Insulin-producing β-cells regenerate ectopically from a mesodermal origin under the perturbation of hemato-endothelial specification
Abstract
To investigate the role of the vasculature in pancreatic β-cell regeneration, we crossed a zebrafish β-cell ablation model into the avascular npas4l mutant (i.e. cloche). Surprisingly, β-cell regeneration increased markedly in npas4l mutants owing to the ectopic differentiation of β-cells in the mesenchyme, a phenotype not previously reported in any models. The ectopic β-cells expressed endocrine markers of pancreatic β-cells, and also responded to glucose with increased calcium influx. Through lineage tracing, we determined that the vast majority of these ectopic β-cells has a mesodermal origin. Notably, ectopic β-cells were found in npas4l mutants as well as following knockdown of the endothelial/myeloid determinant Etsrp. Together, these data indicate that under the perturbation of endothelial/myeloid specification, mesodermal cells possess a remarkable plasticity enabling them to form β-cells, which are normally endodermal in origin. Understanding the restriction of this differentiation plasticity will help exploit an alternative source for β-cell regeneration.
Introduction
The concept of embryonic development and cell fate determination was illustrated by the famous Waddington landscape model decades ago (Waddington, 1957). Waddington’s model not only shows the importance of spatiotemporal precision in cell differentiation but also metaphorises cell fate determination as a sequential and irreversible event. In this hierarchical model, the endoderm follows the lineage paths downwards and progressively differentiates into multiple endodermal cell types, including pancreatic β-cells. Likewise, the mesoderm stays in the mesodermal lineage paths and differentiates into endothelial cells and other mesodermal cell types. However, in recent decades, multiple studies have suggested that committed cells are capable of differentiating across the germ layer boundaries by converting embryonic and/or adult mesodermal fibroblasts into ectodermal neuronal cells (Vierbuchen et al., 2010), multipotent neural stem cells (Ring et al., 2012), endodermal hepatocyte-like cells (Huang et al., 2011; Sekiya and Suzuki, 2011), or pancreatic β-like cells (Zhu et al., 2016) in vitro. These studies highlight the feasibility of converting mesodermal cells into ectodermal or endodermal cells in vitro.
Despite the extensive studies on cell fate conversion across germ layers in vitro, the number of in vivo studies is limited. Ectopic expression of Xsox17β in Xenopus embryos relocated cells normally fated to become ectoderm to appear in the gut epithelium, suggesting a possible change in cell fate in vivo (Clements and Woodland, 2000). Similarly, ectopic expression of sox32/casanova in presumptive endothelial cells switched their cell fate to endoderm in zebrafish embryos (Kikuchi et al., 2001). Furthermore, aggregated morulae and chimeric mouse embryos of β-catenin mutants provided evidence of precardiac mesoderm formation in endodermal tissues in vivo (Lickert et al., 2002). These studies suggest that the classical in vivo germ layer boundaries may not be as clear-cut as previously thought.
In this study, we aimed to elucidate the importance of the vasculature in pancreatic β-cell regeneration, which plays a crucial role in potential therapeutic strategies against diabetes. We employed cloche zebrafish mutants as an avascular model. The mutation of npas4l, a master regulator of endothelial and hematopoietic cell fates, is responsible for the severe loss of most endothelial and blood cells in cloche mutants (Parker and Stainier, 1999; Reischauer et al., 2016; Stainier et al., 1995). Unexpectedly, the npas4l mutation induced ectopic β-cell formation in the mesenchymal region outside of the pancreas after β-cell ablation. Lineage-tracing mesodermal cells expressing draculin (drl), npas4l, and etsrp (previously named etv2) validated the mesodermal lineage of the ectopic β-cells, which are normally endodermal in origin. These findings offer novel insights into cell fate determination and an alternative source of β-cells.
Results
Ectopic β-cell formation in npas4l mutants
To determine the importance of vasculogenesis and vascularisation for β-cell regeneration, we examined β-cell formation in zebrafish carrying the cloche mutation (npas4ls5 mutation) after β-cell ablation, i.e., in the Tg(ins:Flag-NTR);Tg(ins:H2BGFP;ins:DsRed) model. Nitroreductase (NTR), expressed under the insulin promoter, converts the prodrug metronidazole (MTZ) to a cytotoxin to specifically ablate insulin-producing β-cells (Curado et al., 2007). The homozygous mutation of npas4l significantly increased the number of ins:H2BGFP-positive cells during the β-cell regeneration period (Figure 1A–C). In addition, we observed a distinctive ectopic β-cell population in the mesenchymal region outside of the pancreas in the npas4l−/− group, an ectopic location that was very rarely observed in the sibling controls (including both wild-type and heterozygous siblings). This ectopic population of β-cells contributed to the major increase in the number of ins:H2BGFP-positive cells during β-cell regeneration (Figure 1C). Moreover, the comparable and sparse numbers of ins:DsRed-positive cells in the controls and mutants indicate that the npas4l mutation did not enhance the survival of β-cells during the ablation (Figure 1A,B) because the extended maturation time of DsRed (Baird et al., 2000) restricted the detection of DsRed to any surviving β-cells.
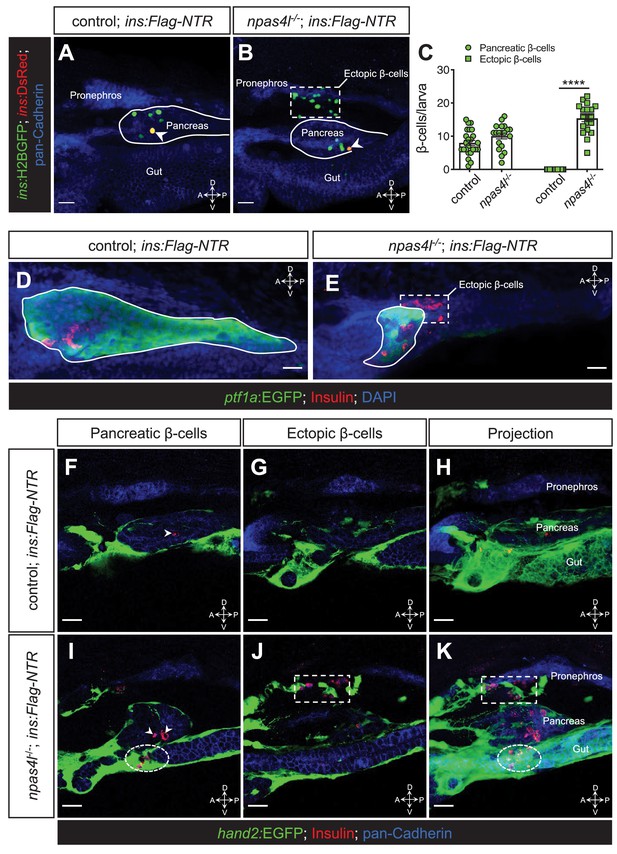
Ectopic β-cell formation in npas4l mutants.
(A, B) Representative confocal projections of the pancreas and neighbouring tissues of control siblings and npas4l−/− Tg(ins:Flag-NTR);Tg(ins:H2BGFP;ins:DsRed) zebrafish larvae at 3 dpf after β-cell ablation by MTZ at 1–2 dpf, displaying regenerated β-cells in green and older β-cells that likely survived the ablation in yellow overlap as the DsRed fluorescence driven by the insulin promoter remained in these cells, at the same time as DsRed had not had enough time to mature in the regenerated β-cells (arrowheads). The ectopic β-cells are indicated by white dashed rectangle. Pancreata are outlined by solid white lines. (C) Quantification of the pancreatic or ectopic β-cells per larva at 3 dpf. ****p<0.0001 (Šidák’s multiple comparisons test); n = 24 (control) and 19 (npas4l−/−). Quantification data are represented as the mean ± SEM. (D, E) Representative image projections of the pancreas and neighbouring tissues in control siblings and npas4l-/- Tg(ins:Flag-NTR);Tg(ptf1a:EGFP) larvae at 3 dpf after β-cell ablation by MTZ at 1–2 dpf, displaying β-cells in red with immunostaining for insulin and ptf1a:EGFP+ exocrine pancreas in green. Pancreata are outlined by solid white lines. Dashed line outlines ectopic β-cells in the mesenchyme (E). (F–K) Representative images and projections of the pancreas and neighbouring mesenchyme of control siblings and npas4l-/- Tg(ins:Flag-NTR);Tg(hand2:EGFP) zebrafish larvae at 3 dpf after β-cell ablation by MTZ at 1–2 dpf, displaying β-cells in red with immunostaining for insulin and hand2:EGFP+ mesenchyme in green. White arrowheads point to β-cells in the pancreas (F, I). Dashed rectangles indicate the ectopic β-cells intermingling with the mesenchyme between the pronephros and the pancreas, without co-expressing insulin and hand2:EGFP (J, K). Selected area in dashed ovals shows other ectopic β-cells intermingling with the mesenchyme ventral to the pancreas (I, K). Scale bars = 20 μm. Anatomical axes: D (dorsal), V (ventral), A (anterior), and P (posterior).
