SNP19M and SNP24M primers (Supplementary file 1, Tab2) were used for PCR amplification, and identified nucleotide polymorphism between wild type and the marker line. Scoring of recombinant progeny for aus further narrowed the locus to a ~ 5 M bases region between SNPs.
Deep Sequencing: Illumina paired-end DNA library kit was used to make genomic DNA libraries of iso31 and aus homozygotes. The libraries were amplified ten times through PCR prior to Illumina Hi-Seq analyses. SNP calling algorithm identified polymorphisms.
Molecular cloning
Request a detailed protocolAus promoter Gal4 constructs: Aus2kGal4 primers were used to amplify the aus 2 kb promoter region from genomic DNA derived from iso31, and cloned into pBPGw (Addgene #17574). aus cDNA clones: UAS-aus primers were used to amplify a truncated aus CDS from an iso31 cDNA library and cloned into a pUAST-attB vector. UAS-ausFL primers were used to amplify the full-length aus cDNA from an iso31 cDNA library and cloned into a pUAST-attB construct.
The PhiC31 integration system was adapted to target ausP-Gal4 constructs or UAS-aus(cDNA) constructs onto attP40 site on the 2nd chromosome or attP2 site on the 3rd chromosome.
Two gRNAs designed to generate a CG16791/aus knockout allele using the CRISPR/Cas9 system were cloned into pCFD4 (Addgene#49411) (Port et al., 2014). Separate primer sets were used to amplify and verify the target sequence. gRNA and primer sequences in Supplementary file 1, Tab 2. pHD-DsRed-attp-CG16791 vector: Approximately 1 kb upstream and downstream of the argus gene (CG16791) were PCR amplified using iso31 genomic DNA as a template. The 5’ CG16791 arm was PCR amplified. The PAM sequence CCG inside the 5’ arm was changed to GCG in the reverse primer (see underline) to prevent potential cutting by Cas9. The 3’ CG16791 arm was amplified by cloning primers, and the PAM sequence inside 3’ arm was changed from CCT to GCT using PAM elimination primers to prevent potential cutting by Cas9. PCR products of the 5’ and 3’ CG16791 arms were cloned into a SmaI site in the pBS-KS vector. After the construct was confirmed by sequencing with T7 and T3 primers, 5’ and 3’ arms were processed with AarI and SapI restriction enzymes, respectively, and inserted into AarI and SapI sites in pDsRed-attP (Addgene#51019). See Supplementary file 1, Tab2 for primer sequences.
The pCFD4-CG16791 gRNAx2 vector and pHD-DsRed-attp-CG16791 vector were mixed to final concentrations of 0.1 μg/μl and 0.5 μg/μl, respectively and injected into vas-Cas9 embryos by the Rainbow transgenic service. A single G0 male was crossed with Chr3 balancer virgin females to establish the line. Only G1 flies expressing DsRed in the eye were tested by extraction of gDNA followed by PCR. Further confirmation was done by southern blotting. The correct gene targeting lines were saved for testing in behavior assays. Knock-out flies (CG16791KO) were back-crossed with the iso31 strain for several times and tested for behavior.
Nucleic acid extraction and analysis
Request a detailed protocolDNA Isolation: Flies (~15) were homogenized in DNA extraction buffer (100mMTris pH7.5; 100 mM EDTA; 100 mM NaCl; 0.5 % SDS). gDNAs were then isolated by sequential LiCl/KAc and isopropanol precipitations, and resuspended in TE for subsequent analysis.
Southern blot analysis: Roche Digoxin kit (Cat# 11093657910) was used to label DsRed DNA probes generated by PCR, using primers recorded in Supplementary file 1. Genomic DNA was digested with restriction enzymes and separated on 1% agarose gel before transfer to a nylon membrane. Digoxin labeled probe was hybridized with the membrane at 42°C overnight. After washing, the membrane was exposed with a chemi-luminescence reaction through anti-Digoxin conjugated alkaline phosphatase (Cat# 11093274910).
RNA: Adult fly heads (~15) were subject to Trizol extraction (Ambion). High-capacity cDNA reverse transcription kits (Applied Biosystems) were used to make cDNA libraries.
