Ciliary Hedgehog signaling regulates cell survival to build the facial midline
Abstract
Craniofacial defects are among the most common phenotypes caused by ciliopathies, yet the developmental and molecular etiology of these defects is poorly understood. We investigated multiple mouse models of human ciliopathies (including Tctn2, Cc2d2a, and Tmem231 mutants) and discovered that each displays hypotelorism, a narrowing of the midface. As early in development as the end of gastrulation, Tctn2 mutants displayed reduced activation of the Hedgehog (HH) pathway in the prechordal plate, the head organizer. This prechordal plate defect preceded a reduction of HH pathway activation and Shh expression in the adjacent neurectoderm. Concomitant with the reduction of HH pathway activity, Tctn2 mutants exhibited increased cell death in the neurectoderm and facial ectoderm, culminating in a collapse of the facial midline. Enhancing HH signaling by decreasing the gene dosage of a negative regulator of the pathway, Ptch1, decreased cell death and rescued the midface defect in both Tctn2 and Cc2d2a mutants. These results reveal that ciliary HH signaling mediates communication between the prechordal plate and the neurectoderm to provide cellular survival cues essential for development of the facial midline.
Introduction
Primary cilia are microtubule-based organelles present on diverse vertebrate cell types and critical for development. Primary cilia function as specialized cellular signaling organelles that coordinate multiple signaling pathways, including the Hedgehog (HH) pathway (Zaghloul and Brugmann, 2011). Defects in the structure or signaling functions of cilia cause a group of human syndromes, collectively referred to as ciliopathies, which can manifest in diverse phenotypes including cystic kidneys, retinal degeneration, cognitive impairment, respiratory defects, left-right patterning defects, polydactyly, and skeletal defects (Baker and Beales, 2009; Hildebrandt et al., 2011; Tobin and Beales, 2009). In addition to these phenotypes, craniofacial defects including cleft lip/palate, high-arched palate, jaw disorders, midface dysplasia, craniosynostosis, tongue abnormalities, abnormal dentition, and tooth number and exencephaly are observed in approximately one-third of individuals with ciliopathies (Brugmann et al., 2010b; Zaghloul and Brugmann, 2011). The molecular and developmental etiology of these craniofacial abnormalities remains poorly understood.
HH signaling is intimately involved in forebrain and midface development (Hu and Helms, 1999; Rubenstein and Beachy, 1998). In humans, inherited mutations that compromise pathway activity impair forebrain development and cause hypotelorism (Fuccillo et al., 2006; Hu and Helms, 1999; Hu and Marcucio, 2009; Marcucio et al., 2005; Muenke and Beachy, 2000; Young et al., 2010). For example, mutations in SHH lead to holoprosencephaly (Chiang et al., 1996; Cohen and Shiota, 2002). Meckel syndrome (MKS), a severe ciliopathy, is also characterized by holoprosencephaly and hypotelorism (Chih et al., 2011; Dowdle et al., 2011; Garcia-Gonzalo et al., 2011). MKS-associated genes encode proteins that form a complex that comprises part of the transition zone, a region of the ciliary base that controls ciliogenesis and ciliary membrane protein composition in a tissue-specific manner (Chih et al., 2011; Dowdle et al., 2011; Garcia-Gonzalo et al., 2011; Roberson et al., 2015). Disruption of this transition zone complex results in the impaired ciliary localization of several membrane-associated signaling proteins including smoothened (SMO), adenylyl cyclase 3 (ADCY3), polycystin 2 (PKD2), and ARL13B (Chih et al., 2011; Garcia-Gonzalo et al., 2011; Roberson et al., 2015).
We investigated the molecular underpinnings of forebrain and midface defects in ciliopathies utilizing multiple mouse mutants affecting the transition zone. The mutants exhibited forebrain and midface defects by E9.5, which persisted throughout development. In these mutants, the prechordal plate, an organizer of anterior head development, displayed defects in HH pathway activation at E8.0. These early prechordal plate defects attenuated Shh expression in the adjacent ventral forebrain. Decreased HH signaling increased apoptosis in the ventral neurectoderm and facial ectoderm. Surprisingly, reducing Ptch1 gene dosage rescued the apoptosis and its corresponding midface defect. Thus, investigating the function of the transition zone has revealed a key role of prechordal plate-activated HH signaling in forebrain and midface cell survival. Moreover, our genetic results reveal that inhibition of PTCH1 can prevent ciliopathy-associated midface defects. Based on these mouse genetic models, we propose that the etiology of hypotelorism in human ciliopathies is a failure of the prechordal plate to induce SHH expression in the overlying ventral neuroectoderm.
Results
The ciliary MKS transition zone complex is essential for midline facial development
Individuals affected by developmental ciliopathies, such as Meckel, orofaciodigital, and Joubert syndromes, often display craniofacial phenotypes including holoprosencephaly and hypotelorism (Baker and Beales, 2009; Dowdle et al., 2011; Garcia-Gonzalo et al., 2011). To explore the etiology of these craniofacial defects, we examined the craniofacial development in Tctn2 mouse mutants (Garcia-Gonzalo et al., 2011; Shaheen et al., 2011). TCTN2 is a component of the MKS transition zone complex critical for ciliary localization of several ciliary membrane proteins, including SMO, a key ciliary mediator of HH signaling (Chih et al., 2011; Corbit et al., 2005; Garcia-Gonzalo et al., 2011). Mutations in human TCTN2 cause Meckel and Joubert syndromes (Huppke et al., 2015; Sang et al., 2011; Shaheen et al., 2011).
Tctn2+/- embryos were phenotypically indistinguishable from Tctn2+/+ embryos (Figure 1—figure supplement 1). In contrast, embryonic day (E) 10.5 Tctn2-/- embryos exhibited an approximately 50% decrease in infranasal distance (the distance between the nasal pits, the nostril anlage) (Figure 1A). One day later in gestation (E11.5), Tctn2-/- embryos also exhibited midfacial narrowing, including hypoplasia of the frontonasal prominence and fusion of the two maxillary prominences at the midline (Figure 1A). Thus, TCTN2 is essential for development of the facial midline.
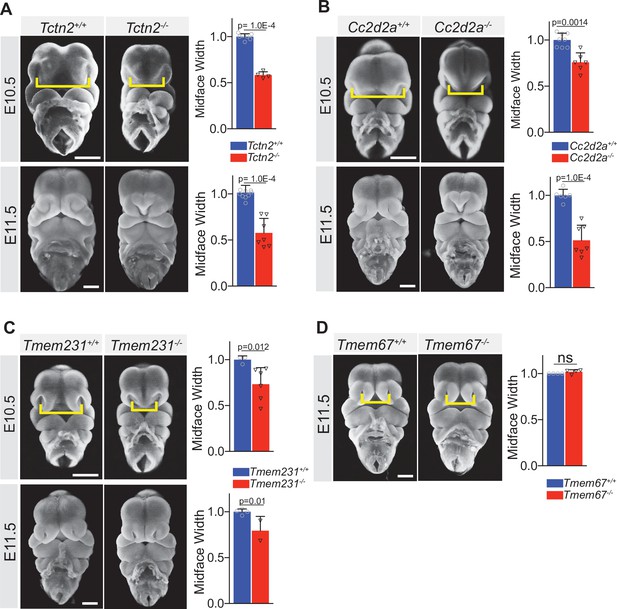
The ciliary Meckel syndrome (MKS) transition zone complex is essential for midline facial development.
