A universal pocket in fatty acyl-AMP ligases ensures redirection of fatty acid pool away from coenzyme A-based activation
Abstract
Fatty acyl-AMP ligases (FAALs) channelize fatty acids towards biosynthesis of virulent lipids in mycobacteria and other pharmaceutically or ecologically important polyketides and lipopeptides in other microbes. They do so by bypassing the ubiquitous coenzyme A-dependent activation and rely on the acyl carrier protein-tethered 4′-phosphopantetheine (holo-ACP). The molecular basis of how FAALs strictly reject chemically identical and abundant acceptors like coenzyme A (CoA) and accept holo-ACP unlike other members of the ANL superfamily remains elusive. We show that FAALs have plugged the promiscuous canonical CoA-binding pockets and utilize highly selective alternative binding sites. These alternative pockets can distinguish adenosine 3′,5′-bisphosphate-containing CoA from holo-ACP and thus FAALs can distinguish between CoA and holo-ACP. These exclusive features helped identify the omnipresence of FAAL-like proteins and their emergence in plants, fungi, and animals with unconventional domain organizations. The universal distribution of FAALs suggests that they are parallelly evolved with FACLs for ensuring a CoA-independent activation and redirection of fatty acids towards lipidic metabolites.
Introduction
The ANL superfamily includes enzymes such as the acyl/aryl-CoA ligases (ACS or FACLs), adenylation domains (A-domains), and luciferases along with the recently identified fatty acyl-AMP ligases (FAALs) (Schmelz and Naismith, 2009). These enzymes are involved in the production of both primary metabolites such as acyl-CoA and secondary metabolites such as antibiotics (Sieber and Marahiel, 2005), complex lipids (Gokhale et al., 2007), cyclic peptides (Walsh, 2004), and lipopeptides (Hansen et al., 2007; Hemmerling et al., 2018). Basic metabolic pathways such as β-oxidation, membrane biogenesis, post-translational modifications, etc., use primary metabolites such as acyl-CoA. The secondary metabolites such as complex lipids that function as virulent molecules in Mycobacteria and bioactive molecules in several microbes that help tide over unfavorable conditions and establish themselves in their niches. Such diverse metabolites are produced by the members of the ANL superfamily through a two-step catalytic mechanism. It begins with the activation of carboxylate moiety containing substrates such as fatty acids or amino acids by adenosine triphosphate (ATP) hydrolysis and finally transferring it to an acceptor such as CoA or holo-ACP. Multiple structural and biochemical studies show that members of the superfamily such as FACLs and A-domains employ a common pocket for the chemically identical CoA and the 4'-PPant moieties attached to the holo-ACP, respectively, for the final transfer. It was later demonstrated that the A-domains can cross-react with CoA to form aminoacyl-CoA (Linne et al., 2007), which points to the liabilities of utilizing a common pocket for binding chemically identical moieties. Promiscuity towards the final acceptor has now been noted in different classes of ANL superfamily members where luciferases are shown to catalyze fatty acyl-CoA formation (Oba et al., 2003) and FACLs producing bioluminescence with molecular oxygen (Oba et al., 2009). While fatty acid/amino acid substrate promiscuity is well studied and exploited in combinatorial biosynthesis of bioactive molecules (Winn et al., 2020), the origin and basis of acceptor promiscuity is relatively less understood.
FAALs are atypical enzyme systems of the ANL superfamily as these show a narrow preference for holo-ACP and not CoA, where they transfer the activated fatty acyl-AMP to the 4'-PPant of holo-ACP (Trivedi, 2004). FAALs rejecting the small, diffusible, and abundant CoA while accepting the ACP-tethered to a 4'-PPant moiety (Figure 1—figure supplement 1a and b) is puzzling as they are chemically identical. In a previous study, it was proposed that the FAAL-specific insertion (FSI), an additional stretch of amino acids found only in the N-terminal domain of FAALs, prevents reaction with CoA (Arora, 2009). However, the deletion or destabilization of the FSI failed to convert FAALs as efficient producers of acyl-CoA as there is only a weak ability to react with CoA (Arora, 2009; Goyal et al., 2012). Moreover, it is also unclear how such a mechanism operates and distinguishes the two chemically identical acceptors, particularly when CoA is an abundant metabolite. These observations prompted us to hypothesize that FAALs have either evolved novel appendages or other modes for binding the acceptor to allow strict rejection of CoA.