Autophagy RNAi Screen for Sleep Behavior actinGS+ dicer and nsybGS+ dicer were separately crossed to RNAi’s for genes with known roles in autophagy (Supplementary file 3). We initially measured total sleep in up to 16 female flies on 5% sucrose-agar food laced with 500 uM Sigma-Aldrich mifepristone / RU486 (Cat#: M8046) in ethanol vehicle (RU+ food), averaging sleep across days 4–5 of exposure to drug. Crosses with a median total sleep two hours or more higher or lower than both GS+ dicer and RNAi controls were considered possible hits (primary criterion; Supplementary file 3). In cases where different RNAi’s for the same gene gave initial hits of opposite direction, we excluded both to avoid probable RNAi off-target effects (secondary criterion; Supplementary file 3). Finally, remaining possible hits were re-run a second time, measuring sleep under the same conditions as the initial screen. Crosses statistically different in the same direction from both controls in the combined runs (tertiary criterion) were considered RNAi hits (Figure 7—figure supplement 1).
For individual genes with multiple consistent RNAi hits (atg1 and atg8b), we backcrossed five generations to iso31, then ran a more detailed analysis of sleep in females on both RU+ and ethanol vehicle laced (RU-) food, using the same crosses that gave hits in our screen. To confirm knockdown of target transcripts, we also crossed these alleles to actinGS+ dicer and harvested RNA from pools of 5 RU+ fed whole female flies with a Qiagen RNeasy Miniprep Plus kit (Cat# 74134). gDNA was removed by both included eliminator columns, and on-column Qiagen RNase-free DNAse treatment (Cat# 79254). RNA was reverse transcribed with Lifetech Superscript II Reverse Transcriptase (Cat# 18064071). cDNAs for putative RNAi target genes and alpha-tubulin were amplified using Lifetech SYBR Green PCR mix (Cat# 4364346) and primers in Supplementary file 1 on an Applied Biosystems ViiA7 qPCR machine. We calculated relative transcript levels by ddCT.
Live imaging experiments
Request a detailed protocolBrains from approximately 1 week old adult female flies singly housed on our lab’s standard yeast-molasses food were dissected and mounted in chilled artificial hemolymph (108 mM NaCl; 5 mM KCl; 2 mM CaCl2; 8.2 mM MgCl2-6H2O; 4 mM NaHCO3; 1 mM NaH2PO4-H2O; 5 mM trehalose; 10 mM sucrose; 5 mM HEPES; 265mOsm and pH7.5) (Cohn et al., 2015). They were live imaged embedded in vacuum grease with a 40 X water immersion objective at 1.3 X digital zoom under a Leica confocal microscope at Alexa488 (green) and Alexa594 (red) wavelengths. Z-stacks containing ~60 μm of the central brain starting from the tips of the antennal lobes were captured.
Ilastik machine learning software was trained to isolate all mCherry(+) puncta from our Z-stacks (Berg et al., 2019). Briefly, for each experiment an equal number of representative brains from each group were marked for signal and noise in the red channel by a human scorer to train the Ilastik algorithm. Slices from the front, middle, and back of each stack were used, taking care to mark a range of diverse examples of signal and background. A similar number of markings were made between groups, to avoid biasing the algorithm. Ilastik’s prediction of signal and background was then reversibly overlaid on unmarked sample sections and visually inspected for accuracy by the human scorer. Once the algorithm passed inspection, simple segmentations of all brains were generated by Ilastik to define puncta and background for input into ImageJ. ImageJ was then used to measure mCherry(+) puncta count and size, and each mCherrry puncta’s green channel intensity from GFP. We then thresholded to background GFP intensity within each brain, and counted mCherry(+) puncta with green fluorescence intensity exceeding background to determine autophagosome and autolysosome percentages. The Ilastik algorithm was validated by quantifying autophagy following treatment with the autophagy-inducer rapamycin (see below).
Brains for Ilastik validation were incubated in artificial hemolymph supplemented with either 2 μM LC Laboratories rapamycin (Cat#: R5000) in ethanol vehicle, or ethanol vehicle alone, for ~2 hours prior to imaging. The drug condition was maintained for each group throughout imaging.
For sleep deprivation, flies were placed in DAM monitors in locomotor tubes filled with fresh yeast-molasses food on top of a mechanical deprivator. During the night preceding imaging, flies were shaken for a period of 2 s every 20 s to disrupt sleep, as previously described (Toda et al., 2019).
For sleep induction, flies were flipped from regular yeast-molasses food onto yeast-molasses food supplemented with either Sigma-Aldrich gaboxadol hydrochloride (Cat#: T101) in water vehicle diluted to 0.1 mg/mL final concentration, or water vehicle alone, during ZT0-1. Flies were maintained on the supplemented food for ~12 hr before imaging from ZT12-14. Sleep was recorded for at ~11 hr after flip onto drugged food, to verify that we observed gaboxadol-induced sleep gain as previously described in the same flies whose brains were imaged (Berry et al., 2015; Dissel et al., 2015).