Frontal view images of Tctn2 (A), Cc2d2a (B), Tmem231 (C) wild-type and null embryos at embryonic day (E)10.5 and E11.5. Tmem67 null embryos (D) display normal midface width at E11.5. Quantification of midface width (denoted by yellow brackets) at respective timepoints was measured via one-way ANOVA followed by Tukey’s multiple comparisons test. Data are expressed as mean, and error bars represent the standard deviation (SD) with individual data points (N) representing biological replicates (biologically distinct samples). Scale bar indicates 500 μm. ns = not significant.
To assess whether this narrowing of the facial midline is specific to TCTN2, we examined the possible involvement of two other components of the MKS complex, TMEM231 and CC2D2A, in craniofacial development. Human CC2D2A mutations cause Meckel and Joubert syndromes, and TMEM231 mutations cause Meckel, Joubert, and Orofaciodigital syndromes (Noor et al., 2008; Roberson et al., 2015; Shaheen et al., 2013; Srour et al., 2012; Tallila et al., 2008). Similar to Tctn2 mutants, both E10.5 Cc2d2a and Tmem231 mutant embryos exhibited decreased infranasal distance (Figure 1B and C, respectively). The similarity of the midline hypoplasia in all three transition zone mutants suggested a common etiology.
We also examined the involvement of a fourth member of the MKS complex, TMEM67, in craniofacial development. Human mutations in TMEM67 also cause Meckel and Joubert syndromes (Otto et al., 2009; Smith et al., 2006). Mutation of mouse Tmem67 causes phenotypes that are less severe than Tctn2, Tmem231, or Cc2d2a (Garcia-Gonzalo et al., 2011). The mild phenotype of Tmem67 mutants may be attributable to its dispensability for ciliary accumulation of HH pathway activator SMO (Garcia-Gonzalo et al., 2011). Unlike Tctn2, Tmem231, and Cc2d2a mutants, Tmem67 mutants did not exhibit altered infranasal distance (Figure 1D). Thus, some, but not all, MKS components are critical for early facial midline development.
Given the central role of the neural crest in craniofacial development as the main source of craniofacial mesenchyme (Santagati and Rijli, 2003), we tested whether transition zone disruption in neural crest can account for the midline hypoplasia observed in Tctn2 mutants. More specifically, we generated E11.5 Wnt1cre;Tctn2fl/- embryos and quantified midface width (Figure 1—figure supplement 2A). Wnt1cre induces recombination throughout the neural crest, and conditional deletion of Tctn2 in the neural crest abrogated ARL13B localization to cilia (Figure 1—figure supplement 2B,C). Interestingly, removing TCTN2 from the neural crest did not cause hypotelorism. These results indicate that altered transition zone function in the neural crest is not the etiology of midline hypoplasia. Therefore, we investigated functions of TCTN2 in the prechordal plate, an early organizing center also critical for the development of anterior head structures.
Tctn2 mutants exhibit defects in prechordal plate differentiation soon after gastrulation
As Tctn2, Cc2d2a, and Tmem231 mutants all displayed facial midline defects at midgestation, we hypothesized that they shared a role in a patterning event early in craniofacial development. One organizing center critical for early forebrain and craniofacial development is the prechordal plate (Camus et al., 2000; Kiecker and Niehrs, 2001; Muenke and Beachy, 2000; Rubenstein and Beachy, 1998; Som et al., 2014). The prechordal plate is the anterior-most midline mesendoderm, immediately anterior to the notochord and in contact with the overlying ectoderm. The homeobox gene Goosecoid (Gsc) is specifically expressed in the prechordal plate at E8.0 and is a marker of differentiation in this organizing center (Belo et al., 1998; Izpisúa-Belmonte et al., 1993). In contrast, Shh and Brachyury (T) are expressed at E8.0 in both the prechordal plate and notochord and are critical for prechordal plate induction (Aoto et al., 2009). Previous work demonstrated that surgical removal of the rat prechordal plate results in midface defects (Aoto et al., 2009) that seemed similar to those of the mouse Tctn2, Cc2d2a, and Tmem231 mouse mutants.
Therefore, we analyzed the prechordal plate of Tctn2 mutants by examining the expression of prechordal plate differentiation marker Gsc and induction markers Shh and T. Gsc is expressed specifically in the prechordal mesoderm, while Shh and T are expressed in both the prechordal mesoderm and notochord (Dale et al., 1997; Herrmann, 1991; Schulte-Merker et al., 1994). In situ hybridization analysis revealed that in Tctn2 mutants, Shh and T expression in the prechordal plate and notochord were unaffected (Figure 2A). Therefore, TCTN2 is not essential for prechordal plate specification. In contrast, Tctn2 mutants exhibited abrogated Gsc expression in the prechordal plate (Figure 2B), indicating that TCTN2 is critical for prechordal plate differentiation.
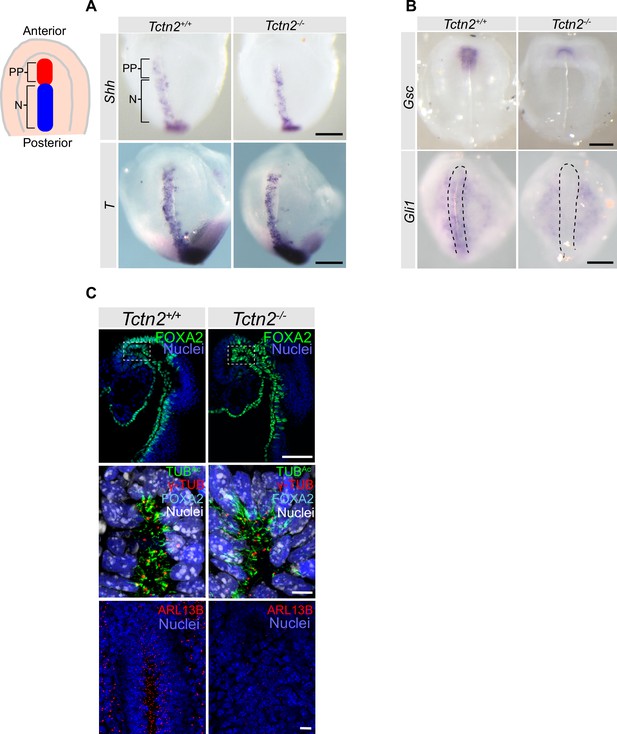
Tctn2 mutants exhibit defects in prechordal plate differentiation soon after gastrulation.
(A) Whole mount in situ hybridization (WM-ISH) of embryonic day (E)8.0 embryos for axial mesendoderm markers Shh and Brachyury (T). (B) WM-ISH of E8.0 embryos for prechordal plate marker Goosecoid (Gsc) and Hedgehog (HH) pathway target Gli1. (C) Whole mount immunofluorecence staining for the prechordal plate transcription factor FOXA2, cilia marker acetylated tubulin (TUBAc), basal body marker gamma tubulin (γ-TUB), and ciliary membrane protein ARL13B in E8.0 embryos. Middle panel in C is magnified region in top panel highlighted by dotted rectangle and rotated 90 degrees. Scale bar in A–B indicates 0.2 mm, C (top panel) is 100 μm, C (middle and bottom panels) is 10 μm.
The transition zone is critical for HH signaling, and one HH protein, SHH, is essential for Gsc expression in the prechordal plate (Aoto et al., 2009; Chih et al., 2011; Garcia-Gonzalo et al., 2011). Therefore, we investigated whether TCTN2 is required for HH signaling in the prechordal plate by examining the expression of the transcriptional target Gli1. Tctn2 mutants exhibited reduced Gli1 expression throughout the axial mesendoderm, including the prechordal plate (Figure 2B). These results indicate that TCTN2 is dispensable for the formation of the prechordal plate, but is required for midline signaling by SHH to induce Gsc expression in this organizing center.
