Cortex cis-regulatory switches establish scale colour identity and pattern diversity in Heliconius
Abstract
In Heliconius butterflies, wing colour pattern diversity and scale types are controlled by a few genes of large effect that regulate colour pattern switches between morphs and species across a large mimetic radiation. One of these genes, cortex, has been repeatedly associated with colour pattern evolution in butterflies. Here we carried out CRISPR knockouts in multiple Heliconius species and show that cortex is a major determinant of scale cell identity. Chromatin accessibility profiling and introgression scans identified cis-regulatory regions associated with discrete phenotypic switches. CRISPR perturbation of these regions in black hindwing genotypes recreated a yellow bar, revealing their spatially limited activity. In the H. melpomene/timareta lineage, the candidate CRE from yellow-barred phenotype morphs is interrupted by a transposable element, suggesting that cis-regulatory structural variation underlies these mimetic adaptations. Our work shows that cortex functionally controls scale colour fate and that its cis-regulatory regions control a phenotypic switch in a modular and pattern-specific fashion.
eLife digest
Heliconius butterflies have bright patterns on their wings that tell potential predators that they are toxic. As a result, predators learn to avoid eating them. Over time, unrelated species of butterflies have evolved similar patterns to avoid predation through a process known as Müllerian mimicry. Worldwide, there are over 180,000 species of butterflies and moths, most of which have different wing patterns. How do genes create this pattern diversity? And do butterflies use similar genes to create similar wing patterns?
One of the genes involved in creating wing patterns is called cortex. This gene has a large region of DNA around it that does not code for proteins, but instead, controls whether cortex is on or off in different parts of the wing. Changes in this non-coding region can act like switches, turning regions of the wing into different colours and creating complex patterns, but it is unclear how these switches have evolved.
Butterfly wings get their colour from tiny structures called scales, which each have their own unique set of pigments. In Heliconius butterflies, there are three types of scales: yellow/white scales, black scales, and red/orange/brown scales. Livraghi et al. used a DNA editing technique called CRISPR to find out whether the cortex gene affects scale type.
First, Livraghi et al. confirmed that deleting cortex turned black and red scales yellow. Next, they used the same technique to manipulate the non-coding DNA around the cortex gene to see the effect on the wing pattern. This manipulation turned a black-winged butterfly into a butterfly with a yellow wing band, a pattern that occurs naturally in Heliconius butterflies. The next step was to find the mutation responsible for the appearance of yellow wing bands in nature. It turns out that a bit of extra genetic code, derived from so-called ‘jumping genes’, had inserted itself into the non-coding DNA around the cortex gene, ‘flipping’ the switch and leading to the appearance of the yellow scales.
Genetic information contains the instructions to generate shape and form in most organisms. These instructions evolve over millions of years, creating everything from bacteria to blue whales. Butterfly wings are visual evidence of evolution, but the way their genes create new patterns isn't specific to butterflies. Understanding wing patterns can help researchers to learn how genetic switches control diversity across other species too.
Introduction
Butterfly wing pattern diversity provides a window into the ways genetic changes underlie phenotypic variation that is spatially limited to specific parts or regions of the organism (McMillan et al., 2020; Orteu and Jiggins, 2020; Rebeiz et al., 2015). Many of the underlying genetic loci controlling differences in colour patterns have been mapped to homologus ‘hotspots’ across disparate taxa. In some cases, this repeated adaptation has occurred through the alteration of downstream effector genes, such as pigment biosynthetic enzymes with functions clearly related to the trait under selection, for example, the genes tan and ebony that control insect melanin pigmentation (reviewed in Massey and Wittkopp, 2016). In other cases, upstream regulatory genes are important, and these are typically either transcription factors (e.g. optix, MITF, Sox10) or components of signalling pathways such as ligands or receptors (e.g. WntA, MC1R, Agouti). These ‘developmental toolkit genes’ influence pigment cell fate decisions by modulating gene regulatory networks (GRNs) (Kronforst and Papa, 2015; Martin and Courtier-Orgogozo, 2017; Prud'homme et al., 2007), and are commonly characterised by highly conserved functions, with rapid evolutionary change occurring through regulatory fine-tuning of expression patterns. One gene that has been repeatedly implicated in morphological evolution but does not conform to this paradigm is cortex, a gene implicated by mapping approaches in the regulation of adaptive changes in the wing patterning of butterflies and moths.
Cortex is one of four major effect genes that act as switch loci controlling both scale structure and colour patterns in Heliconius butterflies, and has been repeatedly targeted by natural selection to drive differences in pigmentation (Nadeau, 2016; Van Belleghem et al., 2017). Three of the four major effect genes correspond to the prevailing paradigm of highly conserved patterning genes; the signalling ligand WntA (Martin et al., 2012; Mazo-Vargas et al., 2017) and two transcription factors optix (Lewis et al., 2019; Reed et al., 2011; Zhang et al., 2017) and aristaless1 (Westerman et al., 2018). Cortex, on the other hand, is an insect-specific gene showing closest homology to the cdc20/fizzy family of cell cycle regulators (Chu et al., 2001; Nadeau et al., 2016; Pesin and Orr-Weaver, 2007). The lepidopteran orthologue of cortex displays rapid sequence evolution and has acquired novel expression domains that correlate with melanic wing patterns in Heliconius (Nadeau et al., 2016; Saenko et al., 2019). It therefore seems likely that the role of cortex in regulating wing patterns has involved a major shift in function, which sits in contrast to the classic model of regulatory co-option of deeply conserved patterning genes.
The genetic locus containing cortex was originally identified in the genus Heliconius as controlling differences in yellow and white wing patterns in H. melpomene and H. erato (Figure 1a) and the polymorphism in yellow, white, black, and orange elements in H. numata. This was inferred using a combination of association mapping and gene expression data (Joron et al., 2006; Nadeau et al., 2016). The same locus has also been repeatedly implicated in controlling colour pattern variation among divergent Lepidoptera, including the peppered moth Biston betularia and other geometrids, the silkmoth Bombyx mori, and other butterflies such as Junonia coenia, Bicyclus anynana, and Papilio clytia (Beldade et al., 2009; van der Burg et al., 2020; Ito et al., 2016; VanKuren et al., 2019; Van't Hof et al., 2019; Van't Hof et al., 2016). This locus therefore contains one or more genes that have repeatedly been targeted throughout the evolutionary history of the Lepidoptera to generate phenotypic diversity.
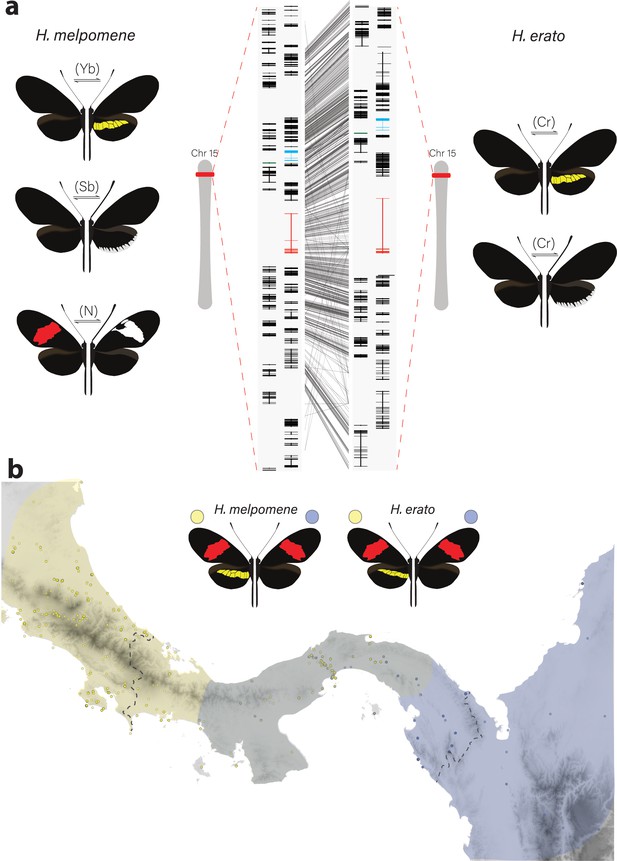
Phenotypic switches of yellow and white colour pattern elements are controlled by homologous loci in Heliconius species.
(a) Homologous loci in both H. erato and H. melpomene are associated with variation in yellow and white patterns between morphs. In H. melpomene, three tightly linked genetic elements located at chromosome 15 have been identified that control variation for the hindwing yellow bar, white margin elements, and forewing band (Yb, Sb, and N, respectively), while in H. erato, variation has been mapped to one element (Cr). The gene cortex is highlighted in red, dome/wash in blue, and dome-trunc in green. Alignments between the two co-mimetic species at the locus is shown (grey lines, 75% alignment identity). Gene models from assemblies of H. melpomene (left) and H. erato (right) are shown for the loci spanning the associated intervals controlling the phenotypic switches highlighted. Horizontal bars indicate exons, vertical bars introns. (b) Focal co-mimetic morphs of H. erato and H. melpomene used in this study, differing in the presence of a hindwing yellow bar, and their ranges across Central America are shown. Yellow: yellow banded morphs, blue: black hindwing morphs, grey: range overlap. Each dot represents a sampled location (data from Rosser et al., 2012). Country borders are indicated by dotted lines.
In Heliconius butterflies, population genomic data suggest that cis-regulatory modules surrounding cortex underlie adaptive variation of yellow and white colour pattern elements (Enciso-Romero et al., 2017; Van Belleghem et al., 2017). These studies predict the existence of modular elements that compartmentalise expression of colour pattern genes across developing wings. However, developmental genes have complex regulatory domains and recent work has suggested that pleiotropy among different enhancers may be more common than is currently appreciated (Lewis et al., 2019; Murugesan et al., 2021; Nagy et al., 2018). Further dissection of the regulatory elements controlling wing pattern variation is thus necessary to assess the relative contribution of pleiotropy versus modularity at colour pattern loci (Lewis and Van Belleghem, 2020).
While fantastically diverse, most of the pattern variation in Heliconius is created by differences in the distribution of just three major scale cell types: Type I (yellow/white), Type II (black), and Type III (red/orange/brown) (Aymone et al., 2013; Gilbert et al., 1987). Each type has a characteristic nanostructure and a fixed complement of pigments. Type I yellow scales contain the ommochrome precursor 3-hydroxykynurenine (3-OHK) (Finkbeiner et al., 2017; Koch, 1993; Reed et al., 2008), whereas Type I white scales lack pigment, and the white colour is the result of the scale cell ultrastructure (i.e. structural white) (Gilbert et al., 1987) (see Figure 9f). Structurally, Type I scales are characterised by the presence of a lamina covering the scale windows and by microribs joining the larger longitudinal ridges. In contrast, Type II scale cells are pigmented with melanin, have larger crossribs and lack a lamina covering the scale windows. Quantitative variation in scale structures between populations (but not within individuals) can cause Type II scales to range from matte black to iridescent blue (Brien et al., 2019; Parnell et al., 2018). Finally, Type III scale cells contain the red ommochrome pigments xanthommatin and dihydroxanthommatin and are characterised by larger spacing between crossribs and ridges.