In the present study, we have used structural, mutational, and biochemical analyses to identify the mechanistic basis of how FAALs can distinguish between near identical acceptors for the acyl transfer reaction. We show that, unlike other members of the superfamily, FAALs achieve acceptor fidelity by avoiding the usage of a promiscuous canonical CoA-binding pocket and utilizing a discriminatory pocket that is distinct from the canonical CoA-binding pocket. Loss- and gain-of-function mutations were generated by identifying the structural determinants that nullify the canonical CoA-binding pocket. Interestingly, we found that the non-functional canonical CoA-binding pocket and the unique discriminatory alternative pocket are unique features of FAALs, which is conserved in all forms of life including plant, fungi, and animals. The identification of such a conserved rejection mechanism across organisms has larger implications in determining the redirection of cellular flux of fatty acids towards synthesis of diverse metabolites across organisms.
Results and discussion
The promiscuous canonical CoA-binding pocket is inaccessible and redundant in FAALs
The structural features of a canonical CoA-binding pocket that allow the proper recognition of CoA/4'-PPant in different ANL superfamily members were compared and contrasted with the analogous structural positions in FAALs. A comprehensive analysis of the canonical CoA-binding pocket in the 26 structures (59 protomers) of the CoA/4'-PPant-bound ANL superfamily members (Supplementary file 1) revealed important aspects of CoA/4'-PPant recognition. It is observed that ligand interacts with the protein through three categories of interactions but none of the structures show the CoA/4'-PPant ligands bound in identical positions or orientations (Figure 1—figure supplement 2a and b). The three categories of interactions are (i) the hydrogen bonds mediated by the A8-motif of the C-terminal domain, (ii) the water-mediated contacts through the N-terminal helices H10 and H14 (notation based on Escherichia coli FAAL, abbreviated as EcFAAL; PDB: 3PBK) (Zhang et al., 2011), and (iii) the interactions with the phosphates (Gulick et al., 2003; Reger et al., 2007; Reger et al., 2008; Kochan et al., 2009; Mitchell et al., 2012; Li and Nair, 2015; Miller et al., 2016; Hughes and Keatinge-Clay, 2011) of the adenosine 3',5'-bisphosphate moiety through the positively charged residues (Arg/Lys) (Figure 1—figure supplement 3) in the loop connecting H23 and β26 (notation based on EcFAAL).
A comparative analysis of the structurally analogous CoA-binding pocket of FAALs (11 structures constituting 23 protomers) (Arora, 2009; Goyal et al., 2012; Zhang et al., 2011; Li et al., 2015; Guillet, 2016) sheds light on why FAALs cannot accept CoA. The analysis reveals an absence of selection from the positively charged residues (Arg/Lys) known to assist in CoA binding in the other members of the ANL superfamily (Figure 1a, Figure 1—figure supplement 3). In addition, the CoA-binding pocket of FAALs shows the presence of bulky residues F279, W224, and M231 (in EcFAAL) at the base and a unique FAAL-specific helix (FSH) (T252-R258 in EcFAAL) at the entrance of the pocket (Figure 1b). These elements are highly conserved in other FAALs (Figure 1c) and restrict the space available for the incoming 4'-PPant arm of both CoA and holo-ACP. The superposition of CoA- and 4'-PPant-bound structures with FAALs revealed that the overcrowded pocket along with the FSH at the entrance of the pocket is unlikely to accommodate either CoA or 4'-PPant of holo-ACP by hindering their entry (Figure 1b). There are at least seven atoms of the N-terminal domain of FAALs at a distance less than 2.5 Å from the atoms of CoA in its various conformations found in the different CoA-bound FACL structures. On the contrary, the N-terminal domain of FACLs shows only one atom at 2.5 Å from the multiple CoA conformations (Supplementary file 2). The distance-based assessment clearly indicates the potential clashes an incoming CoA would face in the canonical pocket of FAALs. Moreover, the absence of positively charged residues will naturally be unfavorable for CoA from being appropriately oriented in the pocket. These observations lead to the hypothesis that the canonical CoA-binding pocket is rendered non-functional because of the inaccessibility in FAALs.