TCTN2 and other members of the MKS complex are required for proper cilia formation in some tissues but not in others (Garcia-Gonzalo et al., 2011). Therefore, we examined whether TCTN2 is required for ciliogenesis in the prechordal plate. The prechordal plate expresses FOXA2 (Jin et al., 2001; Figure 2C). Co-immunostaining embryos for FOXA2 and acetylated tubulin (TUBAc), a marker of cilia, revealed that Tctn2 mutants did not display decreased ciliogenesis in the E8.5 prechordal plate (Figure 2C, middle panel). Previous studies have shown that Tctn1 is expressed in the ventral epithelium of the node of a six-somite stage embryo and in the neural tube, notochord, gut epithelium, and somites at E9.5 (Reiter, 2006). Tctn2 is similarly broadly expressed during mouse development (Diez-Roux et al., 2011; Magdaleno et al., 2006), and CC2D2A is broadly expressed during human development (Mougou-Zerelli et al., 2009).
In cell types in which the MKS complex is dispensable for ciliogenesis, like neural progenitors, it is required for localization of ARL13B to cilia (Garcia-Gonzalo et al., 2011). Therefore, we examined ARL13B localization in E8.0 control embryos and Tctn2 mutants and discovered that ARL13B localization to prechordal plate cilia was attenuated without TCTN2 (Figure 2C, bottom panel). Thus, TCTN2 is not required for ciliogenesis in the prechordal plate, but does control ciliary composition.
Tctn2 mutants display decreased HH signaling in the ventral telencephalon
The axial mesendoderm helps pattern the overlying neurectoderm (Anderson and Stern, 2016; Rubenstein and Beachy, 1998). In the rostral embryo, the prechordal plate patterns the overlying ventral telencephalon via SHH (Chiang et al., 1996; Xavier et al., 2016). As extirpation of the prechordal plate results in decreased SHH activity in the basal telencephalon (Aoto et al., 2009; Aoto and Trainor, 2015), we investigated whether the prechordal plate defects observed in Tctn2 mutants result in mispatterning of the ventral telencephalon. Although Shh expression in the notochord was unaffected in Tctn2 mutants at E8.75, it was severely reduced in the ventral telencephalon (Figure 3A). This reduced expression of Shh in the ventral telencephalon persisted at E9.5 (Figure 3A). These results are concordant with previous findings that mutations in genes encoding other transition zone components disrupt brain development, resulting in holoprosencephaly, reduced telencephalon size, or exencephaly (Dowdle et al., 2011; Garcia-Gonzalo et al., 2011; Reiter, 2006).
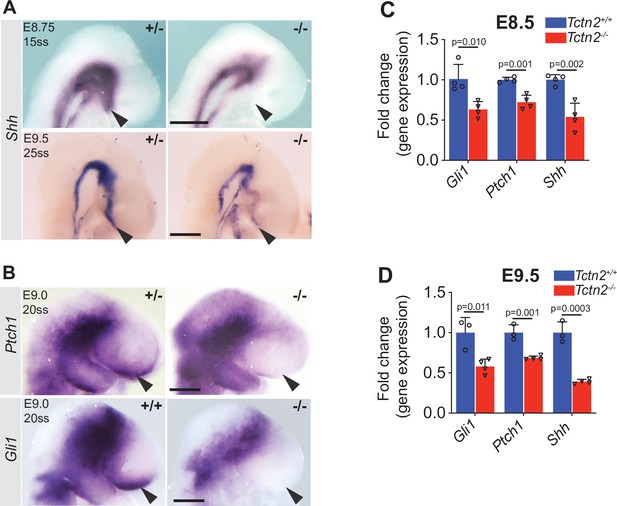
Tctn2 mutants display decreased Hedgehog (HH) signaling in the ventral telencephalon.
Whole mount in situ hybridization (WM-ISH) for Shh in Tctn2 control and mutant embryos at embryonic day (E)8.75 and E9.5 (A) show reduced expression in the ventral telencephalons (arrowheads) of mutant embryos . WM-ISH for HH pathway targets Ptch1 and Gli1 also show reduced expression in the ventral telencephalon in Tctn2 mutants (B, arrowheads). Quantitative real-time polymerase chain reaction (RT-qPCR) analysis of RNA transcripts isolated from E8.5 and E9.5 Tctn2 control and mutant heads (C and D, respectively) show reduced levels of Gli1, Ptch1, and Shh transcripts in the absence of TCTN2, consistent with WM-ISH results. Data in C, D represent mean, and error bar represents the standard deviation (SD). Sample size in C and D indicated with 3-4 biological replicates per assay. Student’s t test performed for statistical analysis. Scale bar indicates 0.5 mm.
To assess whether HH pathway activity was compromised by the absence of TCTN2, we assessed the expression of the HH transcriptional targets Gli1 and Ptch1. Whole mount in situ hybridization (WM-ISH) of Tctn2 mutants revealed dramatically reduced or absent expression of both Gli1 and Ptch1, especially in the basal forebrain (Figure 3B). Consistent with the WM-ISH data, quantitative real-time polymerase chain reaction (RT-qPCR) analysis of E8.5 (Figure 3C) and E9.5 (Figure 3D) Tctn2 mutant heads also revealed decreased expression of Shh, Ptch1, and Gli1, revealing that Tctn2 mutants exhibit both an early defect in prechordal plate differentiation and a defect in HH signaling in the adjacent neurectoderm.
TCTN2 protects neurectoderm and facial ectoderm from apoptosis
In the developing craniofacial complex, SHH induces cell proliferation (Hu and Helms, 1999; Hu et al., 2015). Therefore, we assessed if the reduction in facial midline width in Tctn2 mutants was due to decreased cell proliferation. More specifically, we measured cell proliferation by quantitating phospho-histone H3 (pHH3) in the components of the craniofacial complex – the forebrain, hindbrain, facial ectoderm, and mesenchyme (Figure 4A and B). Tctn2 mutants showed no differences in amount or spatial distribution of cell proliferation.
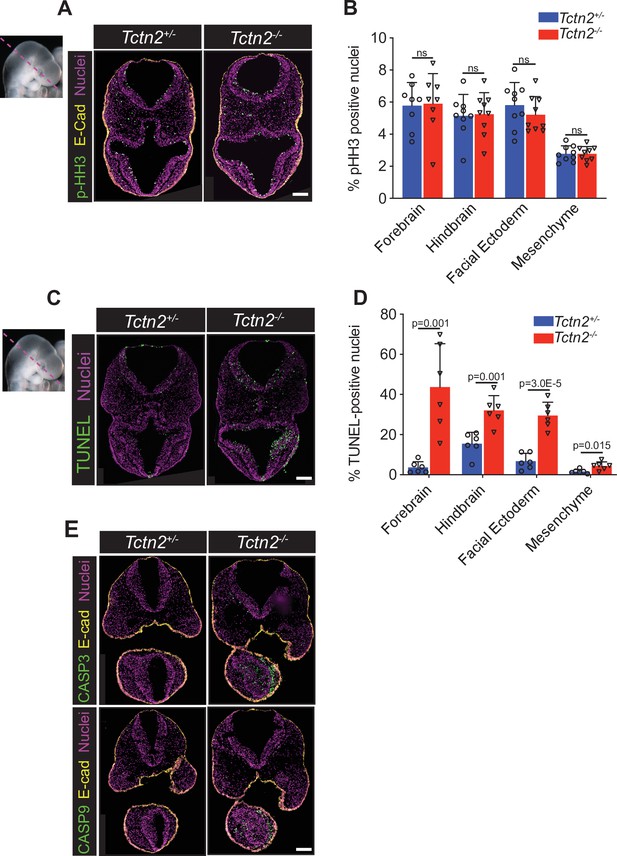
TCTN2 protects the neurectoderm and facial ectoderm from apoptosis.