Here we focus on the role of cortex in specifying these scale types in Heliconius butterflies, an adaptive radiation with over 400 different wing forms in 48 described species (Jiggins, 2017; Lamas, 2004) and where diversity in wing patterns can be directly linked to the selective forces of predation and sexual selection (Brown, 1981; Turner, 1981). Specifically, we combine expression profiling using RNA-seq, ATAC-seq, in situ hybridisation and antibody staining experiments, as well as CRISPR/Cas9 gene knockouts to determine the role that this locus plays in pattern variation of two co-mimetic morphs of H. melpomene and H. erato (Figure 1b). We focus on two mimetic morphs differing specifically in the presence/absence of a yellow hindwing bar, whose phenotypic switch has been mapped to non-coding regions surrounding the gene cortex. We also test its function in diverse patterning morphs, including ones differing in the presence of white margin elements spanning the hindwing, as well as species displaying divergent and complex phenotypes such as the tiger striped silvaniform Heiconius hecale. Despite cortex not following the prevailing paradigm of patterning loci, we demonstrate that the gene plays a fundamental role in pattern variation by modulating a switch from Type I scale cells to Type II and Type III scale cells. Moreover, we show that while the phenotypic effects of cortex extend across the entire fore- and hindwing surface, modular enhancers have evolved in two distantly related Heliconius species that control spatially restricted, pattern-specific expression of cortex. Our findings, coupled with recent functional experiments on other Heliconius patterning loci, are beginning to illuminate how major patterning genes interact during development to determine scale cell fate and drive phenotypic variation across a remarkable adaptive radiation.
Results
The genes cortex and domeless/washout are differentially expressed between colour pattern morphs and between wing sections differing in the presence of the hindwing yellow bar
To identify genes associated with the yellow bar phenotype, we performed differential gene expression (DGE) analysis using developing hindwings sampled from colour pattern morphs in H. erato and H. melpomene differing only in the presence or absence of the hindwing yellow bar (Figures 1b and 2a). In total, we sequenced 18 samples representing three developmental stages (larval, 36 h ± 1.5 h [Day 1 pupae] and 60 h ± 1.5 h [Day 2 pupae]) from two morphs in each of the two species, with hindwings divided into two parts for the pupal stages (Figure 2a). We focused our attention on genes centred on a 47-gene interval on chromosome 15 previously identified as the minimal associated region with yellow band phenotypes by recombination and population genetic association mapping (Nadeau et al., 2016, Supp Table 1; Joron et al., 2006; Moest et al., 2020; Van Belleghem et al., 2017). Both our initial expression analysis and recent analysis of selective sweeps at this locus (Moest et al., 2020) indicate that three genes show differential expression and are likely targets of selection: cortex, domeless (dome), and washout (wash) (Figure 2c). In Heliconius, dome appears to have duplicated in the ancestor of H. erato and H. melpomene, resulting in a full-length copy (referred to here as domeless) and a further copy exhibiting truncations at the C-terminus (domeless-truncated [dome-trunc]) (Figure 1—figure supplements 1–2). Transcriptomic and previous evidence (Lewis et al., 2020) also indicates that dome and wash are transcribed as a single bi-cistronic gene (Figure 1—figure supplements 3–4). Differential expression analysis was thus performed with dome/wash as a single annotation.
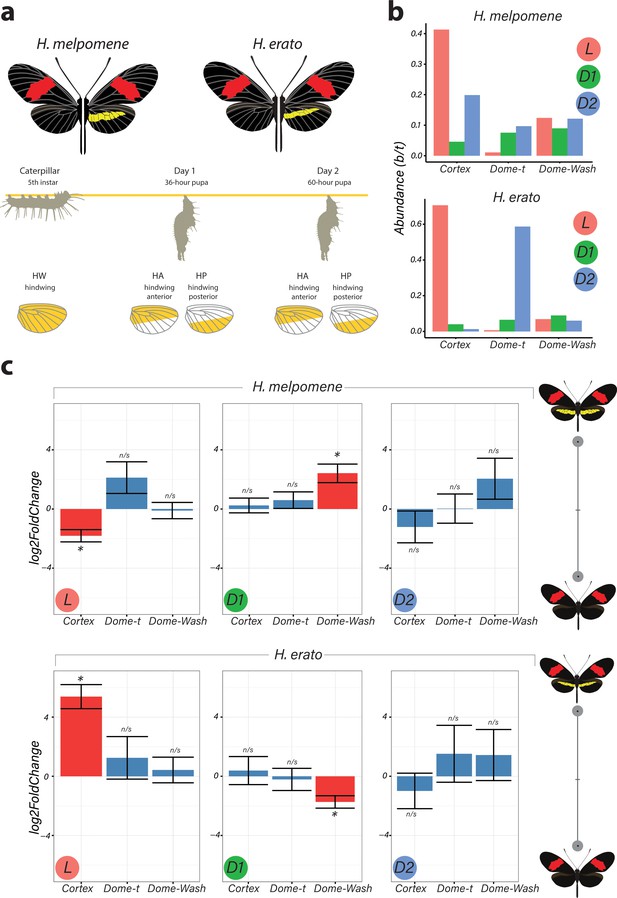
Differential expression of genes at Chromosome 15 implicate cortex as most likely candidate driving yellow bar differences.
(a) Hindwing tissue from co-mimetic morphs of H. melpomene and H. erato were collected at three developmental stages fifth-instar caterpillar, Day 1 Pupae (36hAPF) and Day 2 Pupae (60hAPF). For pupal tissue, hindwing tissue was dissected using the wing vein landmarks shown, corresponding to the future adult position of the hindwing yellow bar (dissection scheme based on Hanly et al., 2019). (b) Relative abundance of transcripts corresponding to the genes cortex, domeless-truncated, domeless/washout throughout developmental stages. (c) Log2FoldChange for the genes cortex, domeless-truncated (dome-t), domeless/washout (dome-wash) across developmental stages. Comparisons are for whole wing discs (Larvae, L) and across wing sections differing in the presence of a yellow bar in pupal wings (D1 and D2; see Figure 2—figure supplement 2: for depiction of contrasts analysed). *Adjusted p<0.05; n/s = not significant. N = 3 for each bar plot.
-
Figure 2—source data 1
RNA-seq samples were genotyped relative to protein-coding WGS SNPs from individuals from the source populations in Panama.
Both SNPs were contained in the protein-coding sequence of the gene Cortex. Individuals from the RNA-seq experiment match the genotype of the source populations.
- https://cdn.elifesciences.org/articles/68549/elife-68549-fig2-data1-v1.docx
-
Figure 2—source data 2
This was repeated for the H. erato samples; here, only one informative protein-coding SNP was found, in the gene parn.
Once again, all individuals match the expected genotype.
- https://cdn.elifesciences.org/articles/68549/elife-68549-fig2-data2-v1.docx
-
Figure 2—source data 3
Primers used for qPCR experiments for housekeeping genes and cortex are shown below.
- https://cdn.elifesciences.org/articles/68549/elife-68549-fig2-data3-v1.docx
-
Figure 2—source data 4
Gene IDs in the H. melpomene Yb locus and their corresponding IDs in the H. erato genome.
- https://cdn.elifesciences.org/articles/68549/elife-68549-fig2-data4-v1.docx
The two species were analysed separately, with both showing only cortex and dome/wash as significantly differentially expressed between morphs among the 47 genes in the candidate region, with cortex differential expression occurring earlier in development. In fifth-instar larvae, cortex is differentially expressed in both species between the two colour pattern morphs, with cortex showing the highest adjusted p-value (Benjamini and Hochberg correction) for any gene in the genome at this stage in H. erato (Figure 2c). Interestingly, cortex transcripts were differentially expressed in opposite directions in the two species, with higher expression in the melanic hindwing morph in H. melpomene and in the yellow banded morph in H. erato. We confirmed this pattern of expression through a SNP association analysis (Figure 2—source data 1–3) and RT-qPCR (Figure 2—figure supplement 1). This pattern is reversed for dome/wash in Day 1 pupae, where a statistically higher proportion of transcripts are detected in H. melpomene rosina (yellow) and in H. erato hydara (melanic) (Figure 2—figure supplements 2–4). No differential expression of these genes was found at Day 2 pupae.
When comparing across hindwing sections differing for the yellow bar phenotype, 22 genes of the associated 47-gene interval were differentially expressed at Day 1 between relevant wing sections in H. melpomene, including cortex and dome/wash (Figure 2—figure supplements 2–4). In contrast, in H. erato Day 1 pupae, only dome/wash was differentially expressed. At Day 2 pupae, there were no differentially expressed genes in either species between relevant wing sections at this locus.
Given the strong support for the involvement of cortex in driving wing patterning differences, we re-analysed its phylogenetic relationship to other cdc20 family genes with more extensive sampling than previous analyses (Nadeau et al., 2016). Our analysis finds strong monophyletic support for cortex as an insect-specific member of the cdc20 family, with no clear cortex homologs found outside of the Neoptera (Figure 2—figure supplement 5). Branch lengths indicate cortex is evolving rapidly within the lineage, despite displaying conserved anaphase promoting complex (APC/C binding motifs, including the C-Box and IR tail Figure 2—figure supplement 6; Chu et al., 2001; Pesin and Orr-Weaver, 2007).
In summary, cortex is the most consistently differentially expressed gene and showed differential expression earlier in development as compared to the other candidate dome/wash. We therefore focused subsequent experiments on cortex, although at this stage we cannot rule out an additional role for dome/wash in yellow pattern specification.
Cortex transcripts localise distally in fifth-instar larval wing discs
Two studies have reported that cortex mRNA expression correlates with melanic patches in two species of Heliconius (Nadeau et al., 2016; Saenko et al., 2019). To further assess this relationship between cortex expression and adult wing patterns, we performed in situ hybridisation on developing wing discs of fifth-instar larvae, where we observed largest cortex transcript abundance, in both the yellow-barred and plain hindwing morphs of H. erato and H. melpomene. Cortex transcripts at this stage localised distally in forewings and hindwings of both species (Figure 3—figure supplement 1). In H. erato demophoon hindwings, expression was strongest at the intervein midline, but extends across vein compartments covering the distal portion of both forewing and hindwing (Figure 3a). By contrast, in H. erato hydara hindwings, cortex transcripts are more strongly localised to the intervein midline forming a sharper intervein expression domain (Figure 3c).
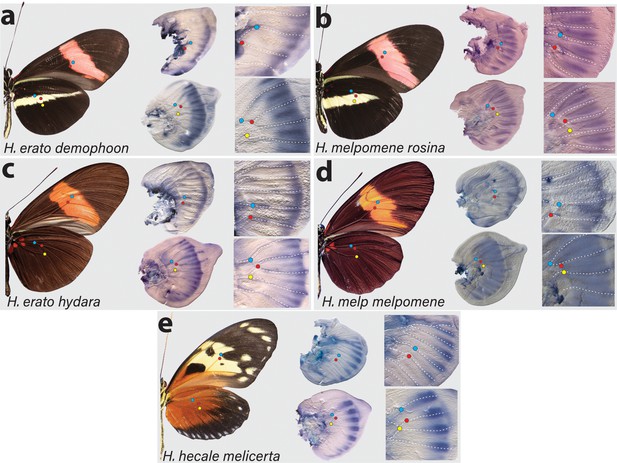
Expression of cortex transcripts in Heliconius melpomene, Heliconius erato, and Heliconius hecale fifth-instar wing discs.