To visualise the location of the ectopic β-cells better, we labelled the pancreas with ptf1a:EGFP expression and observed not only a drastic reduction in the pancreas size (Figure 1D,E, Figure 1—figure supplement 1) but also the regeneration of β-cells clearly outside of the ptf1a-expressing exocrine pancreas in npas4l mutants (Figure 1E). By labelling the mesenchyme with hand2:EGFP expression (Figure 1F–K), we further revealed that the majority of the ectopic β-cells formed in npas4l mutants intermingled with hand2:EGFP-positive mesenchymal cells between the pronephros and the pancreas (Figure 1J,K). In addition, we occasionally observed ectopic β-cells intermingled with hand2:EGFP-positive mesenchymal cells ventral to the pancreas (Figure 1I,K). Although the ectopic β-cells were located among the mesenchymal cells, they did not express hand2:EGFP.
The ectopic β-cells co-express insulin and endocrine markers in npas4l mutants
Next, we examined multiple pancreatic endocrine and β-cell markers, including Isl1, neurod1, pdx1, mnx1, pcsk1, and ascl1b (the functional homologue to Neurog3 in mammals), to validate the β-cell identity of the ectopic insulin-producing cells. The majority of the ectopic β-cells co-expressed insulin as well as these markers during β-cell regeneration (Figure 2). The high co-expression of pcsk1 (Figure 2R,S, Table 1), which encodes an enzyme necessary for insulin biosynthesis, indicates that most of the β-cells in the ectopic population are likely functional. Consistent with previous findings in pancreatic β-cells, not all ectopic β-cells expressed ascl1b:EGFP (Figure 2V,W, Table 1), which suggests that ascl1b works as a transient endocrine cell fate regulator (Flasse et al., 2013). In contrast with Isl1, mnx1, pcsk1, and ascl1b, we observed lower co-expression levels of neurod1 and pdx1 in ectopic β-cells compared with the pancreatic population in npas4l mutants (Table 1). In addition to the reduction in pancreas size (Figure 1—figure supplement 1), the pdx1-expressing pancreatic duct was also reduced in the npas4l mutant (Figure 2—figure supplement 1), indicating that the pancreas and its duct did not expand to form the ectopic β-cells. These observations together suggest that the pancreatic and ectopic β-cells are similar, yet they are two distinct β-cell populations.
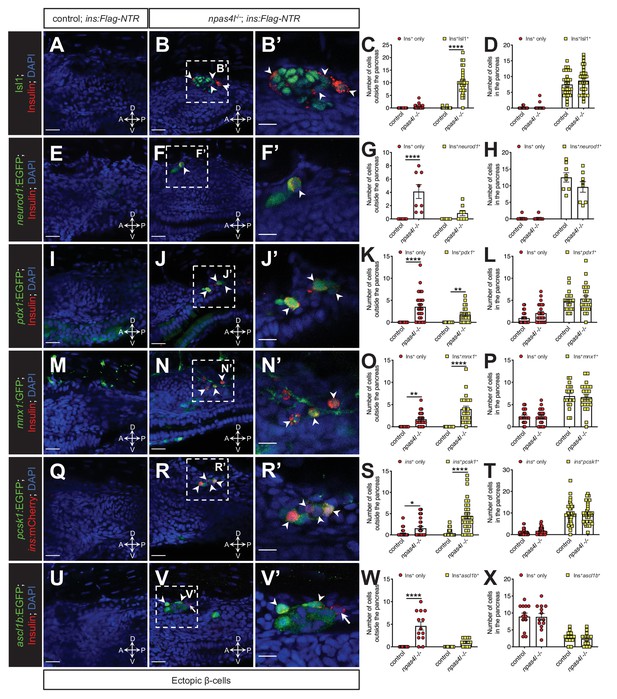
The ectopic β-cells co-express insulin and endocrine markers in npas4l mutants.
Representative confocal images of the tissues adjacent to the pancreas of control siblings and npas4l−/− Tg(ins:Flag-NTR) zebrafish larvae at 3 dpf after β-cell ablation by MTZ at 1–2 dpf, displaying cells expressing pancreatic endocrine cell markers Isl1 (A–B’), neurod1 (E–F’), pdx1 (I–J’), mnx1 (M–N’), pcsk1 (Q–R’), and ascl1b (U–V’) in green, and ectopic β-cells in red with immunostaining for insulin (except Q–R’ with the mCherry fluorescence driven by the insulin promoter). Arrowheads point to ectopic β-cells that express corresponding markers. Arrows point to β-cells that do not express ascl1b (V and V’). B’, F’, J’, N’, R’, and V’ are magnified from the areas indicated by the white dashed square in B, F, J, N, R, and V, respectively. Quantification of β-cells with or without corresponding marker expression in the ectopic location (C, G, K, O, S, and W) or in the pancreas (D, H, L, P, T, and X) per larva at 3 dpf. *p=0.0310, **p=0.0039, and ****p<0.0001 (Šidák’s multiple comparisons test); (C, D) n = 30 (control) and 31 (npas4l−/−); (G, H) n = 8 (control) and 8 (npas4l−/−); (K, L) n = 25 (control) and 24 (npas4l−/−); (O, P) n = 21 (control) and 23 (npas4l−/−); (S, T) n = 40 (control) and 32 (npas4l−/−); (W and X) n = 13 (control) and 13 (npas4l−/−). Data are represented as the mean ± SEM. Scale bars = 20 μm except B’, F’, J’, N’, R’, and V’ (10 μm). Anatomical axes: D (dorsal), V (ventral), A (anterior), and P (posterior).
Percentages of pancreatic or ectopic cells co-expressing insulin and corresponding marker gene or protein in npas4l mutants.
Marker gene/protein | Pancreatic co-expression (%) | Ectopic co-expression (%) |
---|---|---|
Isl1 | 97.9 | 96.8 |
neurod1 | 97.5 | 17.5 |
pdx1 | 72.1 | 32.8 |
mnx1 | 75.3 | 70.2 |
pcsk1 | 85.1 | 74.1 |
ascl1b | 21.2 | 17.8 |
Ectopic β-cells can respond to glucose by displaying calcium oscillations
We further assessed the functionality and maturity of the ectopic β-cells by examining the calcium ion oscillation in vivo to determine their ability in responding to glucose to secrete insulin in the Tg(ins:GCaMP6s);Tg(ins:mCherry);Tg(ins:Flag-NTR) npas4l zebrafish mutants. After β-cell ablation by MTZ and one day of β-cell regeneration, we found comparable proportions of pancreatic (66%) and ectopic (67%) β-cells displaying mild and basal levels of calcium activity at the baseline (Figure 3 and Video 1). Upon glucose treatment, 50% of pancreatic β-cells and 33% of ectopic β-cells elicited calcium oscillations, suggesting that a portion of the pancreatic and ectopic β-cells have the ability to respond to glucose to secrete insulin in the npas4l mutants during β-cell regeneration.
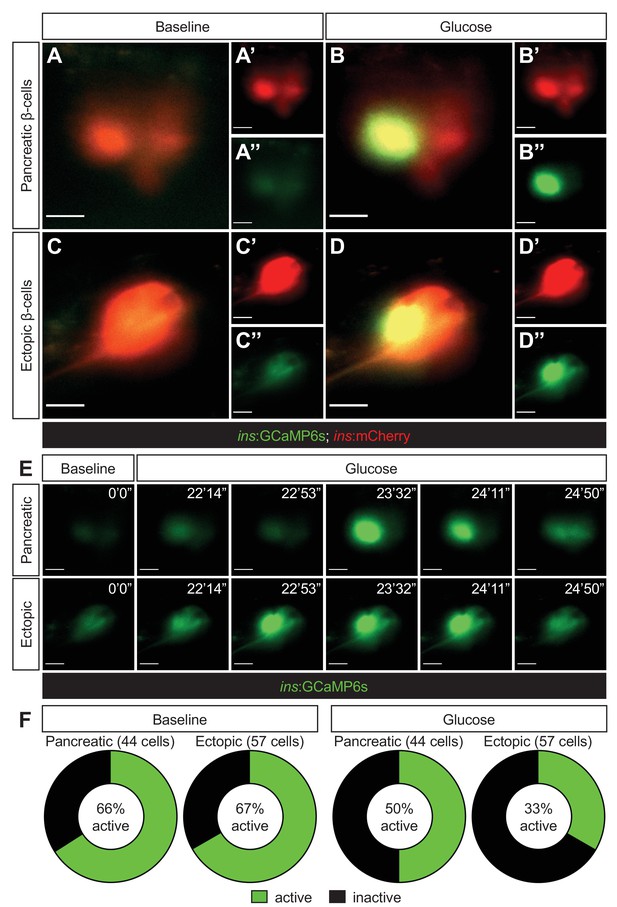
Ectopic β-cells can respond to glucose by displaying calcium oscillations.