Statistics
Statistics were run in GraphPad Prism or JMP software. Shapiro-Wilkes tests were used to assess normality of each group for each individual experiment. Multiple-comparison correction was appropriately applied where multiple comparisons tested multiple hypotheses, but not where multiple comparisons were made to test a single hypothesis, as in non-geneswitch Gal4 driven RNAi and rescue experiments conducted in the manuscript (Shaffer, 1995).
Data availability
All data generated or analysed during this study are included in the manuscript and supporting files.
References
-
ILASTIK: Interactive machine learning for (bio) analyimageNature Methods 16:1226–1232.https://doi.org/10.1038/s41592-019-0582-9
-
Nutrient-dependent regulation of autophagy through the target of rapamycin pathwayBiochemical Society Transactions 37:232–236.https://doi.org/10.1042/BST0370232
-
Reduced sleep in Drosophila Shaker mutantsNature 434:1087–1092.https://doi.org/10.1038/nature03486
-
Sleep restores behavioral plasticity to Drosophila mutantsCurr. Biol. CB 25:1270–1281.https://doi.org/10.1016/j.cub.2015.03.027
-
Interaction between Sleep and Metabolism in Drosophila with Altered Octopamine SignalingThe Journal of Biological Chemistry 287:32406–32414.https://doi.org/10.1074/jbc.M112.360875
-
blue cheese Mutations Define a Novel, Conserved Gene Involved in Progressive Neural DegenerationThe Journal of Neuroscience 23:1254–1264.
-
On variation in some cell organelles during formation of reserve substances in fatty bodies of Drosophila larvaeZeitschrift Fur Zellforschung Und Mikroskopische Anatomie 61:56–95.https://doi.org/10.1007/BF00341522
-
pySolo: a complete suite for sleep analysis in DrosophilaBioinformatics 25:1466–1467.https://doi.org/10.1093/bioinformatics/btp237
-
A dual function for Deep orange in programmed autophagy in the Drosophila melanogaster fat bodyExperimental Cell Research 312:2018–2027.https://doi.org/10.1016/j.yexcr.2006.03.002
-
The Brain’s Glymphatic System: Current ControversiesTrends in Neurosciences 43:458–466.https://doi.org/10.1016/j.tins.2020.04.003
-
A Wacky Bridge to mTORC1 DimerizationDevelopmental Cell 36:129–130.https://doi.org/10.1016/j.devcel.2016.01.006
-
Extensive Involvement of Autophagy in Alzheimer Disease: An Immuno-Electron Microscopy StudyJournal of Neuropathology and Experimental Neurology 64:113–122.https://doi.org/10.1093/jnen/64.2.113
-
Multiple Hypothesis TestingAnnual Review of Psychology 46:561–584.https://doi.org/10.1146/annurev.ps.46.020195.003021
-
The beach domain is critical for blue cheese function in a spatial and epistatic autophagy hierarchyFrontiers in Cell and Developmental Biology 7:129.https://doi.org/10.3389/fcell.2019.00129
-
Bidirectional Regulation of Sleep and Synapse Pruning after Neural InjuryCurrent Biology 30:1063–1076.https://doi.org/10.1016/j.cub.2019.12.065
-
FlyBase 2.0: the next generationNucleic Acids Research 47:D765.https://doi.org/10.1093/nar/gky1003
-
Neurexin regulates nighttime sleep by modulating synaptic transmissionScientific Reports 6:38246.https://doi.org/10.1038/srep38246
Article and author information
Author details
Funding
Howard Hughes Medical Institute
- Amita Sehgal
National Institutes of Health (F32AG056081-03)
- Joseph L Bedont
National Institutes of Health (K99NS118561-01)
- Joseph L Bedont
The funders had no role in study design, data collection and interpretation, or the decision to submit the work for publication.
Acknowledgements
We thank Han Wang and Zhifeng Yue for assistance with fly work. We also thank Ana Maria Cuervo for helpful suggestions regarding autophagy measurements and Taichi Hara, Toshifumi Tomoda, and Nobuo Noda for critical comments on this manuscript.
Copyright
© 2021, Bedont et al.
This article is distributed under the terms of the Creative Commons Attribution License, which permits unrestricted use and redistribution provided that the original author and source are credited.
Metrics
-
- 4,548
- views
-
- 511
- downloads
-
- 36
- citations
Views, downloads and citations are aggregated across all versions of this paper published by eLife.
Citations by DOI
-
- 36
- citations for umbrella DOI https://doi.org/10.7554/eLife.64140