Cortex expression in fifth-instar wing discs is restricted to the distal end of both forewings and hindwings in all species and morphs analysed. In H. erato, expression is strongest at the intervein midline but extends across vein compartments in H. erato demophoon (a), whereas it is more strongly localised to the intervein midline in H. erato hydara (c). In H. melpomene rosina (b), cortex localises in a similar manner to H. erato demophoon, with stronger expression again observed at the intervein midline, whereas expression in H. melpomene melpomene (d) extends more proximally. Expression in H. hecale melicerta (e) is strongest at the distal wing vein margins. Coloured dots represent homologous vein landmarks across the wings.
Expression in H. melpomene rosina is similar to H. erato demophoon at comparable developmental stages, again with stronger expression localised to the intervein midline but extending further proximally than in H. erato demophoon (Figure 3b). In H. melpomene melpomene, hindwing cortex expression extends across most of the hindwing, and does not appear to be restricted to the intervein midline (Figure 3c).
Given that cortex has been implicated in modulating wing patterns in many divergent lepidoptera, we also examined localisation in a Heliconius species displaying distinct patterns: H. hecale melicerta (Figure 3e). Interestingly, in this species, transcripts appear strongest in regions straddling the wing disc veins, with weak intervein expression observed only in the hindwings. Previous data has shown that variation in yellow spots (Hspot) is also controlled by a locus located a chromosome 15 (Huber et al., 2015). Expression in H. hecale melicerta forewings corresponds to melanic regions located in between yellow spots at the wing margins, indicating cortex may be modulating Hspot variation in H. hecale.
Overall, our results suggest a less clear correlation to melanic elements than reported expression patterns (Nadeau et al., 2016; Saenko et al., 2019) where cortex expression in fifth-instar caterpillars is mostly restricted to the distal regions of developing wings, but appears likely to be dynamic across fifth-instar development (Figure 3—figure supplement 1).
Cortex establishes type II and III scale identity in Heliconius butterflies
To assay the function of cortex during wing development, we generated G0 somatic mosaic mutants using CRISPR/Cas9 knock outs. We targeted multiple exons using a combination of different guides and genotyped the resulting mutants through PCR amplification, cloning, and Sanger sequencing (Figure 4—figure supplement 1). Overall KO efficiency was low when compared to similar studies in Heliconius (Concha et al., 2019; Mazo-Vargas et al., 2017), with observed wing phenotype to hatched eggs ratios ranging from 0.3% to 4.8%. Lethality was also high, with hatched to adult ratios ranging from 8.1% to 29.8% (Figure 4—source data 1–2).
Targeting of the cortex gene in H. erato morphs produced patches of ectopic yellow and white scales spanning regions across both forewings and hindwings (Figure 4—figure supplements 2–4). All colour pattern morphs were affected in a similar manner in H. erato. Mutant clones were not restricted to any specific wing region, affecting scales in both proximal and distal portions of wings. The same effect on scale pigmentation was also observed in H. melpomene morphs, with mutant clones affecting both distal and proximal regions in forewings and hindwings (Figure 5a–c). In H. erato hydara, we recovered mutant individuals where clones also spanned the red forewing band (Figure 4b, Figure 4—figure supplement 5). Clones affecting this region caused what appears to be an asymmetric deposition of pigment across the scales, as well as transformation to white, unpigmented scales (Figure 4—figure supplement 5).
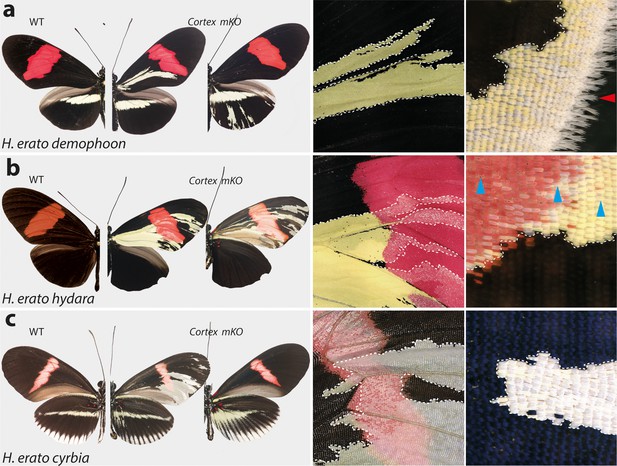
Cortex loss-of-function transforms scale identity across the entire wing surface of Heliconius erato.
Phenotypes of cortex mKO across H. erato morphs reveal a loss of melanic (Type II) and red (Type III) scales and transformation to Type I (yellow or white) scales. Affected regions are not spatially restricted and span both distal and proximal portions of forewings and hindwings. The scale transformation extends to all scale types, including the wing border scales (red arrow head in (a)), and across the red band elements, where mutant scales transform to white, as well as some showing an intermediate phenotype (blue arrow heads in (b)). A positional effect is observed in some morphs, where ectopic Type I scales are either white or yellow depending on their position along the wing (H. erato cyrbia, (c)). Ectopic Type I scales can be induced from both melanic and red scales, switching to either white or yellow depending on wing position and morph. Boundaries between Wild-type (WT) to mutant scales are highlighted (dotted white line).
-
Figure 4—source data 1
CRISPR experiments and guides used per species/morph.
- https://cdn.elifesciences.org/articles/68549/elife-68549-fig4-data1-v1.xlsx
-
Figure 4—source data 2
Sequences for guides yielding successful phenotypes and associated genotyping primers.
- https://cdn.elifesciences.org/articles/68549/elife-68549-fig4-data2-v1.xlsx
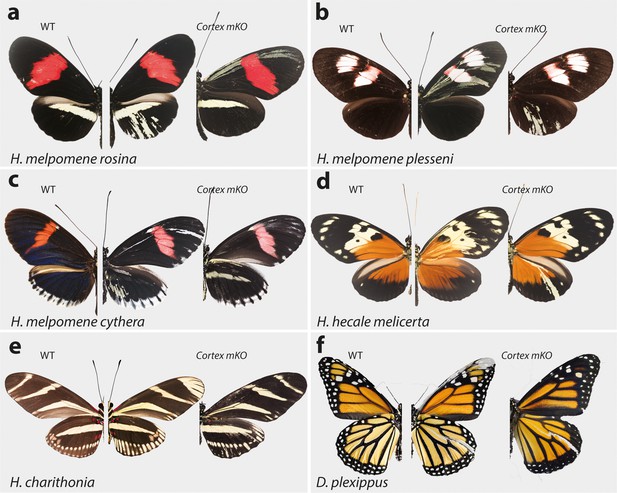
Cortex function is conserved across Heliconius and Nymphalids Phenotypes of cortex mKO across H. melpomene colour pattern morphs.
(a–c) reveal cortex has a conserved function in switching scale cell fates, as in H. erato. This function is also conserved in outgroups to H. melpomene and H. erato (H. hecale melicerta and H. charithonia respectively (d–e)) as well as in distantly diverged nymphalids (D. plexippus (f)). Left; wild-type, middle and right; cortex mKO.
As this locus has been associated with differences in white hindwing margin phenotypes (Jiggins and McMillan, 1997; Figure 1b), we also targeted cortex in mimetic morphs that display the same phenotype in the two species, H. erato cyrbia and H. melpomene cythera. Mutant scales in these colour pattern morphs were also localised across both wing surfaces, with both white and yellow ectopic scales (Figures 4c and 5c). Both the white and blue colouration in these co-mimics is structurally derived, indicating that cortex loss-of-function phenotype also affects the scale ultrastructure. Furthermore, we observed a positional effect, where ectopic scales in the forewing and anterior compartment of the hindwing shifted to yellow, and posterior hindwing scales became white (Figure 4c, Figure 4—figure supplement 5d). This positional effect likely reflects differential uptake of the yellow pigment 3-OHK across the wing surface, which may be related to cryptic differential expression of the yellow-white switch aristaless-1 (Reed et al., 2008; Westerman et al., 2018).
To further test the conservation of cortex function across the Heliconius radiation, as well as nymphalids as a whole, we knocked out cortex in H. charithonia and H. hecale melicerta, outgroups to H. erato and H. melpomene, respectively, and Danaus plexippus as an outgroup to all Heliconiini. Again, ectopic yellow and white scales appeared throughout the wing surface in all species, suggesting a conserved function with respect to scale development among butterflies (Figure 5d–f, Figure 5—figure supplements 1–5).
In summary, cortex mKOs appear to not be restricted to any specific wing pattern elements and instead affect regions across the surface of both forewings and hindwings. Mutant scales are always Type I scales, with differing pigmentation (3-OHK, yellow) or structural colouration (white) depending on morph and wing position. The high rate of mosaicism combined with high mortality rates suggests cortex is likely developmentally lethal. Mutant clones also appear aggregated, suggesting the cortex mKO may affect early phases of cell division or communication during development and produce a growth disadvantage or differential adhesion relative to WT cells that result in grouping effects.
Nuclear localisation of Cortex protein extends across the wing surface in pupal wings
The cortex mRNA expression patterns in larval imaginal disks suggest a dynamic progression in the distal regions, and in a few cases (Figure 3; Nadeau et al., 2016; Saenko et al., 2019), a correlation with melanic patterns whose polymorphisms associate with genetic variation at the cortex locus itself. We thus initially hypothesised that like for the WntA mimicry gene (Martin et al., 2012; Mazo-Vargas et al., 2017, Concha et al., 2019), the larval expression domains of cortex would delimit the wing territories where it is playing an active role in colour patterning. However, our CRISPR based loss-of-function experiments challenge that hypothesis because in all the morphs that we assayed, we found mutant scales across the wing surface.
This led us to re-examine our model and consider that post-larval stages of cortex expression could reconcile the observation of scale phenotypes across the entire wing, rather than in limited areas of the wing patterns. To test this hypothesis, we developed a Cortex polyclonal antibody and found nuclear expression across the epithelium of H. erato demophoon pupal hindwings without restriction to specific pattern elements (Figure 6). In fifth-instar larvae, Cortex protein was detected in a similar pattern to mRNA, with expression visible at the intervein midline of developing wings (Figure 6a). Cortex was then detected across the entire wing surface from 24 hr after pupal formation (a.p.f), until 80 hr a.p.f in our time series (Figure 6b–d, Figure 6—figure supplement 1). Localisation remained nuclear throughout development and appears equal in intensity across hindwing colour pattern elements (Figure 6e).