Representative images captured from a live calcium imaging video of npas4l−/−Tg(ins:GCaMP6s);Tg(ins:mCherry);Tg(ins:Flag-NTR) zebrafish larvae at 3 dpf after β-cell ablation by MTZ from 1 to 2 dpf, showing β-cells expressing ins:mCherry in red and displaying calcium activity in green in the pancreatic (A, B) or ectopic (C, D) domain at the baseline (A, C) or after the addition of 200 mM of glucose to the E3 medium (B, D). (E) Sequential images captured from a live calcium imaging video to show the oscillation of calcium signalling at the baseline and after the glucose treatment, displaying the GCaMP6s signal in green. (F) Quantification of pancreatic or ectopic β-cells displaying calcium activity at the baseline or after glucose treatment in 11 (pancreatic) or 17 (ectopic) npas4l zebrafish mutants. Scale bars = 10 μm.
Live calcium imaging of β-cells in a npas4l mutant.
An example video of live calcium imaging of both pancreatic and ectopic β-cells in a Tg(ins:GCaMP6s);Tg(ins:mCherry);Tg(ins:Flag-NTR) npas4l mutant at 3 dpf after β-cell ablation from 1 to 2 dpf, displaying GCaMP6s signal in green. Glucose (200 mM) was added to the E3 media at around 9 min in the video.
The ectopic β-cells are of mesodermal origin in npas4l mutants and etsrp morphants
We have previously shown that npas4l expression is first initiated in the lateral plate mesoderm at the tailbud stage by in situ hybridisation (Reischauer et al., 2016). In this study, we examined npas4l expression at 20 hr postfertilisation (hpf) and found that npas4l was severely reduced in the lateral plate mesoderm in the npas4l mutants (Figure 4—figure supplement 1A,B), whereas normal expression levels were observed in the tailbud and brain. The cells with reduced npas4l expression were still present in the lateral plate mesoderm as demonstrated by the embryos incubated overnight to further develop the npas4l expression signal (Figure 4—figure supplement 1B’). On the contrary, there were no significant differences in the expression of the examined early mesodermal or endodermal transcription factors foxa2, gsc, and mixl1, or the pancreatic endocrine progenitor marker ascl1b (Figure 4—figure supplement 1C–J). Because the ectopic β-cells induced by the npas4l mutation also resided in the mesenchymal region, and npas4l can act cell-autonomously to affect the hematopoietic and endothelial lineages (Parker and Stainier, 1999), we hypothesised that the ectopic β-cells originated from a mesodermal lineage.
To determine whether the mesoderm was the origin of the ectopic β-cells, we genetically traced the mesodermal cells using drl:CreERT2, a tamoxifen-inducible Cre transgene driven by a drl promoter (Mosimann et al., 2015). The spatial expression pattern of drl in the npas4l mutants resembled that in the sibling controls (Figure 4—figure supplement 2), suggesting that npas4l mutation did not induce any ectopic expression of drl to disrupt the lineage-tracing approach. In combination with a −3.5ubb:LOXP-EGFP-LOXP-mCherry (ubi:Switch) reporter (Mosimann et al., 2011), the drl-expressing mesodermal cells were labelled in red in Tg(drl:CreERT2);Tg(ubi:Switch);Tg(ins:Flag-NTR) (drl-tracing) zebrafish larvae after 4-hydroxytamoxifen (4-OHT) induction (Figure 4A, Figure 4—figure supplement 3). We treated the transgenic embryos with 4-OHT at 10–12 hpf. We chose to label the mesodermal cells during this period as neither endothelial/hematopoietic cells nor β-cells have developed at that stage, i.e., to exclude confounding effects of endothelial/hematopoietic cells or possible ectopic expression of the lineage tracer in the β-cells of the npas4l mutant. To ablate the β-cells, we incubated the 4-OHT-treated transgenic embryos in MTZ at 1–2 days postfertilisation (dpf). We allowed the β-cells to regenerate for 30 hr before we fixed the larvae at 3 dpf for immunostaining (Figure 4B).
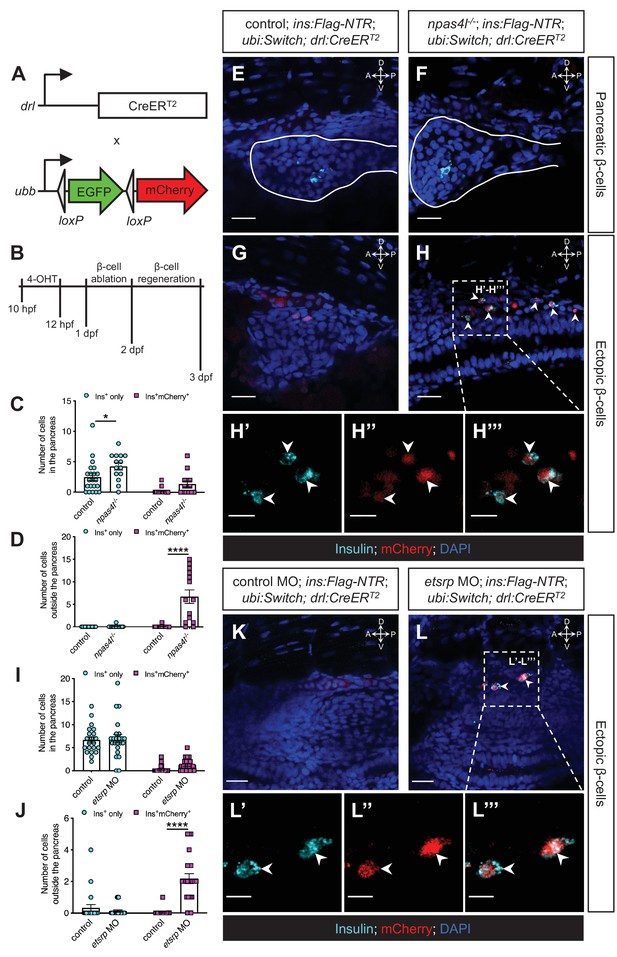
The ectopic β-cells are of mesodermal origin in npas4l mutants and etsrp morphants.
(A) Constructs of −6.35drl:Cre-ERT2 (drl:CreERT2) and −3.5ubb:LOXP-EGFP-LOXP-mCherry (ubi:Switch). Upon 4-OHT induction between 10 and 12 hpf, Cre recombinase expressed by the drl promoter cleave the loxP sites to allow ubb:mCherry expression in the cells that once expressed drl. (B) Scheme for tracing the mesodermal lineage of ectopic β-cells in control siblings and npas4l−/− Tg(ins:Flag-NTR);Tg(ubi:Switch);Tg(drl:CreERT2) zebrafish larvae. (C, D, I, and J) Quantification of the pancreatic or ectopic β-cells with or without mesodermal lineage in npas4l mutants (C, D) or etsrp morpholino (MO)-injected larvae (I, J) at 3 dpf. *p=0.0227 and ****p<0.0001 (Šidák’s multiple comparisons test); (C, D) n = 21 (control) and 14 (npas4l−/−) or (I, J) n = 21 (control) and 23 (etsrp MO). Data are represented as the mean ± SEM. Standard control MO (4 ng) and etsrp MO2 (4 ng) were injected to the control group and etsrp MO group respectively at the one-cell stage (I, J, K–L’’’). (E–H’’’ and K–L’’’) Representative confocal images of pancreatic (E, F) or ectopic β-cells (G, H) of control siblings and npas4l−/−, or ectopic β-cells in control or etsrp MO-injected (K, L) Tg(ins:Flag-NTR);Tg(ubi:Switch);Tg(drl:CreERT2) zebrafish larvae at 3 dpf after β-cell ablation by MTZ at 1–2 dpf, displaying β-cells in cyan with immunostaining for insulin and lineage-traced cells derived from drl-expressing mesodermal cells in red from the Cre-recombined ubi:Switch. Pancreata are outlined by solid white lines (E, F). Arrowheads point to ectopic β-cells derived from the mesoderm (H–H’’’ and L–L’’’). Selected areas in dashed squares in (H) and (L) are magnified in split (H’, H’’, L’ and L’’) and merged (H’’’ and L’’’) channels, respectively. Scale bars = 20 μm (E–H, K, and L) or 10 μm (H’–H’’’ and L’–L’’). Anatomical axes: D (dorsal), V (ventral), A (anterior), and P (posterior).