Immunostaining for proliferation marker phospho-histone H3 (pHH3) in transverse sections of Tctn2 control and mutant embryonic day (E9.5) embryos (A) with corresponding quantification (B). (C) TUNEL staining for analysis of apoptosis in Tctn2 E9.5 embryos with corresponding quantification (D). (E) Immunostaining for intrinsic apoptotic pathway components cleaved-caspase-3 and cleaved-caspase-9 in Tctn2 control and mutant E9.5 embryos. Quantified data represent N = 3 biological replicates (biologically distinct samples) with a minimum of two sections analyzed per sample. Student’s t test performed for statistical analysis. Data in B, D represent the mean, and error bars represent the standard deviation (SD). Scale bar indicates 100 μm. ns = not significant.
In other developmental contexts, HH signaling promotes cell survival (Ahlgren and Bronner-Fraser, 1999; Aoto et al., 2009; Aoto and Trainor, 2015; Litingtung and Chiang, 2000). Therefore, we assessed apoptosis in the craniofacial complex of Tctn2 mutants. Quantification of TUNEL staining revealed that apoptosis was increased in the neurectoderm, facial ectoderm, and mesenchyme of Tctn2 mutants compared to controls, and most dramatically in the ventral telencephalon (Figure 4C and D). To further test whether apoptosis is increased in the absence of TCTN2, we stained for activators of the intrinsic apoptotic pathway, cleaved-caspase-3 and caspase-9 (activated CASP3 and CASP9). Both activated CASP3 and CASP9 were increased in the ventral telencephalon, facial ectoderm, and mesenchyme of Tctn2 mutants at E9.5 (Figure 4E). These data indicate that TCTN2 is required to protect against cell death, but does not affect proliferation, in the neurectoderm, non-neural ectoderm and neural crest mesenchyme. As SHH also protects neurectoderm from apoptosis (Thibert et al., 2003), we propose that TCTN2 mediates cell survival by promoting HH signaling and that the increase in cell death in the neurectoderm, mesenchyme and facial ectoderm underlies the midline hypoplasia in transition zone mutants.
Our finding that selective disruption of transition zone function in the neural crest did not contribute to midline growth (Figure 1—figure supplement 2) coupled with increased apoptosis in the Tctn2 mutant, neurectoderm and facial ectoderm (Figure 4C–D) led us to investigate where TCTN2 is required to coordinate midline facial development. Isl1Cre induced robust reporter recombination in the prechordal plate at E8.5 (Figure 4—figure supplement 1A) and Sox1Cre induced recombination in the neuroectoderm by E9.5 (Figure 4—figure supplement 1B). Using Isl1Cre and Sox1Cre, we removed Tctn2 from the prechordal plate or neurectoderm, respectively. Both Isl1Cre;Tctn2fl/- and Sox1Cre;Tctn2fl/- embryos displayed no decrease in midline width at E11.5 (Figure 4—figure supplement 1C and D).
Similarly, we investigated the function of TCTN2 in the facial ectoderm and telencephalon. Tcfap2aCre and Foxg1Cre activated recombination in the facial ectoderm or telencephalon and ectoderm, respectively (Figure 4—figure supplement 1E, F). Both Tcfap2aCre;Tctn2fl/- and Foxg1Cre;Tctn2fl/- embryos displayed no decrease in midline width at E11.5 (Figure 4—figure supplement 1G, H).
Analysis of ARL13B localization to cilia at E8.5 and E9.5 in the prechordal plate and neurectoderm in Isl1Cre;Tctn2fl/- and Sox1Cre;Tctn2fl/- embryos, respectively, revealed that there was no alteration in ciliogenesis or ciliary localization of ARL13B (Figure 4—figure supplement 2A and B). Analysis of ciliary ARL13B localization in the ectoderm of E11.5 Tcfap2aCre;Tctn2fl/- mutants similarly revealed persistent ARL13B localization to cilia (Figure 4—figure supplement 2C). In contrast, Foxg1Cre;Tctn2fl/- mutants exhibited loss of ARL13B ciliary localization in the basal telencephalon at E11.5 (Figure 4—figure supplement 2D). These results indicate that Tctn2 conditional deletion in either the prechordal plate, neurectoderm, facial ectoderm, or forebrain individually fails to recapitulate the midline narrowing observed in Tctn2-/- embryos. However, the persistence of ARL13B at cilia suggests that TCTN2 function persists for some time after recombination or TCTN2 functions in the prechordal plate, neurectoderm and facial ectoderm may be important for facial development.
Reducing Ptch1 gene dosage rescues the facial midline defect in transition zone mutants
To assess whether decreased HH signaling is not just correlated with midfacial hypoplasia in transition zone mutants, but is causative, we investigated whether modulating the HH pathway could rescue the midface defects. We employed a strategy targeting Ptch1, a negative regulator of the HH pathway, by generating Tctn2-/-;Ptch1+/- embryos and comparing them to Tctn2-/-;Ptch1+/+ embryos. Surprisingly, removing a single allele of Ptch1 in Tctn2 mutants restored midface width at E11.5 (Figure 5A and B).
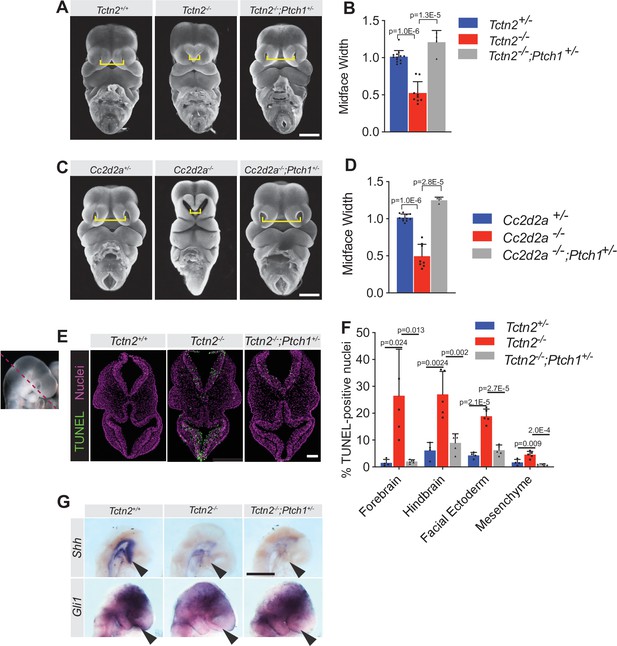
Reducing Ptch1 gene dosage rescues the facial midline defect in transition zone mutants.