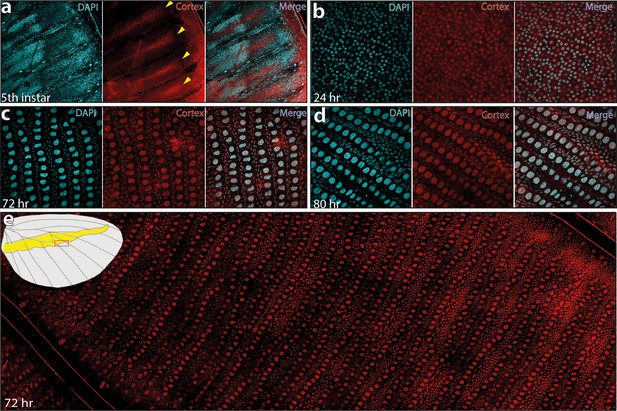
Cortex protein localises across the wings in H. erato demophoon.
Antibody stainings reveal Cortex protein is localised at the distal intervein midline in fifth-instar wing discs (yellow arrowheads) (a). At 24 hr APF, the protein is detected across the wing and localised strongly to the cell nuclei (b). This localisation continues at 72 hr APF(c) and 80 APF (d). In each panel, leftmost insert shows nuclei stained with DAPI (magenta), middle insert with Cortex antibody detected with a secondary alexa-fluor 555 conjugated antibody (red), and right insert shows both channels merged into a composite image. No appreciable difference in localisation is detected across presumptive pattern elements at 72 hr APF (e). The magnified portion of the imaged wing is indicated in the wing cartoon in the top left corner of ( e).
Modular cis-regulatory elements drive the evolution of the mimetic yellow bar
Given the broad effect observed for cortex across both wing surfaces, we next tested whether specific expression might be under the control of pattern-specific cis-regulatory elements (CREs). In order to look for potential CREs, we performed an Assay for Transposase-Accessible Chromatin using sequencing (ATAC-seq) in fifth-instar hindwings of both co-mimetic morphs differing in the presence of the yellow bar in H. erato and H. melpomene (Figure 7—source data 1). We observed many accessible chromatin ‘peaks’ surrounding cortex (Figure 7—figure supplement 1), each of which could represent a potentially active CRE. To narrow down candidate peaks that could be regulating cortex in a pattern-specific manner, we overlayed association intervals with the ATAC-seq signals, which indicate evolved regions between populations of H. melpomene differing in the hindwing yellow bar phenotype. Specifically, we applied the phylogenetic weighting strategy Twisst (topology weighting by iterative sampling of subtrees; Martin and Van Belleghem, 2017) to identify shared or conserved genomic intervals between sets of H. melpomene populations (obtained from Moest et al., 2020) with similar phenotypes around cortex. This method identified a strong signal of association ~8 kb downstream of the annotated cortex stop codon, that overlapped with a clear ATAC-seq peak (Figure 7a,b, Figure 7—figure supplement 1).
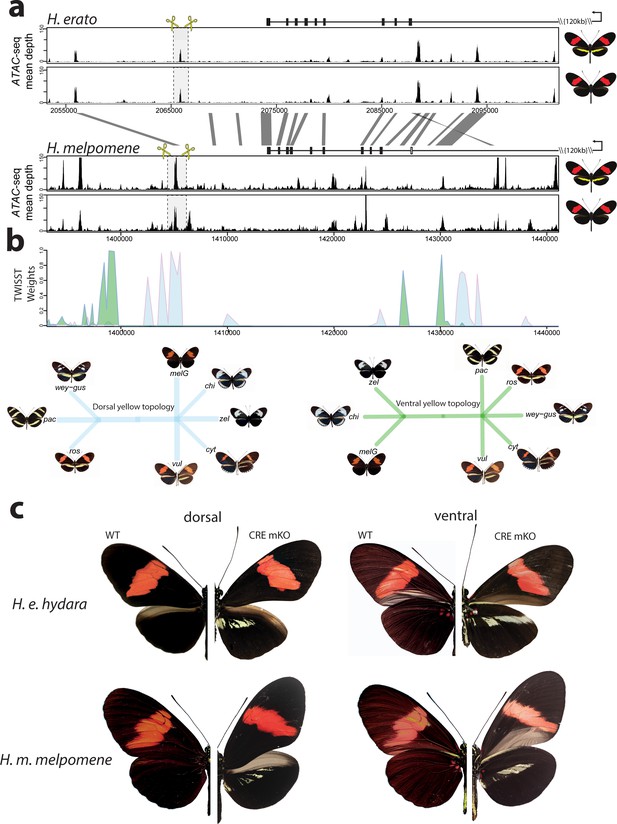
Modular CREs control the presence of the yellow band in Heliconius melpomene and Heliconius erato.
(a) Chromatin accessibility as measured by mean sequence depth for ATAC-seq traces in H. erato (top) and H. melpomene (bottom) in fifth-instar caterpillar hindwings in yellow banded and black morphs. The gene model for cortex is shown above the traces (black rectangles are coding exons, white rectangle non-coding exon, lines are introns, direction of transcription is indicated by an arrow). The transcription start site is around 120 kb from the first coding exon of cortex. Positions of sgRNAs used for peak excision are shown (yellow scissors). Regions with >75% sequence identity between H. melpomene and H. erato are indicated by grey lines. (b) Twisst analysis results showing high genetic association for the presence of a yellow bar in H. melpomene populations with a ventral (ventral topology) or dorsal (dorsal topology) yellow bar. Abbreviations for twisst morphs: wey-gus = H. cydno weymeri-gustavi, chi = H. cydno chioneus, zel = H. cydno zelinde, pac = H. pachinus, ros = H. melpomene rosina, melG = H. melpomene melpomene French Guiana, vul = H. melpomene vulcanus, cyt = H. melpomene cythera. (c) Cortex loss-of-function at the yellow bar CREs affect scales only in the presumptive yellow bar region. CRE KO affects both dorsal (left) and ventral (right) hindwings.
-
Figure 7—source data 1
List of ATAC-seq samples used in this study, and corresponding accession numbers.
- https://cdn.elifesciences.org/articles/68549/elife-68549-fig7-data1-v1.docx
We next sought to knock out this CRE, by designing a pair of sgRNA guides flanking the ATAC-seq signal. We reasoned that since cortex controlled the switch to melanic scales across the entire wing, knocking-out an enhancer in the melanic morph (H. melpomene melpomene), or in F1 hybrids between H. melpomene rosina and H. melpomene melpomene, should result in the appearance of yellow scales in a yellow bar-like pattern. Indeed, upon KO of this CRE we recovered mKOs consistent with a modular enhancer driving cortex expression in a yellow bar-specific pattern, with no clones exhibiting yellow scales extending out of the region that forms the yellow bar (Figure 7c, Figure 7—figure supplement 2).
To test whether the same element was driving the evolution of the yellow bar phenotype in the co-mimetic morph of H. erato, we first targeted the homologous peak, which shares both 95% sequence identity with H. melpomene, as well as the presence of an accessible chromatin mark (Figure 7a). While none of our CRISPR trials resulted in a visible phenotype at this locus (number of injected adults eclosed = 36), we did observe the presence of a further accessible region ~10 kb 3’ of the H. melpomene conserved CRE. We reasoned that a different but positionally close peak could be driving the yellow bar phenotype in H. erato. Remarkably, targeting of this CRE resulted in a yellow bar phenotype in the melanic H. erato hydara, with no clones containing yellow scales extending beyond the region that forms the yellow bar (Figure 7c, Figure 7—figure supplement 3). Deletions at each of the loci were confirmed by PCR amplification, cloning, and Sanger sequencing (Figure 7—figure supplement 4).
Finally, to confirm that the CREs were interacting with the cortex promoter, we took advantage of a previously published set of Hi-C samples in H. erato populations (Lewis et al., 2019), to check for enhancer/promoter interactions through the implementation of virtual 4C plots. For both CREs, we found a statistically significant interaction between CRE and promoter, indicating the observed effect is likely due to the CRE interacting with the cortex promoter, and not a different gene at the locus (Figure 7—figure supplement 5).
Transposable element insertions are associated with the yellow bar phenotype in geographically distinct H. melpomene populations
Given that we were able to induce a yellow bar phenotype by the deletion of a modular CRE, we next asked whether natural populations with this phenotype might also show a similar deletion. To test for the presence of deletions at the candidate CRE, we used extensive publicly available whole-genome re-sequence data for geographically isolated populations differing in the presence of the hindwing yellow bar (Darragh et al., 2019; Enciso-Romero et al., 2017; Kozak et al., 2018; Martin et al., 2019; Van Belleghem et al., 2017). In total, we assayed 16 geographically isolated subspecies across central and south America and looked for signature coverage drops at the targeted ATAC-seq peak, which could be indicative of deletions (Chan et al., 2010; Kemppainen et al., 2021; Figure 8—source data 1). We observed a characteristic drop in coverage at the targeted CRE in all H. melpomene and H. timareta morphs exhibiting a yellow bar phenotype, while no drop was detected in morphs with a melanic hindwing (Figure 8a). Given this characteristic signature associated with the presence of a yellow bar in the sequencing data, we next genotyped across the putative deletion using Sanger sequencing in H. melpomene rosina and H. melpomene melpomene individuals. Surprisingly, we found two transposable element (TE) insertions in H. melpomene rosina with a Helitron-like TE found spanning the CRE peak, suggesting that the coverage drop is instead due to an insertion of repetitive sequence, rather than a deletion. Enhancer disruption is therefore likely caused by TE sequence in yellow bar morphs (Liu et al., 2019). We next assayed three other yellow-barred morphs (H. melpomene bellula, H. melpomene amaryllis, and H. timareta tristero) and found the same TE signatures in all three populations (Figure 8—figure supplement 2), suggesting the TE insertions are likely shared through introgression. No similar signature of reduced coverage was observed in co-mimetic morphs of H. erato, suggesting that sequence divergence is responsible for the evolution of the yellow bar CRE in this species (Figure 8—figure supplement 2).
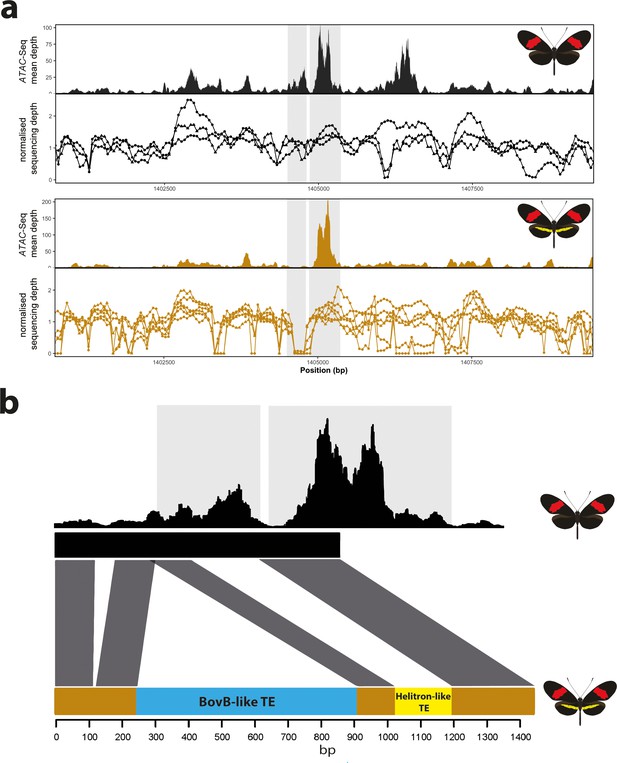
Coverage drop indicative of deletions in yellow-barred populations of H. melpomene.