Immunostaining against insulin displayed a normal set of β-cells in the pancreas of the drl-tracing larvae with or without npas4l mutation after 30 hr of regeneration (Figure 4C,E, and F). In line with the findings shown in Figure 1, the npas4l mutation induced the formation of ectopic β-cells in the mesenchymal region (Figure 4D,G, and H). Furthermore, 98.9% of the ectopic β-cells in the mesenchymal region were mCherry-positive (Figure 4H’–H’’’), indicating that they derived from the drl-expressing mesodermal cells.
With a similar setting, we injected the drl-tracing embryos (without any npas4l mutation) with control or etsrp morpholino at the one-cell stage. Npas4l is essential for the expression of etsrp, which is a key regulator of endothelial/myeloid cell specification and vasculogenesis (Reischauer et al., 2016; Sumanas and Lin, 2006). Similar to the npas4l mutation, knocking down etsrp led to the formation of ectopic β-cells (Figure 4I–L’’’). Co-injecting lower doses of two different etsrp morpholinos (MO1 and MO2) exerted a synergistic effect on ectopic β-cell emergence (Figure 4—figure supplement 4), suggesting that the phenotype was not due to an off-target effect. The majority of the ectopic β-cells (94.3%) in etsrp morphants was also lineage-traced back to the drl-expressing mesodermal cells, suggesting that the ectopic β-cell formation was also of mesodermal origin following etsrp knockdown.
The mesodermal cells lose npas4l expression after differentiating into ectopic β-cells
We then further examined the autonomous role of the progenitors of endothelial and hematopoietic cells in ectopic β-cell emergence in npas4l mutants by tracing the npas4l cell lineage with a zebrafish knock-in line, npas4lPt(+36-npas4l-p2a-Gal4-VP16)bns423. This knock-in line not only carries a +36 bp mutation in exon 2 of npas4l (npas4lbns423 mutation) but also expresses the transcription activator Gal4 under control of the endogenous npas4l promoter (npas4l:Gal4), allowing us to trace the npas4l lineage after crossing it into Tg(UAS:Cre);Tg(ubi:Switch) (Figure 5A).
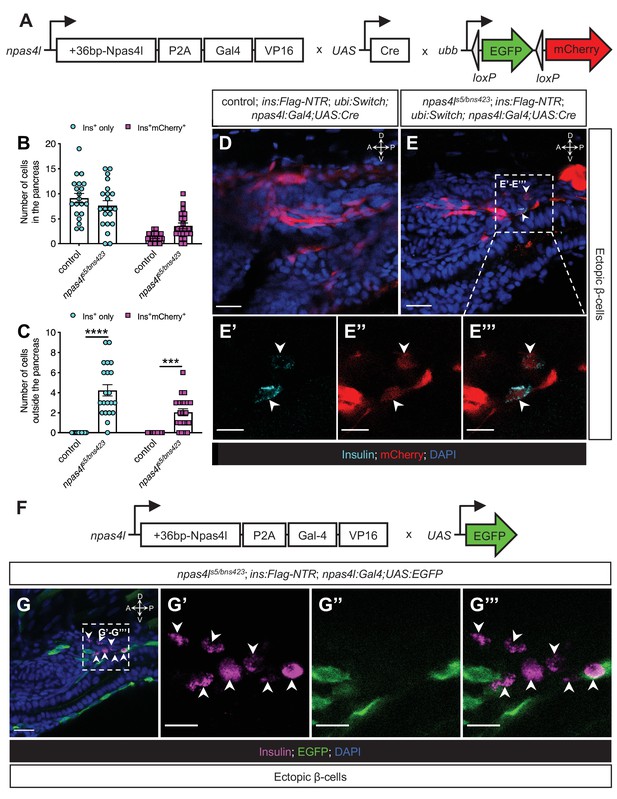
The mesodermal cells lose npas4l expression after differentiating into ectopic β-cells.
(A) Schematics of npas4lPt(+36-npas4l-p2a-Gal4-VP16)bns423 (npas4l:Gal4), UAS:Cre and −3.5ubb:LOXP-EGFP-LOXP-mCherry (ubi:Switch). (B, C) Quantification of the pancreatic or ectopic β-cells with or without npas4l-positive mesodermal origin in control or transheterozygous npas4ls5/bns423 mutants at 3 dpf. ***p=0.0001 and ****P<0.0001 (Šidák’s multiple comparisons test); n = 20 (control) and 21 (npas4ls5/bns423). Data are represented as the mean ± SEM. (D–E’’’) Representative confocal images of ectopic β-cells and npas4l-positive lineage-traced cells in control siblings and npas4ls5/bns423 Tg(ins:Flag-NTR);Tg(ubi:Switch);Tg(npas4l:Gal4);Tg(UAS:Cre) zebrafish larvae at 3 dpf after β-cell ablation by MTZ from 1 to 2 dpf, displaying β-cells in cyan with immunostaining for insulin and lineage-traced cells derived from npas4l-expressing mesodermal cells in red from the Cre-recombined ubi:Switch. The selected area in a dashed square in (E) is magnified in split (E’ and E’’) and merged (E’’’) channels, respectively. Arrowheads point to ectopic β-cells derived from the mesoderm (E–E’’’). (F) Schematics of npas4lPt(+36-npas4l-p2a-Gal4-VP16)bns423 (npas4l:Gal4) and UAS:EGFP. (G–G’’’) Representative confocal images of ectopic β-cells losing npas4l expression in npas4ls5/bns423 Tg(ins:Flag-NTR); Tg(npas4l:Gal4);Tg(UAS:EGFP) zebrafish larvae at 3 dpf after β-cell ablation by MTZ from 1 to 2 dpf, displaying β-cells in magenta with immunostaining for insulin and cells expressing npas4l in green. The selected area in a dashed square in (G) is magnified in split (G’ and G’’) and merged (G’’’) channels, respectively. Arrowheads point to ectopic β-cells without npas4l expression (G–G’’’). Scale bars = 20 μm (D, E, and G) or 10 μm (E’–E’’’ and G’–G’’). Anatomical axes: D (dorsal), V (ventral), A (anterior), and P (posterior).
We obtained a transheterozygous npas4l mutant (npas4ls5/bns423) by crossing this npas4l tracer carrying the npas4lbns423 mutation into the npas4ls5 mutant used in the studies above, and observed similar ectopic β-cell formation (Figure 5B–E’’’) as found in the homozygous npas4ls5 mutant (Figure 1). Some of these ectopic β-cells (32.6%) could be traced back to the npas4l lineage as they were labelled by ubb:mCherry after the Cre-recombination driven by npas4l:Gal4 and UAS:Cre (Figure 5C and E–E’’’), which further supports a mesodermal origin of the ectopic β-cells.
By crossing Tg(npas4l:Gal4) into Tg(UAS:EGFP), the endogenous promoter activity of npas4l could be revealed by EGFP (Figure 5F). The ectopic β-cells emerged in close proximity to mesodermal cells with active npas4l expression in the transheterozygous npas4ls5/bns423 mutants (Figure 5G–G’’’). However, we did not observe any ectopic β-cells with EGFP expression, suggesting that these mesodermal cells have lost the identity of the lateral plate mesoderm with npas4l expression and started to express insulin as in the endodermal pancreatic β-cells instead.
The ectopic β-cells derive from the etsrp-expressing mesodermal lineage in etsrp morphants
To confirm the origin of the ectopic β-cell using a different lineage-tracing approach, we generated Tg(etsrp:Cre) zebrafish, based on the promoter of −2.3estrp:GFP that has been demonstrated to closely recapitulate the endogenous etsrp expression in the lateral plate mesoderm and vasculature (Veldman and Lin, 2012). We then crossed Tg(etsrp:Cre) into Tg(ubi:Switch);Tg(ins:Flag-NTR), and thereby labelling descendants of the etsrp lineage in red (Figure 6A). We validated the efficiency of the etsrp-lineage tracer and revealed that the majority of kdrl-expressing endothelial cells were being traced in the intersegmental vessels (86.6%) and other vasculature (Figure 6—figure supplement 1). At the one-cell stage, we injected the etsrp-tracing embryos with control or etsrp morpholinos. After β-cell ablation by MTZ treatment at 1–2 dpf and β-cell regeneration for 30 hr ectopic β-cells formed in the etsrp morphants, and 73.9% of the ectopic β-cells were labelled in red (Figure 6B–E’’’), illustrating that the etsrp-expressing lineage gave rise to a significant portion of the ectopic β-cells.