(A) Frontal images of Tctn2 wild-type, mutant, and Ptch1+/- rescue embryonic day (E)11.5 embryos with corresponding midline width quantification (B). (C) Frontal images of Cc2d2a wild-type, mutant, and Ptch1+/- rescue E11.5 embryos with corresponding midline width quantification (D). (E) TUNEL assay sections of E9.5 Tctn2 wild-type, mutant, and Ptch1+/- rescue with corresponding quantification (F). (G) Whole mount in situ hybridization (WM-ISH) of E9.5 Tctn2 wild-type, mutant, and Ptch1+/- rescue embryos for Shh and Gli1. Quantified data represent N = 3 biological replicates (biologically distinct samples) with a minimum of two sections analyzed per sample. Data in B, D, F represent the mean, and error bars represent the standard deviation (SD). For statistical analysis, one-way ANOVA was performed with Tukey’s multiple comparison test. Scale bars indicate 500 μm (A, C, G) and 100 μm (E).
To assess whether removing a single allele of Ptch1 restores midface width in other transition zone mutants, we generated Cc2d2a-/-;Ptch1+/- and Cc2d2a-/-;Ptch1+/+ embryos. As with Tctn2, removing a single allele of Ptch1 restored midface width in Cc2d2a mutants (Figure 5C and D). Thus, reducing Ptch1 gene dosage rescues midface expansion in both models of ciliopathy-associated hypoplasia.
As we had hypothesized that increased apoptosis underlay the midface hypoplasia of Tctn2-/-;Ptch1+/+ embryos, we assessed apoptosis in Tctn2-/-;Ptch1+/- embryos via TUNEL staining. Tctn2-/-;Ptch1+/- exhibited less apoptosis than Tctn2-/-;Ptch1+/+ embryos, with a restriction of apoptosis in the ventral telencephalon (Figure 5E–F). These results bolster the hypothesis that increased midline apoptosis accounts for the midline hypoplasia of transition zone mutants.
As genes encoding transition zone MKS components are epistatic to Ptch1 (Reiter, 2006), we pondered how reducing Ptch1 gene dosage restored facial midline development to transition zone MKS component mutants. The best studied role for PTCH1 is in repression of the HH signal transduction pathway. Therefore, we examined HH signal transduction pathway activity in Tctn2-/-;Ptch1+/+ and Tctn2-/-;Ptch1+/- embryos. WM-ISH revealed that expression of neither Shh nor Gli1 was increased in the ventral telencephalons of Tctn2-/-;Ptch1+/- in comparison to Tctn2-/-;Ptch1+/+ embryos (Figure 5G). Thus, the restoration of midface growth by reduction of Ptch1 gene dosage is not due to a restoration of HH signal transduction.
In addition to its role in regulating HH signal transduction, PTCH1 exhibits pro-apoptotic activity in vitro (Thibert et al., 2003). In the absence of ligand, PTCH1 C-terminal domain is cleaved and binds scaffolding proteins TUCAN1 and DRAL to recruit caspase-9 and activate caspase-3, resulting in apoptosis. The colocalization of another PTCH1-binding protein that regulates apoptosis, X-linked inhibitory apoptosis protein (XIAP), with PTCH1 at cilia (Aoto and Trainor, 2015), raises the possibility that PTCH1 cleavage occurs at the primary cilium to induce apoptosis. In addition, PTCH1 may induce apoptosis at the plasma membrane. As reducing Ptch1 gene dosage reduces apoptosis without increasing HH signal transduction in Tctn2 mutants, our data supports a model in which the PTCH1-mediated death of the midline neurectoderm, facial ectoderm, and neural crest-derived mesenchyme, and not alterations in HH signal transduction within those cells, is the etiology of midface defects in transition zone mutants. Taken together, these results suggest a working model for how ciliary HH signaling regulates midface development.
In wild-type embryos, HH signaling within the prechordal plate is critical for Gsc expression and the induction of Shh in the adjacent neurectoderm and inhibition of apoptosis (Figure 6A). In transition zone mutants, defects in prechordal plate signaling cause reduced SHH in the neurectoderm, resulting in increased PTCH1-mediated cell death and midline collapse (Figure 6B). In transition zone mutants lacking a single allele of Ptch1, reduced SHH in the neurectoderm persists, but the attenuated PTCH1 is no longer sufficient to induce extensive cell death, allowing for normal midline facial development (Figure 6C).
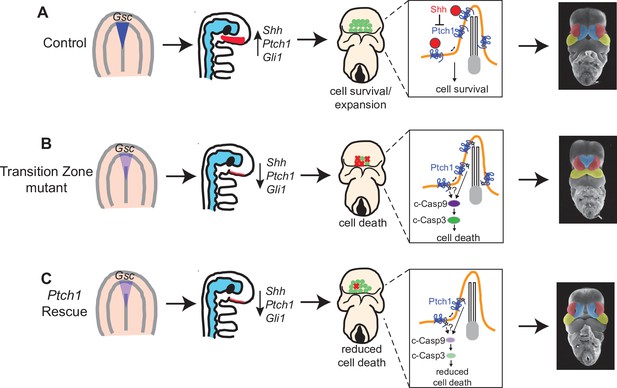
Model for transition zone coordination of facial midline development.
(A) In wild-type embryos, the transition zone complex mediates signaling in the prechordal plate and Hedgehog (HH) pathway activation in the adjacent neurectoderm to at allow for cell survival and normal midline development. (B) In transition zone mutants, disrupted signaling in the prechordal plate results in reduced Shh and HH pathway activation in the neurectoderm resulting in increased cell death and corresponding collapse of the facial midline. (C) In transition zone mutants with Ptch1 haploinsufficiency (Ptch1 rescue), reduced cell death allows for normal midface development despite persistent reduction of Shh and HH pathway activation in the neurectoderm.
Discussion
Using a combination of genetic, developmental, and biochemical techniques, we have identified a mechanism by which disruption of MKS transition zone proteins (TCTN2, CC2D2A, and TMEM231) results in midline hypoplasia and hypotelorism. We traced the origin of the molecular defect contributing to the midline phenotype to the prechordal plate, defects in which resulted in reduced HH pathway activation and cell survival in the adjacent neurectoderm and facial midline collapse. We uncovered Ptch1 gene dosage as a key mediator of cell survival in the facial midline of transition zone mutants, as loss of a single allele of Ptch1 rescued cell survival and midline development in Tctn2 and Cc2d2a mutants. Together, these results reveal a new paradigm whereby primary cilia mediate signal crosstalk from the prechordal plate to the adjacent neurectoderm to promote cell survival, without which the facial midline collapses and hypotelorism results.
Different ciliopathies are associated with either narrowing or expansion of the facial midline (hypotelorism or hypertelorism) (Schock et al., 2015; Zaghloul and Brugmann, 2011). Severe ciliopathies associated with perinatal lethality, such as MKS, can present with hypotelorism or hypertelorism while other ciliopathies such as Joubert syndrome typically present with hypertelorism (Brugmann et al., 2010b; MacRae et al., 1972; Schock and Brugmann, 2017). How disruption of ciliary functions can give rise to these opposing phenotypes has been an active area of interest. Hypertelorism is attributable to roles for cilia in promoting GLI3 repressor formation in neural crest cells (Brugmann et al., 2010a; Chang et al., 2014; Chang et al., 2016; Liu et al., 2014). Our work implicates a distinct etiology of hypotelorism: rather than involving neural crest, midline hypoplasia can be caused by defects in the ciliary transition zone in the prechordal plate.