(a) Mean sequence depth for ATAC-seq traces for the excised CRE are shown above normalised depth in sequencing coverage for populations of H.melpomene (circles) and H. timareta (triangles) differing for the presence of a yellow bar. Yellow-barred populations display a drop in coverage for both ATAC signal and sequencing depth at a position corresponding to a portion of the targeted CRE (highlighted by the grey lines). Morphs analysed for melanic hindwing H. melpomene and H. timareta: H. m. maletti, H. m. melpomene, H. t. florencia. Morphs analysed for yellow-barred H. melpomene and H. timareta: H. m. bellula, H. m. amaryllis, H. m. rosina, H. m. burchelli, H t. thelxione, H. t. tristero. (b) Sanger sequencing of target regions in H. m. melpomene and H. m. rosina reveals an insertion of two TE elements surrounding the yellow bar CRE. A larger BovB-like element of 690 bp (blue) and a smaller 163 bp Helitron-like element (yellow) are present in the H. m. rosina sequences, but not the H. m. melpomene sequences. Base pair positions of the consensus Sanger sequencing traces are shown below.
-
Figure 8—source data 1
List of individuals used in coverage depth analysis, and corresponding accession numbers.
- https://cdn.elifesciences.org/articles/68549/elife-68549-fig8-data1-v1.docx
-
Figure 8—source data 2
Consensus sequences recovered from Sanger sequencing across the H. melpomene/timareta CRE.
The BovB-like TE element is indicated in blue; The Helitron-like fragment in orange. Both are absent from the H. melpomene melpomene sequence.
- https://cdn.elifesciences.org/articles/68549/elife-68549-fig8-data2-v1.docx
Cortex coding KO causes partial homeotic shifts in scale structure
Previous studies have shown an association between scale ultrastructure and pigmentation in Heliconius butterflies (Concha et al., 2019; Gilbert et al., 1987; Janssen et al., 2001; Zhang et al., 2017). In particular, it has been reported that perturbation by wounding transforms both the pigment content and structure of scales in a tightly coupled way (Janssen et al., 2001). We thus asked whether ectopic yellow/white scales generated through cortex knockout were accompanied by structural transformations using scanning electron microscopy (SEM) in the same way as ectopic colour scales generated through wounding or WntA knockouts (Janssen et al., 2001; Concha et al., 2019). To account for known positional effects on scale structure, we compared wild-type and mutant scales from homologous locations across the wing surface.
We observed ultrastructural shifts that are consistent with partial homeosis in cortex mutant scales in both H. melpomene and H. erato (Figure 9, Figure 9—figure supplement 1). In all cases where a yellow or white (Type I) clone was present in a region that would otherwise be black or blue (Type II) in the wild type, the ultrastructure of the scale was notably different. Wild-type blue and black scales have crossribs at a spacing of ~0.6 µm, lack lamina between ridges and crossribs, and have no prominent microribs, while both wild-type and mutant Type I scales have no prominent crossribs, lamina that fills the spaces between the microribs and ridges, and prominent microribs at a spacing of ~0.2 µm (Figure 9a–d, Figure 9—source data 1–2). A consistent difference between all Type I scales (mutant and wild type) is the presence of a lamina covering the inter-ridge space (Figure 9f). These ultrastructural shifts suggest that the perturbation of cortex affects scale fate decision, not only shifting pigmentation type, but also scale morphology.
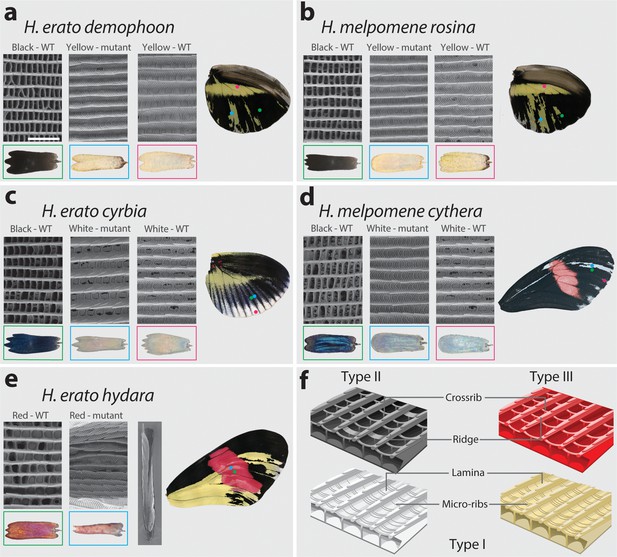
SEM reveals structural changes induced by cortex KO.
Ultrastructural morphology of H. erato demophoon (a) and H. melpomene rosina (b) hindwing scales for wild-type (WT) black, mutant yellow and wild-type yellow scales. Light micrographs of each scale are shown below the representative SEM images. Scale morphologies are also presented for morphs displaying shifts to white scales in H. erato cyrbia (c) and H. melpomene cythera (d). Structural changes in the red scales of H. erato hydara (e) are accompanied by scale deformations, resulting in a curled appearance. Cartoon depiction of scale ultrastructure illustrating differences between scale types (f). Type I scales are characterised by the presence of a lamina covering the scale windows and by microribs joining the longitudinal ridges. Type II scale cells display larger crossribs and lack a lamina covering the scale windows. Type III scale cells and are characterised by larger spacing between crossribs and ridges. Scale bar in (a) = 3 µm.
-
Figure 9—source data 1
Pairwise Wilcox test adjusted p-values for quantitative measures of scale structures and features in H.melpomene.
- https://cdn.elifesciences.org/articles/68549/elife-68549-fig9-data1-v1.docx
-
Figure 9—source data 2
Pairwise Wilcox test adjusted p-values for quantitative measures of scale structures and features in H.erato.
- https://cdn.elifesciences.org/articles/68549/elife-68549-fig9-data2-v1.docx
Red scales (Type III) that are within a coding KO clone take on an aberrant structure and pigmentation. Scales were frequently found to be curled up laterally, and while ommochrome pigment is sometimes visibly deposited in the scale, it is granular in appearance rather than diffuse throughout the scale (Figure 9e, Figure 9—figure supplement 2). These ‘granular’ pigment accumulations could not be observed as a distinct structure by SEM, suggesting that they are under the surface of the scale. As with wild-type and mutant Type I scales, prominent microribs can also be observed on these rolled scales, but due to the topological deformity of these scales, it was not possible to take accurate measurements.
Discussion
Cortex is a key scale cell specification gene
The genetic locus containing the gene cortex represents a remarkable case of convergent evolution, where repeated and independent mutations surrounding the gene are associated with shifts in scale pigmentation state in at least nine divergent species of Lepidoptera (Beldade et al., 2009; van der Burg et al., 2020; Nadeau et al., 2016; Van Belleghem et al., 2017; VanKuren et al., 2019; Van't Hof et al., 2019; Van't Hof et al., 2016). While these studies have linked putative regulatory variation around cortex to the evolution of wing patterns, its precise effect on scale cell identity and pigmentation has remained speculative until now. Here, we demonstrate that cortex is a causative gene that specifies melanic and red (Type II and Type III) scale cell identity in Heliconius and acts by influencing both downstream pigmentation pathways and scale cell ultrastructure. We also show that cortex is under the control of modular enhancers that appear to control the switch between mimetic yellow bar phenotypes in both H. melpomene and H. erato. Our combination of expression studies and functional knockouts demonstrate that this gene acts as a key scale cell specification switch across the wing surface of Heliconius butterflies, and thus has the potential to generate much broader pattern variation than previously described patterning genes.
While we have shown that cortex is a key scale cell specification gene, it remains unclear how a gene with homology to the fizzy/cdc20 family of cell cycle regulators acts to modulate scale identity. In Drosophila, Fizzy proteins are known to regulate APC/C activity through the degradation of cyclins, leading to the arrest of mitosis (Raff et al., 2002). In particular, fizzy-related (fzr) induces a switch from the mitotic cycle to the endocycle, allowing the development of polyploid follicle cells in Drosophila ovaries (Schaeffer et al., 2004; Shcherbata et al., 2004). Similarly, cortex has been shown to downregulate cyclins during Drosophila female meiosis, through its interaction with the APC/C (Pesin and Orr-Weaver, 2007; Swan and Schüpbach, 2007). Immunostainings show that Cortex protein localises to the nucleus in Heliconius pupal wings, suggesting a possible interaction with the APC/C in butterfly scale building cells. Ploidy levels in Lepidoptera scale cells have been shown to correlate with pigmentation state, where increased ploidy and scale size lead to darker scales (Cho and Nijhout, 2013; Iwata and Otaki, 2016). cortex may thus be modulating ploidy levels by inducing endoreplication cycles in developing scale cells. However, we currently have no direct evidence for a causal relationship between ploidy state and pigmentation output, and a mechanistic understanding of this relationship and any role cortex may be playing in modulating ploidy levels will require future investigation.
A curious result reported from our RNA-seq dataset is that differential expression appears to occur in opposite directions between the two co-mimetic morphs. While this could represent some difference in the precise role of cortex between H. melpomene and H. erato, the pattern may also be explained by the limited sampling during fifth-instar development in both species. The dynamic expression observed during fifth-instar wing development suggests that levels of cortex expression may be changing in a precise and variable manner of short periods of development. A more precise time series across fifth-instar and early pupal development may thus be needed to reveal the precise difference in cellular function of cortex between these species.
The mimetic yellow bar phenotype switch is controlled by the evolution of modular enhancers
In H.melpomene, we were able to narrow down a clear peak of association with the presence of accessible chromatin marks and showed that KO of this region results in the appearance of a yellow bar phenotype in black hindwing morphs (Figure 7). Interestingly, when targeting the homologous peak in H. erato, we failed to recover any type of phenotype, but were able to induce the appearance of a yellow bar through the targeting of an adjacent peak, not present in the H. melpomene datasets, indicating that an independently evolved CRE is driving this phenotype in H. erato.
These results, coupled with the coding KOs, suggests that the CREs are enhancers that are able to drive cortex expression in a yellow bar specific manner. It is therefore puzzling that both the in situ hybridisation and antibody experiments failed to recover an association between Cortex localisation and the yellow bar phenotype. One possibility is that, because cortex is expressed throughout the wing, the differences in cortex expression that drive the pattern difference are either highly discrete in time and therefore hard to observe, or are the consequence of subtle changes in concentration that we could not detect with immunofluorescence. Moreover, cortex is known to have complex patterns of alternative splicing (Nadeau et al., 2016), suggesting that perhaps both our polyclonal antibody and in situ probes lack the specificity to detect localisation of specific alternatively spliced variants. This lack of a conspicuous link between expression and function is a puzzling result that will require further investigation in future. The ideal experiments would utilise the identified enhancers as enhancer traps, to show they are able to drive expression in a pattern-specific manner, as well as perform knock-in experiments in the reciprocal co-mimetic morph, to show that these regions are sufficient to drive the phenotypic switches.