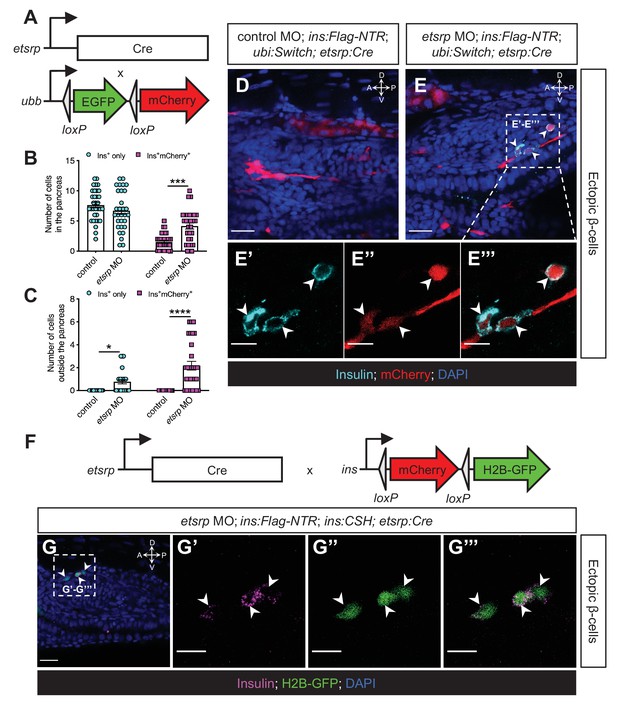
The ectopic β-cells derive from the etsrp-expressing mesodermal lineage in etsrp morphants.
(A) Constructs of etsrp:Cre and −3.5ubb:LOXP-EGFP-LOXP-mCherry (ubi:Switch). (B, C) Quantification of the pancreatic or ectopic β-cells with or without etsrp-positive mesodermal origin in control or etsrp morpholino (MO)-injected larvae at 3 dpf. *p=0.0169, ***p=0.0002, and ****p<0.0001 (Šidák’s multiple comparisons test); n=33 (control) and 30 (etsrp MO). Data are represented as the mean ± SEM. (D–E’’’) Representative confocal images of ectopic β-cells and etsrp-positive lineage-traced cells in control or etsrp MO-injected Tg(ins:Flag-NTR);Tg(ubi:Switch);Tg(etsrp:Cre) zebrafish larvae at 3 dpf after β-cell ablation by MTZ at 1–2 dpf, displaying β-cells in cyan with immunostaining for insulin and lineage-traced cells derived from etsrp-expressing mesodermal cells in red from the Cre-recombined ubi:Switch. The selected area in a dashed square in (E) is magnified in split (E’, E’’) and merged (E’’’) channels, respectively. Arrowheads point to ectopic β-cells derived from the mesoderm (E–E’’’). (F) Constructs of etsrp:Cre and ins:LOXP-mCherry-LOXP-Hsa.HIST1H2BJ-GFP (ins:CSH). (G–G’’’) Representative confocal images of ectopic β-cells derived from the etsrp-expressing lineage in etsrp MO-injected Tg(ins:Flag-NTR);Tg(ins:CSH);Tg(etsrp:Cre) zebrafish larvae at 3 dpf after β-cell ablation by MTZ at 1–2 dpf, displaying β-cells in magenta with immunostaining for insulin and lineage-traced cells derived from etsrp-expressing mesodermal cells in nuclear green from the Cre-recombined ins:CSH. The selected area in a dashed square in (G) is magnified in split (G’ and G’’) and merged (G’’’) channels, respectively. Scale bars = 20 μm (D, E, and G) or 10 μm (E’–E’’’ and G’–G’’’). Anatomical axes: D (dorsal), V (ventral), A (anterior), and P (posterior).
Moreover, we replaced ubi:Switch with ins:LOXP-mCherry-LOXP-Hsa.HIST1H2BJ-GFP (ins:CSH) in the etsrp-tracing zebrafish larvae to directly trace insulin-expressing cells originating from the etsrp-expressing mesodermal lineage (Figure 6F). The co-localisation of insulin staining and the nuclear green tracer further confirms the mesodermal lineage of the ectopic β-cells (Figure 6G–G’’’).
Together, we used three different lineage-tracing models as well as three different loss of function models, that is using the promoter of drl, npas4l, or etsrp to drive Cre in npas4ls5/s5 mutants, npas4ls5/bns423 mutants, or etsrp morphants. This suggests that the ectopic β-cell formation is not restricted to the loss of a specific gene, but rather due to the perturbation of endothelial/myeloid specification.
Discussion
In this study, we first examined the role of blood vessels in β-cell regeneration in the cloche zebrafish mutant, which carries a homozygous npas4l mutation (Reischauer et al., 2016). We then unexpectedly revealed β-cells regenerating ectopically in the mesenchymal area. The ectopic β-cells were likely functional because they expressed several endocrine and β-cell markers, including Isl1, mnx1, and pcsk1, and were capable of responding to glucose to induce calcium oscillations during β-cell regeneration, although we do not know if they possess all the features of bona fide β-cells. By combining in situ hybridisation, lineage tracing, and confocal microscopy, we successfully traced the origin of the ectopic β-cells to the mesodermal lineage. A recent study has reported the conversion of Etsrp-deficient vascular progenitors into skeletal muscle cells and highlighted the plasticity of mesodermal cell fate determination within the same germ layer (Chestnut et al., 2020). Our data demonstrated the plasticity of β-cell differentiation across the committed germ layers in vivo, i.e., switching from a mesodermal to an endodermal fate in a regenerative setting, while gastrulation and cell fate commitment in the germ layers are considered to be irreversible in development. Ectopic pancreata have been observed before, e.g., in Hes1 mutant mice (Fukuda, 2006; Sumazaki et al., 2004), although that has been shown to be through an expansion of the pancreas rather than through changes in cell fate determination across organs or germ layers (Jørgensen et al., 2018). Our discovery is, to our knowledge, the first demonstration of ectopic β-cells with a mesodermal origin in vivo.
The recent genome-wide study with zebrafish embryos has confirmed the crucial role of npas4l in the early specification of endothelium and blood as the expressions of some endothelial and hematopoietic genes like tal1, etsrp, lmo2, gfi1aa, and gata1a were downregulated in homozygous npas4l mutants (Marass et al., 2019), suggesting that some populations of mesodermal cells may not have acquired their designated cell fates and remained in a more plastic state. In line with the important role of etsrp in the endothelial cell fate determination, Chestnut et al., 2020 have reported a significant reduction in the cell clusters of endothelial cells and endothelial progenitor cells in homozygous etsrp mutants in a single-cell RNA-seq analysis. Interestingly, they have also shown a remarkable increase in the cluster of lateral plate mesoderm, which points to the possibility that a larger pool of more versatile mesodermal cells remained in the homozygous etsrp mutants. However, it is difficult to deduce why those mesodermal cells with perturbed cell fates would be competent to differentiate into ectopic insulin-expressing β-cells from these transcriptomic data; because the population of these competent mesodermal cells might be very small according to the number of ectopic β-cells that we observed and their single-cell RNA-seq was not performed after β-cell ablation.
Since we could trace the origin of ectopic β-cells back to drl, npas4l, and etsrp lineages, we believe that the versatile lateral plate mesoderm close to the pancreas could contribute to the β-cell regeneration in npas4l mutants or etsrp morphants. Tracing the lineages with early mesodermal, endodermal, and endocrine markers, and sorting these lineage-traced cells could be necessary for a single-cell transcriptomic study to fully understand the cellular status of this small population of versatile cells and further elucidate the underlying molecular mechanisms. Before deciphering these mechanisms, we cannot formally rule out the possibility that the mechanisms of ectopic β-cell formation in npas4l mutants and etsrp morphants could be different.
The mutated gene in the cloche mutant was named npas4l because its encoded protein shares homology with human NPAS4 (Reischauer et al., 2016). Although injecting human NPAS4 mRNA or zebrafish npas4l mRNA into zebrafish cloche mutant embryos at the one-cell stage could rescue the mutants, Npas4 knockout mice are unlikely to share the same severe vascular and hematopoietic defects as zebrafish npas4l mutants because Npas4 knockout mice survive to adulthood (Lin et al., 2008). This discrepancy suggests that other members of the mammalian NPAS protein family or other proteins may be functionally redundant with NPAS4 in vascular and hematopoietic development. Mammalian NPAS4 has been shown to have important cell-autonomous functions in β-cells (Sabatini et al., 2018; Speckmann et al., 2016). In zebrafish, npas4a is the main npas4 paralogue expressed in β-cells (Tarifeño-Saldivia et al., 2017), meaning that it is unlikely the phenotype we identified early in development in npas4l mutants is related to the functions of Npas4 in β-cells. Further studies on NPAS4, related bHLH transcription factors, and ETV2 in mammals should elucidate whether inactivating such factors promotes β-cell formation with or without significantly perturbing the development of blood cells and vessels.