The earliest alteration we detected in Tctn2-/- signaling centers that regulate craniofacial development was in the prechordal plate at the end of gastrulation. TCTN2 was dispensable for the expression of Shh and T in the prechordal plate, indicating that induction and specification of the prechordal plate were not dependent on transition zone function. In contrast, TCTN2 was essential for prechordal plate expression of Gli1 and Gsc. In tissues such as the limb bud, TCTN2 is dispensable for ciliogenesis but critical for ciliary HH signaling and the induction of HH target genes such as Gli1 (Chih et al., 2011; Dowdle et al., 2011; Garcia-Gonzalo et al., 2011). We found that, similarly in the prechordal plate, TCTN2 is dispensable for ciliogenesis but critical for induction of Gli1.
In many developmental events, such as limb patterning, SHH signals to neighboring cells to induce a pattern (Panman and Zeller, 2003; Zhulyn et al., 2014). In other developmental events, such as notochord to neural tube signaling, SHH signals produced by the notochord induce the expression of Shh in the overlaying neural tube (Fuccillo et al., 2006). SHH produced by the prechordal plate may fall into the latter category, as the absence of Shh expression in Tctn2-/- embryos presages reduced Shh expression and reduced expression of HH pathway transcriptional targets Gli1 and Ptch1 in the region of the basal forebrain sometimes referred to as the rostral diencephalon ventral midline. Thus, in the posterior midline, the notochord induces Shh expression in overlying neuroectoderm, and in the anterior midline, the prechordal plate induces Shh expression in the overlying neuroectoderm. In the failure of the prechordal plate to induce Shh expression in the overlying neurectoderm, Tctn2 mutants recapitulate previous observations of Lrp2 mutants which display attenuated responses to SHH (Christ et al., 2012). One possible mechanism by which SHH may activate expression of Shh in the basal forebrain is via the induction of the transcription factor SIX3. SIX3 is regulated by HH signaling and required for the induction of Shh in the developing forebrain (Geng et al., 2008; Jeong et al., 2008). However, our observation that Six3 expression is unaltered in the forebrains of Tctn2 mutants diminishes support for this hypothesis.
In caudal neural tube and limb patterning, HH signals induce patterning. In hair follicles and cerebellar granule cells, HH signaling promotes proliferation. In addition to roles in patterning and proliferation, HH signals can bind to PTCH1 to inhibit apoptosis (Aoto and Trainor, 2015; Borycki et al., 1999; Thibert et al., 2003). Our data are consistent with a role of PTCH1 in promoting apoptosis in the neuroectoderm and facial ectoderm which is inhibited by prechordal plate-produced SHH. In the absence of TCTN2, the ventral telencephalon does not produce SHH, releasing PTCH1 to promote apoptosis in the midline and resulting in midface hypoplasia. This model is consistent with previous data demonstrating that surgical ablation of the prechordal plate reduces the forebrain (Aoto et al., 2009). Increased cleaved-caspase-3 and caspase-9 staining in the basal forebrain and facial ectoderm of E9.5 Tctn2 mutants provides further support for apoptosis contributing to the associated midline hypoplasia.
We speculate that in tissues, such as the developing spinal cord where cilia are required for SHH expression in the floor plate, ciliary dysfunction will cause increased apoptosis. In other tissues, such as the limb bud where cilia are not required for SHH expression in the zone of proliferating activity, we predict that ciliary dysfunction will not cause increased apoptosis. Thus, ciliary dependence of SHH expression may etermine which tissues, like the craniofacial midline, increase apoptosis upon ciliary dysfunction.
To try to narrow down the tissues in which transition zone function is critical for midline facial development, we used conditional mouse genetics to delete Tctn2 in different tissues that comprise the craniofacial complex. Deletion of Tctn2 in the prechordal plate (via Isl1Cre) or the neurectoderm (via Sox1Cre) did not recapitulate the midline hypoplasia observed in Tctn2-/- embryos (Figure 4—figure supplement 1). Similarly, deletion of Tctn2 in the facial ectoderm (via Tcfap2aCre) or in the forebrain and facial ectoderm (via Foxg1Cre) also failed to result in midline hypoplasia (Figure 4—figure supplement 1). These results could reflect the dispensibility of TCTN2 in these tissues for facial development, or could reflect residual TCTN2 function after Tctn2 recombination. This latter possibility is supported by persistent ARL13B localization at the cilium in several tissues after conditional deletion of Tctn2. As the half-life of TCTN2 and the TCTN2 level required to sustain transition zone activity are unclear, residual TCTN2 activity may support normal ciliary signaling even after conditional gene ablation.
Surprisingly, removing one allele of Ptch1 fully rescues the midface defect in both Tctn2 and Cc2d2a transition zone cilia mutants. Even more surprisingly, this phenotypic rescue is not associated with restoration of either Shh expression or HH pathway activation in the basal forebrain. We propose that reducing Ptch1 levels attenuates the PTCH1-mediated pro-apoptotic program normally attenuated by SHH in the basal forebrain. However, it remains possible that reducing PTCH1 levels activates GLI effectors to induce an anti-apoptotic program that does not include general HH target genes or Shh.
In summary, we have identified the primary cilia transition zone as a critical regulator of facial midline development. The transition zone component TCTN2 was critical for SHH signaling in the prechordal plate and uncovered a signaling paradigm whereby the transition zone promotes cell survival by mediating crosstalk between the prechordal plate and neurectoderm to promote HH pathway activation. These results provide insights into how primary cilia mediate cell survival to promote facial development.
Materials and methods
Reagent type (species) or resource | Designation | Source or reference | Identifiers | Additional information |
---|---|---|---|---|
Antibody | Anti-Arl13b (rabbit polyclonal) | Proteintech | 17711–1-AP | (1:1000) |
Antibody | Anti-cleaved-caspase-3 (Asp175) (rabbit polyclonal) | Cell Signaling | #9664 | (1:400) |
Antibody | Anti-cleaved-caspase-9 (Asp353) | Cell Signaling | #9509 | (1:100) |
Antibody | Anti-phospho-histone H3 (Ser28) (rabbit polyclonal) | Cell Signaling | #9713 | (1:400) |
Antibody | Anti-acetylated tubulin (mouse monoclonal) | Sigma-Aldrich | T6793 | (1:1000) |
Antibody | Anti-FoxA2 (rabbit monoclonal) | abcam | Ab108422 | (1:400) |
Antibody | Anti-Gamma tubulin (goat polyclonal) | Santa Cruz Biotechnology | Sc-7396 | (1:200) |
Antibody | Anti-GFP (chicken polyclonal) | Aves Labs | GFP-1020 | (1:1000) |
Antibody | Anti-E-cadherin (rat monoclonal) | Invitrogen | 13–1900 | (1:1000) |
Commercial assay or kit | RNAeasy Micro Kit | QIAGEN | 74004 | |
Commercial assay or kit | In Situ Cell Death Detection Kit | Roche | 11684795910 | |
Commercial assay or kit | iScript cDNA synthesis kit | BioRad | 1708891 | |
Genetic reagent (Mus musculus) | Tctn2tm1.1Reit | PMID:21565611 | MGI:5292130;RRID:MGI:5292219 | |