In H. melpomene, we found a clear association between the absence of an accessible chromatin peak in yellow-barred populations with a characteristic drop in coverage over the same region, that overlaps with both the targeted CRISPR and association intervals. The mapped profiles show that this drop in coverage is explained by phenotype, rather than geography, in contrast to other adjacent regions. Upon further investigation, we found a large 690 bp TE insertion 5’ of the peak of interest as well as a Helitron-like sequence overlapping the peak in H. melpomene rosina. This raises the interesting possibility that this portion of the enhancer might contain the binding sites necessary to drive cortex in a yellow bar specific manner, and that recurrent TE insertions across this region are driving the evolution of this phenotype in H. melpomene populations. We also note that this insertion is observed in mimetic morphs of a different species, H. timareta, with which H. melpomene has previously been described to share regulatory regions at other patterning loci via adaptive introgression (Morris et al., 2019; Wallbank et al., 2016). Thus, adaptive introgression of this region and its structural variants is likely facilitating mimicry in this system (Heliconius Genome Consortium et al., 2012).
Heliconius wing patterning is controlled by interactions among major patterning genes
Functional knockouts now exist for all the four major loci known to drive pigmentation differences in Heliconius (Mazo-Vargas et al., 2017; Westerman et al., 2018; Zhang et al., 2017). These loci represent the major switching points in the GRNs that are ultimately responsible for determining scale cell identity. This work underscores the importance of two patterning loci, cortex and WntA, as master regulators of scale cell identity. Both are upregulated early in wing development and have broad effects on pattern variation (Concha et al., 2019; Nadeau et al., 2016). The signalling molecule WntA modulates forewing band shape in Heliconius by delineating boundaries around patterns elements, and is expressed in close association with future pattern elements (Concha et al., 2019; Martin et al., 2012). Unlike cortex mutants, WntA KOs shift scale cell identity to all three cell types (I, II, and III), depending on genetic background. Thus, WntA acts as a spatial patterning signal inducing or inhibiting colour in specific wing elements, in contrast to cortex, which acts as an ‘on-off’ switch across all scales on the butterfly wing.
Interestingly, cortex knockouts lead to shifts in scale fate irrespective of WntA expression. This suggests either that cortex is required as an inductive signal to allow WntA to signal further melanisation, or that two, independent ways to melanise a scale are available to the developing wing. The latter hypothesis is supported by certain H. erato colour pattern WntA mutants, where even in putatively cortex-positive regions, scales are able to shift to Type I in the absence of WntA alone (Concha et al., 2019). This indicates that while under certain conditions cortex is sufficient to induce the development of black scales, WntA is also required as a further signal for melanisation in some genetic backgrounds. Under this scenario, colour pattern morphs may be responding epistatically to different WntA/cortex alleles present in their respective genetic backgrounds. This is also consistent with genetic evidence for epistasis between these two loci seen in crossing experiments, whereby the yellow bar in H. erato favorinus results from an interaction between the Cortex and WntA loci (Mallet, 1989).
Under a simple model (Figure 10), cortex is one of the earliest regulators and sets scale differentiation to a specific pathway switches between Type I (yellow/white) and Type II/III (black/red) scales. Thus, we can envision a differentiating presumptive scale cell (PSC) receiving a Cortex input as becoming Type II/III competent, with complete Type III differentiation occurring in the presence of optix expression (Zhang et al., 2017). This is consistent with our data, which shows cortex is also required as a signal for Type III (red) scales to properly develop. Several cortex mutant individuals had clones across red pattern elements and failed to properly develop red pigment. The development of red scales in Heliconius butterflies is also dependent on expression of the transcription factor optix during mid-pupal development (Lewis et al., 2019; Reed et al., 2011; Zhang et al., 2017). Therefore, cortex expression is required for either downstream signalling to optix or to induce a permissive scale morphology for the synthesis and deposition of red pigment in future scales. Cortex is thus necessary for the induction of Type III scale cells but insufficient for their proper development.
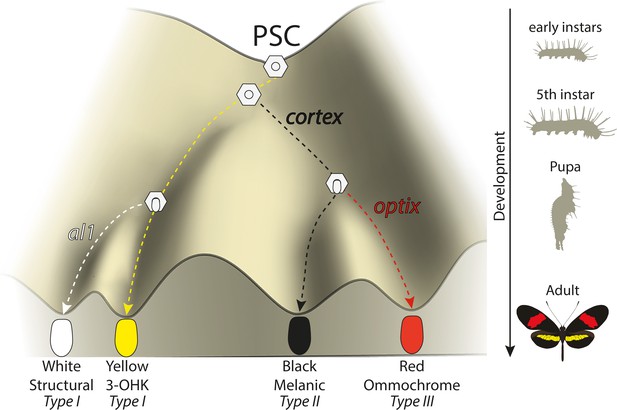
Expression of key genes affect scale fate decisions and influence downstream pigmentation state.
During early-instar development, wing disc cells differentiate into presumptive scale cells (PSCs). Throughout fifth-instar growth, PSCs express key scale cell specification genes such as cortex, which induce differentiation into Type II (optix−) scales or Type III (optix+) scales. In the absence of cortex, scale cells differentiate into Type I scales, which differ in pigmentation state based on 3-OHK synthesis controlled by aristaless1 expression. Model based on the epigenetic landscape (Waddington) and by observations made by Gilbert et al., 1987.
Conversely, a PSC lacking a Cortex input differentiates into a Type I scale, whose pigmentation state depends on the presence of the transcription factor aristaless1 (al1), where al1 is responsible for inducing a switch from yellow to white scales in Heliconius by affecting the deposition of the yellow pigment 3-OHK (Westerman et al., 2018). The uptake of 3-OHK from the haemolymph occurs very late in wing development, right before the adult ecloses (Reed et al., 2008). Our cortex mKOs revealed a shift to both yellow and white scales, with their appearance being positionally dependent; more distally located scales generally switch to white, while more proximal scales become yellow. This pigmentation state is likely controlled by differences in al1 expression varying between wing sections in different ways across morphs.
However, the switch induced by Cortex under this model is likely not a simple binary toggle, and is perhaps dependent on a given protein threshold or heterochrony in expression rather than presence/absence, as we find that Cortex protein also localises to the presumptive yellow bar in developing pupal wings. Moreover, the RNA-seq data presented suggests other linked genes may also be playing a role in controlling pattern switches between Heliconius morphs. In particular, we report the presence of a bi-cistronic transcript containing the ORFs of the genes dome/wash, which are differentially expressed during early pupal wing development. While a precise role for dome/wash in wing patterning remains to be demonstrated, it raises the possibility that multiple linked genes co-operate during Heliconius wing development to drive pattern diversity. It is noteworthy that in the locally polymorphic H. numata, all wing pattern variation is controlled by inversions surrounding cortex and dome/wash, both of which are also differentially expressed in H. numata (Saenko et al., 2019). This raises the interesting possibility that evolution has favoured the interaction of multiple genes at the locus that have since become locked into a supergene in H. numata.
Conclusions
The utilisation of ‘hotspots’ in evolution has become a recurring theme of evolutionary biology, with several examples in which independent mutations surrounding the same gene have driven adaptive evolution (e.g Pitx1, Scute) (Stern and Orgogozo, 2009). One proposed facilitator of such hotspots is through the action of genes acting as ‘input-output’ modules, whereby complex spatio-temporal information is translated into a co-ordinated cell differentiation program, in a simple switch-like manner. One prediction of the nature of such genes would be a switch-like behaviour such as that observed for cortex in this study, as well as the presence of a complex modular cis-regulatory architecture surrounding the gene that is able to integrate the complex upstream positional information into the switch-like output. A conserved feature of the cortex locus in Lepidoptera is the presence of large intergenic regions surrounding the gene, with evidence these may be acting as modular cis-regulatory switches in Heliconius (Enciso-Romero et al., 2017; Van Belleghem et al., 2017), fitting the predicted structure of input-output genes. Unlike canonical input-output loci however, cortex expression appears not to be restricted to any particular colour pattern element in any given species/morph, and yet is capable of producing a switch-like output (Type I vs Type II/III scales). Furthermore, our work shows that two independent CREs in H. melpomene and H. erato evolved to control the presence/absence of a yellow hindwing bar. However, it is still unclear how cortex mechanistically affects pigmentation differences, and given its widespread usage throughout Lepidoptera, it is of general interest to understand its role in driving scale pigmentation.
Materials and methods
Butterfly husbandry
Request a detailed protocolHeliconius butterflies were collected in the tropical forests of Panama and Ecuador. Adults were provided with an artificial diet of pollen/glucose solution supplemented with flowers of Psiguria, Lantana, and/or Psychotria alata according to availability. Females were provided with Passiflora plants for egg laying (P. menispermifolia for H. melpomene, P. biflora for H. erato and H. charithonia, and P. vitifolia for H. hecale). Eggs were collected daily, and caterpillars reared on fresh shoots of P. williamsi (melpomene), P. biflora (erato and charithonia), and P. vitifolia for H. hecale. Late fifth (final) instar caterpillars were separated into individual pots in a temperature-monitored room for RNA-seq experiments, where they were closely observed for the purpose of accurate developmental staging.
Phylogenetic analysis of domeless and cortex
Request a detailed protocolTo identify orthologues of dome across the Lepidoptera, we performed tBLASTn searches using the previously annotated H. melpomene Hmel2 (Hm) and H.erato demophoon V1 (Hed) dome sequences against the genomes of Operophtera brumata V1 (Ob), Trichoplusia ni Hi5.VO2 (Tn), Bombyx mori ASM15162v1 (Bm), Manduca sexta 1.0 (Ms), Plodia interpunctella V1 (Pi), Amyeolis transitella V1 (At), Phoebis sennae V1.1 (Ps), Bicyclus anynana V1.2 (Ba), Danaus plexippus V3 (Dp), Dryas iulia helico3 (Di), Agraulis vanillae helico3 (Av), Heliconius erato lativitta V1 (Hel) genomes found on Lepbase (Challi et al., 2016). As a trichopteran outgroup, we used a recently published Pacbio assembly of Stenopsyche tienmushanensis (St) (Luo et al., 2018). Recovered amino acid translations were aligned using clustal omega (Madeira et al., 2019). The resulting alignments were used to produce a phylogenetic tree using PhyML (Guindon et al., 2010), based on a best fit model using AIC criterion (selected model was JTT + G + I+F). The tree was visualised and re-rooted to the Trichopteran outgroup using FigTree.
To confirm cortex as a cdc20 gene, we retrieved full-length protein homologs from TBLASTN searches and used them to generate a curated alignment with MAFFT/Guidance2 with a column threshold of 0.5. Guidance2 is an alignment reliability method that parses aligned residues while also maximising tree robustness (Sela et al., 2015), thus ruling out biases introduced from paralog-specific domains. We then constructed a maximum-likelihood tree using W-IQ-TREE with the ‘Auto’ function to find a best-fit model of substitution.