In summary, we have shown that the npas4l mutation and etsrp knockdown each induces ectopic regeneration of β-cells from the mesoderm. Our findings suggest a plasticity potential of mesodermal cells to differentiate into endodermal cells including β-cells (Figure 7). Future studies on the restriction of this plasticity would not only increase our understanding of the gating role of Npas4l and Etsrp in cell fate determination but also help to exploit an alternative source for β-cell regeneration.
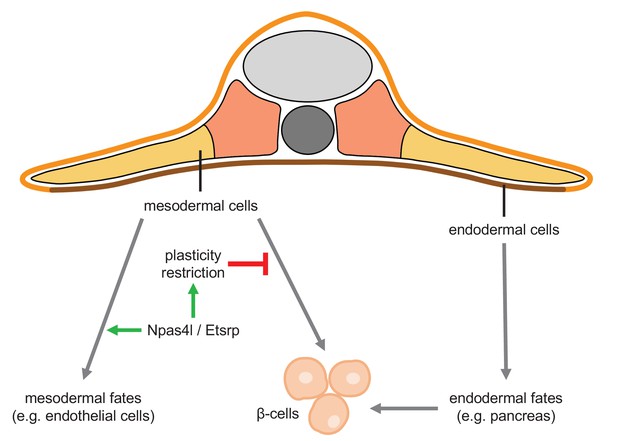
Npas4l/Etsrp restricts the plasticity of the mesoderm.
Mesoderm and endoderm normally follow Waddington’s landscape model to further differentiate into cells with mesodermal fates and endodermal fates, respectively, during development. However, mutating npas4l or knocking down etsrp not only abolishes the endothelial/myeloid specification but also induces plasticity of mesodermal cells to enable their differentiation into β-cells across the germ layer boundary.
Materials and methods
Reagent type (species) or resource | Designation | Source or reference | Identifiers | Additional information |
---|---|---|---|---|
Genetic reagent (Danio rerio) | npas4ls5 | PMID:7588049 | ZFIN: ZDB-ALT-010426–6 | |
Genetic reagent (Danio rerio) | npas4lPt(+36-npas4l-p2a-Gal4-VP16)bns423 | Other | Will be described in detail elsewhere (K.M. and D.Y.R.S., Manuscript in preparation) | |
Genetic reagent (Danio rerio) | Tg(ins:Hsa.HIST1H2BJ-GFP;ins:DsRed)s960 | PMID:25117518 | ZFIN: ZDB-ALT-131001–6 | |
Genetic reagent (Danio rerio) | Tg(ins:FLAG-NTR,cryaa:mCherry)s950 | PMID:22608007 | ZFIN: ZDB-ALT-130930–5 | |
Genetic reagent (Danio rerio) | Tg(ptf1a:EGFP)jh1 | PMID:16258076 | ZFIN: ZDB-ALT-070531–2 | |
Genetic reagent (Danio rerio) | TgBAC(hand2:EGFP)pd24 | PMID:21397850 | ZFIN: ZDB-ALT-110128–40 | |
Genetic reagent (Danio rerio) | TgBAC(neurod1:EGFP)nl1 | PMID:18305245 | ZFIN: ZDB-ALT-080701–1 | |
Genetic reagent (Danio rerio) | TgBAC(pdx1:EGFP)bns13 | PMID:31142539 | ZFIN: ZDB-ALT-191212–6 | |
Genetic reagent (Danio rerio) | Tg(mnx1:GFP)ml2 | PMID:16162647 | ZFIN: ZDB-ALT-051025–4 | |
Genetic reagent (Danio rerio) | Tg(pcsk1:EGFP)KI106 | PMID:27516442 | ZFIN: ZDB-ALT-161115–10 | |
Genetic reagent (Danio rerio) | TgBAC(ascl1b:EGFP-2A-Cre-ERT2)ulg006 | PMID:26329351 | ZFIN: ZDB-ALT-160205–2 | |
Genetic reagent (Danio rerio) | Tg(ins:GCaMP6s,cryaa:RFP) | PMID:28939870 | ZFIN: ZDB-ALT-181221–2 | |
Genetic reagent (Danio rerio) | Tg(−3.5ubb:LOXP-EGFP-LOXP-mCherry)cz1701 | PMID:21138979 | ZFIN: ZDB-ALT-110124–1 | |
Genetic reagent (Danio rerio) | Tg(−6.35drl:Cre-ERT2,cryaa:Venus)cz3333 | PMID:26306682 | ZFIN: ZDB-ALT-160129–4 | |
Genetic reagent (Danio rerio) | Tg(5xUAS:EGFP)nkuasgfp1a | PMID:18202183 | ZFIN: ZDB-ALT-080528–1 | |
Genetic reagent (Danio rerio) | Tg(kdrl:EGFP)s843 | PMID:16251212 | ZFIN: ZDB-ALT-050916–14 | |
Genetic reagent (Danio rerio) | Tg(ubb:LOXP-CFP-LOXP-zgc:114046-mCherry)jh63 | PMID:25773748 | ZFIN: ZDB-ALT-151007–31 | |
Genetic reagent (Danio rerio) | Tg(ins:LOXP-mCherry-LOXP-Hsa.HIST1H2BJ-GFP,cryaa:Cerulean)s934 | PMID:21497092 | ZFIN: ZDB-ALT-111031–2 | |
Genetic reagent (Danio rerio) | Tg(etsrp:iCre;cryaa:Venus)KI114 | This paper | See Materials and methods, section Zebrafish | |
Genetic reagent (Danio rerio) | Tg(UAS:Cre, cryaa:Cerulean)bns382 | This paper | See Materials and methods, section Zebrafish | |
Antibody | Anti-GFP (chicken polyclonal) | Aves Labs | GFP-1020 | (1:500) |
Antibody | Anti-RFP (rabbit polyclonal) | Abcam | ab62341 | (1:500) |
Antibody | Anti-tdTomato (goat polyclonal) | MyBioSource | MBS448092 | (1:500) |
Antibody | Anti-insulin (rabbit polyclonal) | Cambridge Research Biochemicals | Customised | (1:100) |
Antibody | Anti-pan-cadherin (rabbit polyclonal) | Sigma-Aldrich | C3678 | (1:5000) |
Antibody | Anti-islet-1-homeobox (mouse monoclonal) | DSHB | 40.3A4 supernatant | (1:10) |
Recombinant DNA reagent | p5E-MCS (plasmid) | Tol2kit | 228 | |
Recombinant DNA reagent | p5E-etsrp (plasmid) | This paper | −2.3etsrp promoter inserted into p5E-MCS | |
Recombinant DNA reagent | pME-iCre (plasmid) | Other | From Kristen M. Kwan’s lab | |
Recombinant DNA reagent | p3E-polyA (plasmid) | Tol2kit | 302 | |
Recombinant DNA reagent | pDestTol2gY (plasmid) | Other | From Naoki Tsuji | |
Recombinant DNA reagent | −2.3etsrp:iCre,cryaa:Venus (plasmid) | This paper | See Materials and methods, section Zebrafish | |
Sequence-based reagent | −2.3etsrp_FWD | This paper | PCR primer | TATAGGGCGAATTGggtaccTTCAGTAAGCAGACTCCTTCAATCA |
Sequence-based reagent | −2.3etsrp_REV | This paper | PCR primer | AGCTGGAGCTCCAccgcggTTCGGCATACTGCTGTTGGAC |
Sequence-based reagent | Standard control morpholino | Gene Tools | Morpholino | CCTCTTACCTCAGTTACAATTTATA |
Sequence-based reagent | Random control morpholino | Gene Tools | Morpholino | Mixture of many oligo sequences |
Sequence-based reagent | etsrp morpholino MO1 | Gene Tools | Morpholino ZFIN: ZDB-MRPHLNO-060407–2 | TTGGTACATTTCCATATCTTAAAGT |
Sequence-based reagent | etsrp morpholino MO2 | Gene Tools | Morpholino ZFIN: ZDB-MRPHLNO-060407–3 | CACTGAGTCCTTATTTCACTATATC |
Sequence-based reagent | npas4l_ISH_FWD | This paper | PCR primer | ACTCGGGCATCAGGAGGATC |
Sequence-based reagent | npas4l_ISH_REV | This paper | PCR primer | (CCTAATACGACTCACTATAGGG)GACACCAGCATACGACACACAAC |
Sequence-based reagent | drl_ISH_FWD | This paper | PCR primer | ATGAAGAATACAACAAAACCC |
Sequence-based reagent | drl_ISH_REV | This paper | PCR primer | (CCTAATACGACTCACTATAGGG)TGAGAAGCTCTGGCCGC |
Sequence-based reagent | npas4ls5_FWD | PMID:27411634 | PCR primer | TTCCATCTTCTGAATCCTCCA |
Sequence-based reagent | npas4ls5_REV | PMID:27411634 | PCR primer | GGACAGACCCAGATACTCGT |
Sequence-based reagent | npas4ls5_SEQ | This paper | Sequencing primer | TTTCTGCCGTGAATGGATGTG |
Commercial assay or kit | Gateway LR Clonase II Enzyme Mix | Invitrogen | 11791–020 | |
Commercial assay or kit | Phusion High-Fidelity DNA Polymerase | Thermo Scientific | F-530L | |
Commercial assay or kit | In-Fusion HD Cloning Kit | Takara Bio | 639648 | |
Commercial assay or kit | MAXIscript T7/T3 Transcription Kit | Invitrogen | AM1324 | |
Chemical compound, drug | Metronidazole | Sigma-Aldrich | M3761 | |
Chemical compound, drug | 4-Hydroxytamoxifen | Sigma-Aldrich | H7904 | |
Software, algorithm | ImageJ | PMID:22930834 | ||
Software, algorithm | Fiji | PMID:22743772 | ||
Software, algorithm | LAS X Version 3.5.5.19976 | Leica | ||