Genetic reagent (Mus musculus) | Cc2d2aGt(AA0274)Wtsi | PMID:21725307 | MGI:4344514;RRID:MGI:5292228 | |
Genetic reagent (Mus musculus) | Tmem231Gt(OST335874)Lex | PMID:22179047 | MGI:4284576;RRID:MGI:5301844 | |
Genetic reagent (Mus musculus) | Tmem67tm1Dgen | PMID:21725307 | MGI:5292220;RRID:MGI:5292226 | |
Genetic reagent (Mus musculus) | Wnt1Cre: H2az2Tg(Wnt1-cre)11Rth | PMID:9843687 | MGI:2386570;RRIDSupplemental:IMSR_JAX:003829 | Gift from Brian Black |
Genetic reagent (Mus musculus) | Isl1tm1(cre)Sev | PMID:16556916 | MGI:3623159;RRID:IMSR_HAR:3351 | Gift from Brian Black |
Genetic reagent (Mus musculus) | Sox1tm1(cre)Take | PMID:17604725 | MGI:3807952;RRID:IMSR_RBRC05065 | Gift from Jeff Bush |
Genetic reagent (Mus musculus) | Foxg1tm1(cre)Skm | PMID:10837119 | MGI:1932522;RRID:IMSR_JAX:004337 | Gift from Stavros Lomvardas |
Genetic reagent (Mus musculus) | Tg(Tcfap2a-cre)1Will | PMID:21087601 | MGI:4887352;RRID:MGI:4887452 | Gift from Trevor Williams |
Sequence-based reagent | Shh in situ probe | PMID:7916661 | Gift from Andrew McMahon | |
Sequence-based reagent | Gsc in situ probe | PMID:1352187 | Gift from Edward De Robertis | |
Sequence-based reagent | Ptch1 in situ probe | PMID:10395791 | Gift from Lisa Goodrich | |
Sequence-based reagent | RT-qPCR primers | This paper | *See Supplementary file 1 | |
Sequence-based reagent | Genotyping primers | This paper | *See Supplementary file 1 | |
Software, algorithm | GraphPad Prism | GraphPad Prism (https://graphpad.com) | RRID:SCR_002798 | |
Software, algorithm | ImageJ | ImageJ (http://imagej.nih.gov/ij/) | RRID:SCR_003070 |
Mouse strains
Request a detailed protocolAll mouse protocols were approved by the Institutional Animal Care and Use Committee (IACUC) at the University of California, San Francisco. Tctn2+/- (Tctn2tm1.1Reit), Cc2d2a+/- (Cc2d2aGt(AA0274)Wtsi), Tmem231+/- (Tmem231Gt(OST335874)Lex), and Tmem67+/- (Tmem67tm1Dgen) mouse allele references can be found in the Key Resources table (Garcia-Gonzalo et al., 2011). Wnt1Cre (Tg(Wnt1-cre)11Rth) and Islet1Cre (Isl1tm1(cre)Sev) mice were obtained from Brian Black, Sox1Cre (Sox1tm1(cre)Take) mice were obtained from Jeff Bush, Foxg1Cre (Foxg1tm1(cre)Skm) mice were obtained from Stavros Lomvardas, and Tcfap2aCre (Tg(Tcfap2a-cre)1Will) mice were obtained from Trevor Williams. The Ptch1tm1Mps allele was used in this study as a null allele. The Tctn2 conditional allele (Tctn2lox or Tctn2tm1cReit) was derived from the Tctn2tm1aReit allele from which the validated Tctn2tm1.1Reit null allele was previously derived (Garcia-Gonzalo et al., 2011; Sang et al., 2011). More specifically, we removed the puromycin resistance cassette of Tctn2tm1aReit by Cre-mediated recombination, leaving two loxP sites flanking the first three Tctn2 exons to generate Tctn2fl. All mice were maintained on a C57BL/6J background. For timed matings, noon on the day a copulation plug was detected was considered to be 0.5 days postcoitus. Genotyping primers for all mouse strains used in this study can be found in Supplementary file 1.
Immunofluorescence
Request a detailed protocolThe antibodies used in this study were rabbit α-ARL13B (1:1000, Proteintech 17711–1-AP), rabbit α-cleaved-caspase-3 (Asp175) (1:400, Cell Signaling #9664), rabbit α-cleaved-caspase-9 (Asp353) (1:100, Cell Signaling #9509), rabbit α-phospho-histone H3 (Ser28) (1:400, Cell Signaling #9713), chicken anti-GFP (1:1000, Aves labs GFP-1020), goat gamma-tubulin (1:200, Santa Cruz sc7396), mouse acetylated-tubulin (1:1000, Sigma T6793), rat E-cadherin (1:1000, Invitrogen 13–1900), and rabbit FoxA2 (1:400 abcam ab108422). The In Situ Cell Death Detection Kit, Fluorescein (Roche) was used for TUNEL cell death assay. For immunofluorescence antibody staining of frozen tissue sections, embryos were fixed overnight in 4% PFA/PBS, washed in PBS and cryopreserved via overnight incubation in 30% sucrose/PBS. Embryos were embedded in OCT and frozen at –80°C. Frozen OCT blocks were cut into 10 μM sections. For immunostaining, frozen sections were washed 3 × 5’ in PBST (0.1%Tween-20/PBS) followed by blocking for 2hr in blocking solution (5% donkey serum in PBS + 0.3% Triton X-100 +0.2% Na-azide). Slides were incubated overnight in primary antibody diluted in blocking solution at 4 degrees. The following day, slides were washed 3 × 10’ in PBST, stained with appropriate AlexaFluor 488, 568, or 647 conjugated secondary antibodies (Life Technologies) at 1:1000 and Hoecsht or DAPI nuclear stain in blocking buffer for 1 hr, rinsed 3 × 10’ with PBST and mounted using Fluoromount-G (Southern Biotech). All steps performed at room temperature unless otherwise noted. *Note: For gamma-tubulin antibody staining, antigen retrieval by incubating with 1%SDS/PBST for 5 min prior to blocking and primary antibody incubation is required for good staining. Stained samples were imaged on a Leica SP-5 confocal microscope. Images were processed using FIJI (ImageJ).
In situ hybridization
Request a detailed protocolWM-ISH was performed as previously described (Harrelson et al., 2012). DIG-labeled riboprobes were made using plasmids from the following sources: Shh (Echelard et al., 1993), Gsc (Blum et al., 1992), Ptch1 (Goodrich et al., 1999), Foxa2 (Brennan et al., 2001), Gli1 (EST W65013).
RT-qPCR
Request a detailed protocolFor gene expression studies, RNA was extracted from E8.5/E9.5 embryo heads using an RNAeasy Micro Kit (QIAGEN), and cDNA synthesis was performed using the iScript cDNA synthesis kit (BioRad). RT-qPCR was performed using EXPRESS Sybr GreenER 2× master mix with ROX (Invitrogen) and primers homologous to Shh, Ptch1, or Gli1 on an ABI 7900HT RT-PCR machine. Expression levels were normalized to the geometric mean of three control genes (Actb, Hprt and Ubc), average normalized Ct values for control and experimental groups determined, and relative expression levels determined by ΔΔCt. The RT-qPCR of each RNA sample was performed in quadruplicate with a minimum of N = 3 biological replicates (biologically distinct samples) per genotype. All primers used for RT-qPCR experiments can be found in Supplementary file 1.
Embryo processing for midface imaging
Request a detailed protocolEmbryos were harvested in ice-cold PBS, staged by counting somite number, and fixed o/n at 4 degrees in 4%PFA/PBS. Embryo heads were removed and stained in 0.01% ethidium bromide in PBS at room temperature for 15 min. Embryos were positioned using glass beads in PBS and imaged on a Leica MZ16 F fluorescence stereomicroscope.
Image Quantification
Request a detailed protocolFor 2D midface width quantification, the infranasal distance was measured using FIJI software by drawing a line between the center of each nasal pit. For quantification of cell death and proliferation assays, a minimum of two sections per embryo were quantified over three biological replicates (biologically distinct samples). Staining with epithelial marker E-cadherin was used for quantification of facial ectoderm while nuclear morphology was used to separate mesenchyme, hindbrain, and forebrain tissue compartments. For quantification, threshold was first set for each image followed by binary watershed separation to obtain accurate nuclei counts. The percentage of TUNEL+ or pHH3+ nuclei were compared between Tctn2 mutant and control samples.