Tissue sampling and RNA-seq
Request a detailed protocolH. melpomene rosina and H. erato demophoon butterflies were collected around Gamboa, Panama; H. melpomene melpomene and H. erato hydara butterflies were collected around Puerto Lara, Darien, Panama. Methodology for sample preparation and sequencing was performed as previously descri Hanly et al., 2019. The datasets generated and/or analysed during the current study are available in the SRA repository (PRJNA552081). Reads from each species were aligned to the respective genome assemblies Hmel2 (Davey et al., 2016) and Herato_demophoon_v1 (Van Belleghem et al., 2017), using Hisat2 with default parameters (Kim et al., 2019). The genomes and annotations used are publicly available at http://www.lepbase.org. Reads were counted with HTSeq-count in union mode (Anders et al., 2015) and statistical analysis performed with the R package DESeq2 (Love et al., 2014). Comparisons for larvae were for whole hindwings, grouping samples by pattern form. Samples for pupal stages included wings that were dissected into anterior and posterior compartment as in Hanly et al., 2019, and were analysed in DESeq2 using the GLM:
(Compartments: anterior hindwing [HA], posterior hindwing [HPo]). H. melpomene and H. erato were analysed separately; homology between genes was determined by reciprocal BLAST. The fold-changes and adjusted p-values given in Figure 2 reflect the primary contrast, showing the effect of pattern form given the effect of compartment. Read counts were determined for whole hindwings at all stages.
RT-qPCR
Request a detailed protocolThe expression level of cortex in larval hindwings was further analysed by qPCR in H. e. demophoon and H. e. hydara. Three individuals were used for each morph. Each individual was an independent replicate (i.e. no pooling of samples). RNA was extracted from the hindwing tissue of larva using Trizol followed by DNase-treatment. An mRNA enrichment was performed using the Dynabeads mRNA purification kit (Thermo Fisher). The mRNA was then converted to cDNA by reverse transcription using the iScript cDNA synthesis kit (Bio-Rad). All reactions had a final cDNA concentration of 2 ng µl−1 and a primer concentration of 400 nM. The RT-qPCR was carried out using Brilliant III Ultra-fast SYBR green qPCR master mix (Agilent Technologies), on a AriaMx Real-time PCR system (Agilent Technologies) according to manufacturer’s instructions. The PCR programme consisted of 95°C for 2 min followed by 40 cycles of 95°C for 5 s, 58°C for 30 s, and 70°C for 30 s. qPCR experiments were performed using three biological replicates, three technical replicates, and a no template control. Expression levels were normalised using the geometric mean of three housekeeping genes, eF1α, rpL3 and polyABP that have previously been validated for Heliconius numata (Piron Prunier et al., 2016). The relative expression levels were analysed using the R = 2−ΔΔCt method (Livak and Schmittgen, 2001). Primer specificity was confirmed using melting curve analysis and the PCR products were checked on a 2% (w/v) agarose gel. The primer efficiency of each gene was calculated using the standard curve given by a 10-fold serial dilution of cDNA (1, 10−1, 10−2, 10−3, 10−4) and regression coefficient (R2) values.
In situ hybridisations
Request a detailed protocolFifth-instar larval wing disks and whole mount in situ hybridisations were performed following a published procedure (Martin and Reed, 2014) and imaged using a Leica S4E microscope (Leica Microsystems). Riboprobe synthesis was performed using the following primers from a fifth-instar wing disc cDNA library extracted from H. melpomene:
Forward primer 5’-CCCGAGATTCTTTCAGCGAAAC-3’ and Reverse primer 5’- ACCGCTCCAACACCAAGAAG-3’. Templates for riboprobes were designed by attaching a T7 promoter through PCR and performing a DIG labelled transcription reaction (Roche). The same H. melpomene probe was used in all in situ hybridisation experiments. The resulting probe spanned from Exon two to Exon 7 and was 841 bp long.
Immunohistochemistry and image analysis
Request a detailed protocolPupal wings were dissected around 60–70 hr post-pupation in PBS and fixed at room temperature with fix buffer (400 µl 4% paraformaldehyde, 600 µl PBS 2 mM EGTA) for 30 min. Subsequent washes were done in wash buffer (0.1% Triton-X 100 in PBS) before blocking the wings at 4°C in block buffer (0.05 g bovine serum slbumin, 10 ml PBS 0.1% Triton-X 100). Wings were then incubated in primary antibodies against Cortex (1:200, monoclonal rabbit anti-Cortex) at 4°C overnight, washed, and added in secondary antibody (1:500, donkey anti-rabbit lgG, AlexaFlour 555, ThermoFisher Scientific A-31572). Before mounting, wings were incubated in DAPI with 50% glycerol overnight and finally transferred to mounting medium (60% glycerol/40% PBS 2 mM EGTA) for imaging. Z-stacked two-channelled confocal images were acquired using a Zeiss Cell Observer Spinning Disk Confocal microscope.
CRISPR/Cas9 genome editing
Request a detailed protocolGuide RNAs were designed corresponding to GGN20NGG sites located within the cortex coding region and across putative CREs using the program Geneious (Kearse et al., 2012). To increase target specificity, guides were checked against an alignment of both H. melpomene and H. erato re-sequence data at the scaffolds containing the cortex gene (Moest et al., 2020; Van Belleghem et al., 2017), and selected based on sequence conservation across populations. Based on these criteria, each individual guide was checked against the corresponding genome for off-target effects, using the default Geneious algorithm. Guide RNAs with high conservation and low off-target scores were then synthesised following the protocol by Bassett and Liu, 2014. Injections were performed following procedures described in Mazo-Vargas et al., 2017, within 1–4 hr of egg laying. Several combinations of guide RNAs for separate exons at different concentrations were used for different injection experiments. For H. charithonia, we used the H. erato-specific guides, and for H. hecale, we used the H. melpomene guides.
Genotyping
Request a detailed protocolDNA was extracted from mutant leg tissue and amplified using oligonucleotides flanking the sgRNAs target region. PCR amplicons were column purified, subcloned into the pGEM-T Easy Vector System (Promega), and sequenced on an ABI 3730 sequencer.
ATAC-seq
Request a detailed protocolH. melpomene rosina and H. erato demophoon butterflies were collected around Gamboa, Panama; H. melpomene melpomene and H. erato hydara butterflies were collected around Puerto Lara, Darien, Panama. Caterpillars of each species were reared on their respective host plants and allowed to grow until the wandering stage at fifth instar. Live larvae were placed on ice for 1–2 min and then pinned and dissected in 1× ice cold PBS. The colour of the imaginal discs, as well as the length of the lacunae, gradually change throughout the larva’s final day of development, so that they can be used to confirm the staging inferred from pre-dissection cues (Reed et al., 2008). All larvae used for this project were stage 3.5 or older. ATAC-seq protocol was based on previously described methodology (Lewis and Reed, 2019) and edited as follows. The imaginal discs were removed and suspended whole in 350 µl of sucrose solution (250 mM d-sucrose, 10 mM Tris–HCl, 1 mM. MgCl2, 1× protease inhibitors [Roche]) inside labelled 2 ml dounce homogenisers (Sigma-Aldrich) for nuclear extraction. Imaginal discs corresponding to the left and right hindwings were pooled. After homogenising the tissue on ice, the resulting cloudy solution was centrifuged at 1000 rcf for 7 min at 4 °C. The pellet was then resuspended in 150 µl of cold lysis buffer (10 mM Tris–HCl, 10 mM NaCl, 3 mM MgCl2, 0.1% IGEPAL CA-630 [Sigma-Aldrich], 1× protease inhibitors) to burst the cell membranes and release nuclei into the solution. Samples were then checked under a microscope with a counting chamber following each nuclear extraction, to confirm nuclei dissociation and state and to assess the concentration of nuclei in the sample. Finally, based on these observations, a calculation to assess number of nuclei, and therefore DNA, to be exposed to the transposase was performed. This number was fixed on 400,000 nuclei, which is the number of nuclei with ~0.4 Gb genomes (H. erato genome size) required to obtain the amount of DNA for which ATAC-seq is optimised (Buenrostro et al., 2013). For H. melpomene, this number was 500,333, since the genome size of H. melpomene is 0.275 Gb. (Buenrostro et al., 2013). For quality control, a 15 µl aliquot of nuclear suspension was stained with trypan blue, placed on a hemocytometer and imaged at 64×. After confirmation of adequate nuclear quality and assessment of nuclear concentration, a subsample of the volume corresponding to 400,000 nuclei (H. erato) and 500,333 (H. melpomene) was aliquoted, pelleted 1000 rcf for 7 min at 4°C and immediately resuspended in a transposition mix, containing Tn5 in a transposition buffer. The transposition reaction was incubated at 37°C for exactly 30 min. A PCR Minelute Purification Kit (Qiagen) was used to interrupt the tagmentation and purify the resulting tagged fragments, which were amplified using custom-made Nextera primers and a NEBNext High-fidelity 2× PCR Master Mix (New England Labs). Library amplification was completed between the STRI laboratory facilities in Naos (Panama) and Cambridge (UK). The amplified libraries were sequenced as 37 bp paired-end fragments with NextSeq 500 Illumina technology at the Sequencing and Genomics Facility of the University of Puerto Rico.
Topology weighting by iterative sampling of subtrees (Twisst) analysis
Request a detailed protocolWe applied the phylogenetic weighting strategy Twisst (topology weighting by iterative sampling of subtrees; Martin and Van Belleghem, 2017) to identify shared or conserved genomic intervals between sets of Heliconius melpomene and Heliconius cydno populations with similar phenotypes around the cortex gene locus on chromosome 15. Given a tree and a set of pre-defined groups Twisst determines a weighting for each possible topology describing the relationship of groups or phylogenetic hypothesis. Similar to Enciso-Romero et al., 2017 we evaluated the support for two alternative phylogenetic hypotheses using genomic data obtained from Moest et al., 2020. Hypothesis one tested for monophyly of samples that have a dorsal yellow hindwing bar. This comparison included the geographic colour patterns morphs with a dorsal hindwing bar H. m. rosina, H. c. weymeri weymeri, H. c. weymeri gustavi and H. pachinus versus the all-black dorsal hindwing morphs H. m. vulcanus, H. m. melpomene (French Guiana), H. m. cythera, H. c. chioneus and H. c. zelinde. Hypothesis two tested for monophyly of samples that have a ventral yellow hindwing bar versus an all-black ventral hindwing. This comparison included the geographic colour patterns morphs with a ventral hindwing bar H. m. rosina, H. m. vulcanus, H. m. cythera, H. c. weymeri weymeri, H. c. weymeri gustavi and H. pachinus versus the all-black ventral hindwing morphs H. m. melpomene (French Guiana), H. c. chioneus and H. c. zelinde. Maximum-likelihood trees were built from sliding windows of 50 SNPs with a step size of 20 SNPs using PhyML v3.0 (Guindon et al., 2010) and tools available at https://github.com/simonhmartin/twisst (Martin, 2020).Only windows were considered that had at least 10 sites for which each population had at least 50% of its samples genotyped. Twisst was run with a fixed number of 1000 subsampling iterations.