Software, algorithm | ZEN 3.1 | Zeiss | ||
Software, algorithm | GraphPad Prism 9 | GraphPad Software | ||
Other | DAPI | ThermoFisher Scientific | D1306 | (1 µg/mL) |
Zebrafish
The following previously published mutant and transgenic zebrafish (Danio rerio) lines were used: clocheS5 (Field et al., 2003) as the npas4ls5 mutant, Tg(ins:Hsa.HIST1H2BJ-GFP;ins:DsRed)s960 (Tsuji et al., 2014) abbreviated as Tg(ins:H2BGFP;ins:DsRed), Tg(ins:FLAG-NTR,cryaa:mCherry)s950 (Andersson et al., 2012) abbreviated as Tg(ins:Flag-NTR), Tg(ptf1a:EGFP)jh1 (Godinho et al., 2005), TgBAC(hand2:EGFP)pd24 (Kikuchi et al., 2011), TgBAC(neurod1:EGFP)nl1 (Obholzer et al., 2008), TgBAC(pdx1:EGFP)bns13 (Helker et al., 2019), Tg(mnx1:GFP)ml2 (Flanagan-Steet et al., 2005), Tg(pcsk1:EGFP)KI106 (Lu et al., 2016), TgBAC(ascl1b:EGFP-2A-Cre-ERT2)ulg006 (Ghaye et al., 2015) abbreviated as Tg(ascl1b:EGFP), Tg(ins:GCaMP6s,cryaa:RFP) (Singh et al., 2017) generated by reinjecting the plasmid and abbreviated as Tg(ins:GCaMP6s), Tg(−3.5ubb:LOXP-EGFP-LOXP-mCherry)cz1701 (Mosimann et al., 2011) abbreviated as Tg(ubi:Switch), Tg(−6.35drl:Cre-ERT2,cryaa:Venus)cz3333 (Mosimann et al., 2015) abbreviated as Tg(drl:CreERT2), Tg(5xUAS:EGFP)nkuasgfp1a (Asakawa et al., 2008) abbreviated as Tg(UAS:EGFP), Tg(kdrl:EGFP)s843 (Jin et al., 2005), Tg(ubb:LOXP-CFP-LOXP-zgc:114046-mCherry)jh63 (Wang et al., 2015), and Tg(ins:LOXP-mCherry-LOXP-Hsa.HIST1H2BJ-GFP,cryaa:Cerulean)s934 (Hesselson et al., 2011), which is referred to Tg(ins:mCherry) in Figures 2 and 3 and Video 1 and Tg(ins:CSH) in Figure 6.
The npas4lPt(+36-npas4l-p2a-Gal4-VP16)bns423 line (abbreviated as npas4l:Gal4 or npas4lbns423) was generated using the GeneWeld system (Wierson et al., 2019) and will be described in detail elsewhere (K.M. and D.Y.R.S., Manuscript in preparation).
The Tg(etsrp:iCre;cryaa:Venus)KI114 line, abbreviated as Tg(etsrp:Cre), was generated by the Tol2 transposon system, and the construct was made by MultiSite Gateway Cloning (Invitrogen). The amplicon of the −2.3etsrp promoter was synthesised from zebrafish genomic DNA with a forward primer 5’-TATAGGGCGAATTGggtaccTTCAGTAAGCAGACTCCTTCAATCA-3’ and a reverse primer 5’-AGCTGGAGCTCCAccgcggTTCGGCATACTGCTGTTGGAC-3’ by Phusion High-Fidelity DNA Polymerase (Thermo Scientific) as an insert for In-Fusion Cloning (Takara Bio) with p5E-MCS using restriction sites KpnI and SacII to yield p5E-etsrp. Subsequently, p5E-etsrp, pME-iCre, p3E-polyA, and pDestTol2gY were used for the LR reaction to generate the construct −2.3etsrp:iCre,cryaa:Venus. Expressing iCre (improved Cre recombinase) by an etsrp promoter would cleave the loxP sites of reporters, e.g. ubi:Switch, which allows us to trace the descendants differentiated from an etsrp lineage.
The Tg(UAS:Cre, cryaa:Cerulean)bns382 line, abbreviated as Tg(UAS:Cre), was generated via Tol2-mediated transgenesis by injecting a construct that placed the Cre recombinase coding sequence downstream of five tandem copies of the upstream activation sequence (UAS). The eye-marker cryaa:Cerulean cassette (Hesselson et al., 2009) was inserted downstream of Cre in the reverse orientation using 0.56 kb of the cryaa promoter (Kurita et al., 2003).
Males and females ranging in age from 3 months to 2 years were used for breeding to obtain new offspring for experiments. Individuals were sorted into the control sibling group (npas4l+/+, npas4ls5/+, or npas4lbns423/+) and the npas4l mutant group (npas4ls5/s5 or npas4ls5/bns423) based on the characteristic pericardial oedema and blood-cell deficit. Zebrafish larvae were allocated into different experimental groups based on their phenotypes and genotypes in experiments involving cloche mutants. In morpholino knockdown experiments, zebrafish embryos were randomly assigned to each experimental condition for injection. Experimental procedures were performed on the zebrafish from 10 hpf to 3 dpf before the completion of sex determination and gonad differentiation. All zebrafish, except npas4l mutants and etsrp morphants, appeared healthy and survived to adulthood. The npas4l mutants exhibited pericardial oedema, bell-shaped hearts, and blood deficits, as previously reported (Stainier et al., 1995). The etsrp morphants had similar phenotypes. All studies involving zebrafish were performed in accordance with local guidelines and regulations and approved by regional authorities.
Chemical ablation of β-cells
Request a detailed protocolAs in our previous report (Schulz et al., 2016), we ablated β-cells by incubating the β-cell ablation model Tg(ins:Flag-NTR) zebrafish in E3 medium supplemented with 10 mM metronidazole (MTZ, Sigma-Aldrich), 1% DMSO (VWR), and 0.2 mM 1-phenyl-2-thiourea (Acros Organics) for 24 hr from 1 to 2 dpf.
Microinjection of morpholinos
Request a detailed protocolStandard control morpholino (5’-CCTCTTACCTCAGTTACAATTTATA-3’), random control morpholino, etsrp morpholino MO1 (5’-TTGGTACATTTCCATATCTTAAAGT-3’), and MO2 (5’-CACTGAGTCCTTATTTCACTATATC-3’) (Sumanas and Lin, 2006) were purchased from Gene Tools, LLC. Morpholinos were injected into the one-cell-stage zebrafish embryos at the doses specified in the figure legends.
Lineage tracing by tamoxifen-inducible cre recombinase
Request a detailed protocolTo genetically trace the mesodermal lineage, we treated Tg(ins:Flag-NTR);Tg(ubi:Switch);Tg(drl:CreERT2) zebrafish embryos with 10 μM 4-OHT (Sigma-Aldrich) in E3 medium in 90 mm Petri dishes, with approximately 60 individuals per dish, from 10 to 12 hpf. Upon induction by 4-OHT, cytoplasmic CreERT2 would be translocated to the nucleus to excise the loxP-flanked EGFP to enable mCherry expression in drl-expressing cells and their descendants, indicating a mesodermal lineage.
Sample fixation for immunostaining
Request a detailed protocolBefore fixing the zebrafish larvae, we confirmed the presence of the transgenes by determining the corresponding fluorescent markers and subsequently examined them under a widefield fluorescence microscope LEICA M165 FC (Leica Microsystems). We then euthanised the zebrafish larvae with 250 mg/L tricaine (Sigma-Aldrich) in E3 medium followed by washing in distilled water three times. We fixed the samples in 4% formaldehyde (Sigma-Aldrich) in PBS (ThermoFisher Scientific) at 4°C overnight. After washing away the fixative with PBS three times, we removed the skin and crystallised yolk of the zebrafish larvae by forceps under the microscope to expose the pancreas and mesenchyme for immunostaining.