Data availability
All data generated or analysed during this study are included in the manuscript and supporting files.
References
-
Organizers in DevelopmentCurrent Topics in Developmental Biology 117:435–454.https://doi.org/10.1016/bs.ctdb.2015.11.023
-
Co-ordinated brain and craniofacial development depend upon Patched1/XIAP regulation of cell survivalHuman Molecular Genetics 24:698–713.https://doi.org/10.1093/hmg/ddu489
-
Making sense of cilia in disease: The human ciliopathiesAmerican Journal of Medical Genetics Part C 151C:281–295.https://doi.org/10.1002/ajmg.c.30231
-
The prechordal midline of the chondrocranium is defective in Goosecoid-1 mouse mutantsMechanisms of Development 72:15–25.https://doi.org/10.1016/s0925-4773(97)00204-9
-
Sonic hedgehog controls epaxial muscle determination through Myf5 activationDevelopment 126:4053–4063.
-
A primary cilia-dependent etiology for midline facial disordersHuman Molecular Genetics 19:1577–1592.https://doi.org/10.1093/hmg/ddq030
-
Craniofacial ciliopathies: A new classification for craniofacial disordersAmerican Journal of Medical Genetics Part A 152A:2995–3006.https://doi.org/10.1002/ajmg.a.33727
-
Teratogenesis of holoprosencephalyAmerican Journal of Medical Genetics 109:1–15.https://doi.org/10.1002/ajmg.10258
-
Disruption of a ciliary B9 protein complex causes Meckel syndromeAmerican Journal of Human Genetics 89:94–110.https://doi.org/10.1016/j.ajhg.2011.06.003
-
Morphogen to mitogen: the multiple roles of hedgehog signalling in vertebrate neural developmentNature Reviews. Neuroscience 7:772–783.https://doi.org/10.1038/nrn1990
-
CiliopathiesThe New England Journal of Medicine 364:1533–1543.https://doi.org/10.1056/NEJMra1010172
-
The role of sonic hedgehog in normal and abnormal craniofacial morphogenesisDevelopment 126:4873–4884.
-
Tectonic gene mutations in patients with Joubert syndromeEuropean Journal of Human Genetics 23:616–620.https://doi.org/10.1038/ejhg.2014.160
-
Regulation of a remote Shh forebrain enhancer by the Six3 homeoproteinNature Genetics 40:1348–1353.https://doi.org/10.1038/ng.230
-
Otx2 and HNF3beta genetically interact in anterior patterningThe International Journal of Developmental Biology 45:357–365.
-
The role of prechordal mesendoderm in neural patterningCurrent Opinion in Neurobiology 11:27–33.https://doi.org/10.1016/s0959-4388(00)00170-7
-
Reproductive ToxicologyReproductive Toxicology 48:88–97.https://doi.org/10.1016/j.reprotox.2014.05.009
-
Ocular manifestations of the Meckel syndromeArchives of Ophthalmology 88:106–113.https://doi.org/10.1001/archopht.1972.01000030108028
-
Molecular interactions coordinating the development of the forebrain and faceDevelopmental Biology 284:48–61.https://doi.org/10.1016/j.ydbio.2005.04.030
-
Genetics of ventral forebrain development and holoprosencephalyCurrent Opinion in Genetics & Development 10:262–269.https://doi.org/10.1016/S0959-437X(00)00084-8
-
CC2D2A, encoding a coiled-coil and C2 domain protein, causes autosomal-recessive mental retardation with retinitis pigmentosaAmerican Journal of Human Genetics 82:1011–1018.https://doi.org/10.1016/j.ajhg.2008.01.021
-
Hypomorphic mutations in meckelin (MKS3/TMEM67) cause nephronophthisis with liver fibrosis (NPHP11)Journal of Medical Genetics 46:663–670.https://doi.org/10.1136/jmg.2009.066613
-
Patterning the limb before and after SHH signallingJournal of Anatomy 202:3–12.https://doi.org/10.1046/j.1469-7580.2003.00138.x
-
TMEM231, mutated in orofaciodigital and Meckel syndromes, organizes the ciliary transition zoneThe Journal of Cell Biology 209:129–142.https://doi.org/10.1083/jcb.201411087
-
Patterning of the embryonic forebrainCurrent Opinion in Neurobiology 8:18–26.https://doi.org/10.1016/s0959-4388(98)80004-4
-
Cranial neural crest and the building of the vertebrate headNature Reviews. Neuroscience 4:806–818.https://doi.org/10.1038/nrn1221
-
Discovery, Diagnosis, and Etiology of Craniofacial CiliopathiesCold Spring Harbor Perspectives in Biology 9:a028258.https://doi.org/10.1101/cshperspect.a028258
-
Expression of zebrafish goosecoid and no tail gene products in wild-type and mutant no tail embryosDevelopment 120:843–852.
-
A TCTN2 mutation defines a novel Meckel Gruber syndrome locusHuman Mutation 32:573–578.https://doi.org/10.1002/humu.21507
-
Mutations in TMEM231 cause Meckel-Gruber syndromeJournal of Medical Genetics 50:160–162.https://doi.org/10.1136/jmedgenet-2012-101431
-
Illustrated review of the embryology and development of the facial region, part 3: an overview of the molecular interactions responsible for facial developmentAJNR. American Journal of Neuroradiology 35:223–229.https://doi.org/10.3174/ajnr.A3453
-
Mutations in TMEM231 cause Joubert syndrome in French CanadiansJournal of Medical Genetics 49:636–641.https://doi.org/10.1136/jmedgenet-2012-101132
-
Identification of CC2D2A as a Meckel syndrome gene adds an important piece to the ciliopathy puzzleAmerican Journal of Human Genetics 82:1361–1367.https://doi.org/10.1016/j.ajhg.2008.05.004
-
The nonmotile ciliopathiesGenetics in Medicine 11:386–402.https://doi.org/10.1097/GIM.0b013e3181a02882
-
Hedgehog receptor function during craniofacial developmentDevelopmental Biology 415:198–215.https://doi.org/10.1016/j.ydbio.2016.02.009
Article and author information
Author details
Funding
National Institute of Dental and Craniofacial Research (R01DE029454)
- Jeremy F Reiter
National Institute of Arthritis and Musculoskeletal and Skin Diseases (R01AR054396)
- Jeremy F Reiter
National Institute of Dental and Craniofacial Research (F30DE024684)
- Shaun Abrams
The funders had no role in study design, data collection and interpretation, or the decision to submit the work for publication.
Ethics
This study was performed in strict accordance with the recommendations in the Guide for the Care and Use of Laboratory Animals of the National Institutes of Health. All mouse protocols were approved by the Institutional Animal Care and Use Committee (IACUC) of the University of California, San Francisco (protocol AN178683).
Copyright
© 2021, Abrams and Reiter
This article is distributed under the terms of the Creative Commons Attribution License, which permits unrestricted use and redistribution provided that the original author and source are credited.
Metrics
-
- 1,475
- views
-
- 230
- downloads
-
- 8
- citations
Views, downloads and citations are aggregated across all versions of this paper published by eLife.
Citations by DOI
-
- 8
- citations for umbrella DOI https://doi.org/10.7554/eLife.68558