Hi-C and virtual 4C plots
Request a detailed protocolAnalysis of chromatin contacts between distal cis-regulatory loci and the cortex promoter region was performed as previously described (Lewis et al., 2019 PNAS, Lewis and Van Belleghem, 2020). In brief, Hi-C data produced from day three pupal H. e. lativitta wings was used to generate an empirical expected distribution and read counts for Hi-C contacts between 5 kb windows centred on the distal CRE and cortex promoter were used to determine the observed contacts between loci. Fisher’s exact test was then performed to determine significance of the observed contacts relative to those expected from the background model. Virtual Hi-C signal plots were generated using a custom python script (Ray et al., 2019).
Coverage depth analysis and TE genotyping
Request a detailed protocolHigh-depth whole-genome sequences of 16 H. melpomene, H. timareta, and H. erato subspecies were obtained from the European Nucleotide Archive, accession numbers can be found in Figure 8—source data 1. To assess structural variation putatively affecting the yellow phenotype, reads were mapped to the reference genomes of subspecies that lacked the yellow bar stored in the genome browser Lepbase, ‘hmel2.5’ for H. melpomene and H. timareta, and ‘Heliconius_erato_lativitta_v1’ for H. erato (Challi et al., 2016) with BWA mem (Li and Durbin, 2009). Median sequencing depths across the scaffold containing cortex were computed for all individuals (n = 79) in 50 bp sliding windows and a mapping quality threshold of 30 with the package Mosdepth (Pedersen and Quinlan, 2018). Window median depths were normalised by dividing them by the mean depth for the full scaffold per individual. We then averaged the normalised median depths of all individuals per subspecies, to visualise deviations from the mean sequencing depth across the region in geographically widespread subspecies with and without the yellow bar. We then genotyped across the putative H. melpomene deletion using the primers employed for CRISPR genotyping (See Figure 4—source data 2). The products were then cloned into the pGEM-T Easy Vector System (Promega) and sequenced them on an ABI 3730 sequencer from both directions using T7 forward and M13 reverse primers. Sequencing was performed from three separate colonies, and a consensus sequence was created based on an alignment of the three replicates from populations of H. m. melpomene and H. m. rosina.
SEM imaging
Request a detailed protocolIndividual scales from wild-type and mutant regions of interest were collected by brushing the surface of the wing with an eyelash tool, then dusted onto an SEM stub with double-sided carbon tape. Stubs were then colour imaged under the Keyence VHX-5000 microscope for registration of scale type. Samples were sputter-coated with one 12.5 nm layer of gold for improving sample conductivity. SEM images were acquired on a FEI Teneo LV SEM, using secondary electrons and an Everhart-Thornley detector using a beam energy of 2.00 kV, beam current of 25 pA, and a 10 μs dwell time. Individual images were stitched using the Maps 3.10 software (ThermoFisher Scientific).
Morphometrics analysis
Request a detailed protocolMorphometric measurements of scale widths and ridge distances were carried out on between 10 and 20 scales of each type, using a custom semi-automated R pipeline that derives ultrastructural parameters from large SEM images (Day et al., 2019). Briefly, ridge spacing was assessed by Fourier transforming intensity traces of the ridges acquired from the FIJI software (Schindelin et al., 2012). Scale width was directly measured in FIJI by manually tracing a line, orthogonal to the ridges, at the section of maximal width.
Data availability
ATAC-Seq sequencing data have been deposited under ENA BioProject (accession number PRJEB43672). Raw data on morphometrics and high magnification images of mutants are available on Dryad (doi:https://doi.org/10.5061/dryad.8gtht76m0).
-
Dryad Digital RepositoryCortex cis-regulatory switches establish scale colour identity and pattern diversity in Heliconius.https://doi.org/10.5061/dryad.8gtht76m0
-
ENAID PRJEB43672. Cortex cis-regulatory switches establish scale colour identity and pattern diversity in Heliconius.
-
NCBI BioProjectID SRAPRJNA552081. Wing RNAseq from Heliconius melpomene, Heliconius erato, Agraulis vanillae Raw sequence reads.
References
-
The biology of Heliconius and related generaAnnual Review of Entomology 26:427–457.https://doi.org/10.1146/annurev.en.26.010181.002235
-
Development of polyploidy of scale-building cells in the wings of Manduca sextaArthropod Structure & Development 42:37–46.https://doi.org/10.1016/j.asd.2012.09.003
-
Male pheromone composition depends on larval but not adult diet in Heliconius melpomeneEcological Entomology 44:397–405.https://doi.org/10.1111/een.12716
-
Ultraviolet and yellow reflectance but not fluorescence is important for visual discrimination of conspecifics by Heliconius eratoThe Journal of Experimental Biology 220:1267–1276.https://doi.org/10.1242/jeb.153593
-
BookCorrelations of Ultrastructure and Pigmentation Suggest How Genes Control Development of Wing Scales of Heliconius ButterfliesThe Journal of research on the Lepidoptera.
-
Correlations between scale structure and pigmentation in butterfly wingsEvolution and Development 3:415–423.https://doi.org/10.1046/j.1525-142X.2001.01046.x
-
BookThe Ecology and Evolution of Heliconius ButterfliesOxford University Press.https://doi.org/10.1093/acprof:oso/9780199566570.001.0001
-
The genetic basis of an adaptive radiation: warning colour in two Heliconius speciesProceedings of the Royal Society of London. Series B: Biological Sciences 264:1167–1175.https://doi.org/10.1098/rspb.1997.0161
-
Genetic population structure constrains local adaptation in sticklebacksMolecular Ecology 30:1946–1961.https://doi.org/10.1111/mec.15808
-
Graph-based genome alignment and genotyping with HISAT2 and HISAT-genotypeNature Biotechnology 37:907–915.https://doi.org/10.1038/s41587-019-0201-4
-
BookAtlas of Neotropical Lepidoptera: Checklist Pt. 4a Hesperioidea-PapilionoideaScientific Pub.
-
Genome-Wide regulatory adaptation shapes Population-Level genomic landscapes in HeliconiusMolecular Biology and Evolution 36:159–173.https://doi.org/10.1093/molbev/msy209
-
Mechanisms of change: a Population-Based perspective on the roles of modularity and pleiotropy in diversificationFrontiers in Ecology and Evolution 8:261.https://doi.org/10.3389/fevo.2020.00261
-
The EMBL-EBI search and sequence analysis tools APIs in 2019Nucleic Acids Research 47:W636–W641.https://doi.org/10.1093/nar/gkz268
-
BookMorphological Evolution Repeatedly Caused by Mutations in Signaling Ligand GenesIn: Sekimura T, Nijhout H. F, editors. Diversity and Evolution of Butterfly Wing Patterns: An Integrative Approach. Springer. pp. 59–87.
-
The genetic basis of pigmentation differences within and between Drosophila speciesCurrent Topics in Developmental Biology 119:27–61.https://doi.org/10.1016/bs.ctdb.2016.03.004
-
From patterning genes to process: unraveling the gene regulatory networks that pattern Heliconius wingsFrontiers in Ecology and Evolution 8:221.https://doi.org/10.3389/fevo.2020.00221
-
Genes controlling mimetic colour pattern variation in butterfliesCurrent Opinion in Insect Science 17:24–31.https://doi.org/10.1016/j.cois.2016.05.013
-
The genomics of coloration provides insights into adaptive evolutionNature Reviews Genetics 21:461–475.https://doi.org/10.1038/s41576-020-0234-z
-
Wing scale ultrastructure underlying convergent and divergent iridescent colours in mimetic Heliconius butterfliesJournal of the Royal Society Interface 15:20170948.https://doi.org/10.1098/rsif.2017.0948
-
The roles of fzy/Cdc20 and fzr/Cdh1 in regulating the destruction of cyclin B in space and timeJournal of Cell Biology 157:1139–1149.https://doi.org/10.1083/jcb.200203035
-
Unraveling the tangled skein: the evolution of transcriptional regulatory networks in developmentAnnual Review of Genomics and Human Genetics 16:103–131.https://doi.org/10.1146/annurev-genom-091212-153423
-
Testing historical explanations for gradients in species richness in Heliconiine butterflies of tropical americaBiological Journal of the Linnean Society 105:479–497.https://doi.org/10.1111/j.1095-8312.2011.01814.x
-
Fiji: an open-source platform for biological-image analysisNature Methods 9:676–682.https://doi.org/10.1038/nmeth.2019
-
Adaptation and evolution in Heliconius: a defense of NeoDarwinismAnnual Review of Ecology and Systematics 12:99–121.https://doi.org/10.1146/annurev.es.12.110181.000531
-
Complex modular architecture around a simple toolkit of wing pattern genesNature Ecology & Evolution 1:1–12.https://doi.org/10.1038/s41559-016-0052
-
Genetic convergence of industrial melanism in three geometrid mothsBiology Letters 15:20190582.https://doi.org/10.1098/rsbl.2019.0582
-
Butterfly mimicry polymorphisms highlight phylogenetic limits of gene reuse in the evolution of diverse adaptationsMolecular Biology and Evolution 36:2842–2853.https://doi.org/10.1093/molbev/msz194
Article and author information
Author details
Funding
Biotechnology and Biological Sciences Research Council (BB/R007500/1)
- Luca Livraghi
- Eva SM van der Heijden
- Ian A Warren
- Charlotte Wright
- Chris D Jiggins
National Science Foundation (IOS-1656553 and IOS-1755329)
- Joseph J Hanly
- Ling Sheng Loh
- Anna Ren
- Arnaud Martin
Puerto Rico Science, Technology and Research Trust (#2020-00142)
- Steven M Van Bellghem
- Riccardo Papa
Smithsonian Institution (NSF IOS-1656389)
- Carolina Concha
- W Owen McMillan
National Science Foundation (NSF IOS 1656389)
- Riccardo Papa
The funders had no role in study design, data collection and interpretation, or the decision to submit the work for publication.
Acknowledgements
We thank Oscar Paneso, Elizabeth Evans, Rachel Crisp, and Cruz Batista, for technical support with rearing of butterflies and CRISPR larvae, and to Markus Möest, and Tim Thurman for assistance with butterfly collection. We are also grateful to Krzysztof ‘Chris’ Kozak and Chi Yun for thoughtful discussions and feedback on the manuscript. We thank the GW Nanofabrication and Imaging Center for enabling SEM, and in particular Christine Brantner, Chris Day, and Anastas Popratiloff for their technical assistance.
Copyright
© 2021, Livraghi et al.
This article is distributed under the terms of the Creative Commons Attribution License, which permits unrestricted use and redistribution provided that the original author and source are credited.
Metrics
-
- 4,205
- views
-
- 522
- downloads
-
- 53
- citations
Views, downloads and citations are aggregated across all versions of this paper published by eLife.
Citations by DOI
-
- 53
- citations for umbrella DOI https://doi.org/10.7554/eLife.68549