Immunostaining and confocal imaging
Request a detailed protocolAs in our previous report (Liu et al., 2018), we started immunostaining by incubating the zebrafish samples in blocking solution (0.3% Triton X-100, 4% BSA and 0.02% sodium azide from Sigma-Aldrich in PBS) at room temperature for 1 hr. We then incubated the samples in blocking solution with primary antibodies at 4°C overnight. After removing the primary antibodies, we washed the samples with washing buffer (0.3% Triton X-100 in PBS) eight times at room temperature for 2 hr. Afterwards, we incubated the samples in blocking solution with fluorescent dye-conjugated secondary antibodies and the nuclear counterstain DAPI (ThermoFisher Scientific) if applicable at 4°C overnight. Next, we removed the secondary antibodies and nuclear counterstain and washed the samples with washing buffer eight times at room temperature for 2 hr. The following primary antibodies were used: anti-GFP (1:500, Aves Labs, GFP-1020), anti-RFP (1:500, Abcam, ab62341), anti-tdTomato (1:500, MyBioSource, MBS448092), anti-insulin (1:100, Cambridge Research Biochemicals, customised), anti-pan-cadherin (1:5000, Sigma, C3678), and anti-islet-1-homeobox (1:10, DSHB, 40.3A4 supernatant).
Before confocal imaging, we mounted the stained samples in VECTASHIELD Antifade Mounting Medium (Vector Laboratories) on microscope slides with the pancreas facing the cover slips. We imaged the pancreas and neighbouring mesenchyme of every zebrafish sample that we had mounted with the confocal laser scanning microscopy platform Leica TCS SP8 and LAS X (Leica Microsystems). We analysed the images by Fiji (Schindelin et al., 2012) and classified a β-cell as pancreatic when it was located in the pancreas, as delineated by pan-cadherin, DAPI, or ptf1a:EGFP labelling. The insulin-positive cells outside of the pancreas were defined as ectopic β-cells.
Live calcium imaging of zebrafish β-cells
Request a detailed protocolImaging of pancreatic and ectopic β-cells was performed on 3 dpf Tg(ins:GCaMP6s);Tg(ins:mCherry);Tg(ins:Flag-NTR) npas4l mutants on a ZEISS LSM 980 confocal microscope equipped with a W Plan-Apochromat ×20/1 NA water correction lens and operated by ZEN. The GCaMP6s and mCherry signals from β-cells were simultaneously acquired using the 488 nm and 587 nm laser lines. The GCaMP6s signal was rendered in green, and the mCherry signal was rendered in red. Time series recordings were taken with an in-plane resolution of 1024 × 1024 pixels and a fully open pinhole to maximise light capture. The videos were recorded for 400 cycles, with approximately 2 s acquisition time per frame. Videos were analysed in ImageJ (Schneider et al., 2012).
Whole-mount in situ hybridisation
Request a detailed protocolZebrafish embryos at 10 and 20 dpf were fixed with 4% paraformaldehyde in PBS at 4°C overnight. Whole-mount in situ hybridisation was performed according to the method in a previous report (Thisse and Thisse, 2008). Probes against npas4l and drl were synthesised from transcription templates from PCR using bud-stage zebrafish cDNA, Phusion High-Fidelity DNA Polymerase (Thermo Scientific) and primer pairs 5’-ACTCGGGCATCAGGAGGATC-3’ plus 5’-(CCTAATACGACTCACTATAGGG) GACACCAGCATACGACACACAAC-3’ for npas4l, and 5’-ATGAAGAATACAACAAAACCC-3’ plus 5’-(CCTAATACGACTCACTATAGGG) TGAGAAGCTCTGGCCGC-3’ for drl, respectively. Plasmids carrying probe templates of foxa2, gsc, mixl1, and ascl1b were linearised by corresponding restriction enzymes. T7 (except foxa2 probe with T3) was employed for transcription, and digoxigenin (Roche) was used for labelling. To genotype the npas4ls5 mutants, PCR was performed using gDNA from the imaged samples and primers 5’-TTCCATCTTCTGAATCCTCCA-3’ plus 5’-GGACAGACCCAGATACTCGT-3’ at the conditions previously reported (Reischauer et al., 2016). The PCR products were then sent for sequencing with the following primer 5’-TTTCTGCCGTGAATGGATGTG-3’ (Eurofins Genomics).
Statistical analysis
Request a detailed protocolSimilar experiments were performed at least twice independently. The number of cells in the confocal microscopy images was all quantified manually with the aid of the Multipoint Tool from Fiji (Schindelin et al., 2012). Data were then analysed with Prism (GraphPad). Statistical analyses were carried out by two-tailed t-tests when two groups were analysed and by ANOVA when more than two groups were analysed. We have presented the results as the mean values ± SEM and considered p-values ≤ 0.05 to be statistically significant. The n number represents the number of zebrafish individuals in each group of each experiment.
Data availability
All data generated or analysed during this study are included in the manuscript and source data files.
References
-
Conditional targeted cell ablation in zebrafish: a new tool for regeneration studiesDevelopmental Dynamics 236:1025–1035.https://doi.org/10.1002/dvdy.21100
-
Formation of the digestive system in zebrafish. II. pancreas morphogenesisDevelopmental Biology 261:197–208.https://doi.org/10.1016/S0012-1606(03)00308-7
-
Ectopic pancreas formation in Hes1-knockout mice reveals plasticity of endodermal progenitors of the gut, bile duct, and pancreasJournal of Clinical Investigation 116:1484–1493.https://doi.org/10.1172/JCI27704
-
Chamber identity programs drive early functional partitioning of the heartNature Communications 6:8146.https://doi.org/10.1038/ncomms9146
-
Vesicular glutamate transporter 3 is required for synaptic transmission in zebrafish hair cellsJournal of Neuroscience 28:2110–2118.https://doi.org/10.1523/JNEUROSCI.5230-07.2008
-
Fiji: an open-source platform for biological-image analysisNature Methods 9:676–682.https://doi.org/10.1038/nmeth.2019
-
NIH image to ImageJ: 25 years of image analysisNature Methods 9:671–675.https://doi.org/10.1038/nmeth.2089
-
Critical role for Adenosine receptor A2a in β-cell proliferationMolecular Metabolism 5:1138–1146.https://doi.org/10.1016/j.molmet.2016.09.006
-
Etsrp/Etv2 is directly regulated by Foxc1a/b in the zebrafish angioblastCirculation Research 110:220–229.https://doi.org/10.1161/CIRCRESAHA.111.251298
-
BookThe Strategy of the Genes; a Discussion of Some Aspects of Theoretical BiologyAllen & Unwin.
-
Fate mapping of ptf1a-expressing cells during pancreatic organogenesis and regeneration in zebrafishDevelopmental Dynamics 244:724–735.https://doi.org/10.1002/dvdy.24271
-
Human pancreatic beta-like cells converted from fibroblastsNature Communications 7:10080.https://doi.org/10.1038/ncomms10080
Article and author information
Author details
Funding
Ragnar Söderbergs stiftelse
- Olov Andersson
Max Planck Society
- Didier YR Stainier
Vetenskapsrådet
- Olov Andersson
Novo Nordisk Fonden
- Olov Andersson
Karolinska Institutetvia SRP Diabetes & StratRegen
- Olov Andersson
H2020 European Research Council (772365)
- Olov Andersson
The funders had no role in study design, data collection and interpretation, or the decision to submit the work for publication.
Acknowledgements
We are very thankful to Abdeljabbar El Manira for making equipment available for calcium imaging; Dirk Meyer for plasmids carrying probe templates of foxa2, gsc, mixl1, and ascl1b; Christian Mosimann for sharing the Tg(−6.35drl:Cre-ERT2,cryaa:Venus)cz3333 line and information; and Naoki Tsuji for the pDestTol2gY plasmid.
Ethics
Animal experimentation: This study was performed in accordance with the recommendations of Karolinska Institutet. All of the animals were handled according to approved institutional animal care and in accordance with rules in L150 from the Swedish Board of Agriculture. The protocol was approved by Stockholms Djurförsöksetiska nämnd (Permit Number: N145/15 and 6848-2020).
Copyright
© 2021, Liu et al.
This article is distributed under the terms of the Creative Commons Attribution License, which permits unrestricted use and redistribution provided that the original author and source are credited.
Metrics
-
- 1,717
- views
-
- 163
- downloads
-
- 8
- citations
Views, downloads and citations are aggregated across all versions of this paper published by eLife.
Citations by DOI
-
- 8
- citations for umbrella DOI https://doi.org/10.7554/eLife.65758