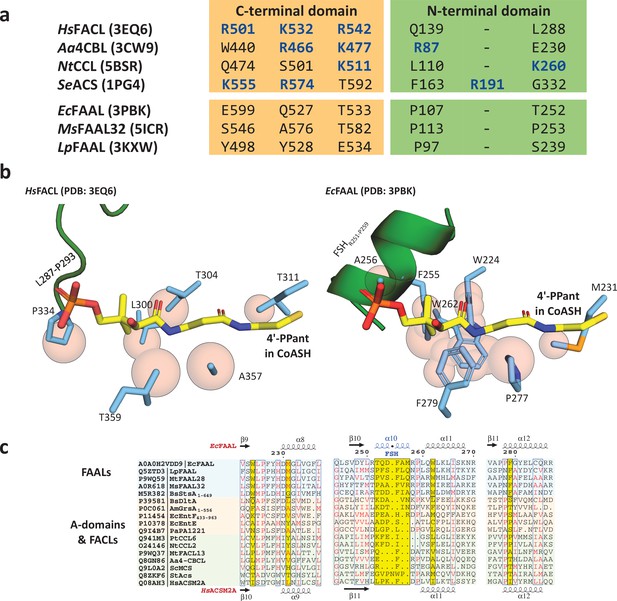
The presence of conserved negative selection elements and absence of positive selection elements in canonical coenzyme A (CoA)-binding pocket of fatty acyl-AMP ligases (FAALs) can prevent CoA binding.
(a) The CoA-interacting residues in fatty acyl/aryl-CoA ligases (FACLs) (HsFACL, Aa4CBL, NtCCL, and SeACS) and the structurally analogous residues in FAALs (EcFAAL, MsFAAL32, and LpFAAL) are tabulated. The positively charged residues (blue) mainly are from the C-terminal domain (orange) and occasionally from the N-terminal domain (green). (b) The residues (cyan) in the vicinity (≤2.5 Å) of the bound CoA (yellow; HsFACL; PDB: 3EQ6) are shown as van der Waals spheres (light red) to compare the canonical pocket in FAALs and FACLs. The adenosine 3',5'-bisphosphate moiety of CoA is omitted in the representation for clarity. A unique FAAL-specific helix (FSHR251-P259) at the entry of the canonical pocket shown in cartoon representation (green) is replaced by a loop (L287-P293) in FACLs. (c) The canonical pocket-obstructing features seen in FAALs (light cyan) are highlighted in yellow in the structure-based sequence alignment and compared to other representative members of the ANL superfamily (FACLs in light green and A-domains in light orange). The secondary structures of EcFAAL (PDB: 3PBK) and HsFACL (PDB: 3EQ6) are marked at the top and bottom of the alignment, respectively.
Resurrecting the canonical CoA pocket enables gain of function in FAALs
The structural analysis was used to design mutations in FAALs and FACLs where the bulkier residues of the canonical CoA-binding pocket of FAALs were mutated to smaller residues to induce ‘gain of function’ (Figure 2a and b). Likewise, the smaller residues in the canonical CoA-binding pocket of FACLs were substituted with the corresponding conserved bulkier residues present in FAALs to measure the ‘loss of function’ (Figure 2d and e). Individual mutations reducing the size of residues in the canonical CoA-binding pocket resulted in the production of acyl-CoA or ‘gain of function’ in FAALs that otherwise does not make any acyl-CoA (Figure 2c). A considerable amount of the total acyl-AMP formed was converted to acyl-CoA, ~80% in the case of MsFAAL32Δ254-257 and ~60% in the case of RsFAALΔ240-243, when the FSH segment was deleted as compared to their wild-type proteins, respectively. Some of the single-point mutants such as A253F in MtFACL13 show reduced production of acyl-CoA by ~80%, while mutations in combinations such as A276F/A232M of AfFACL reduce the turnover of acyl-AMP to acyl-CoA by 98% as compared to wild-type (100%) (Figure 2f). These observations clearly indicate that the size of residues in the canonical CoA-binding pocket dictates the ability to accommodate CoA and hence the ability to facilitate the thioesterification reaction with CoA.
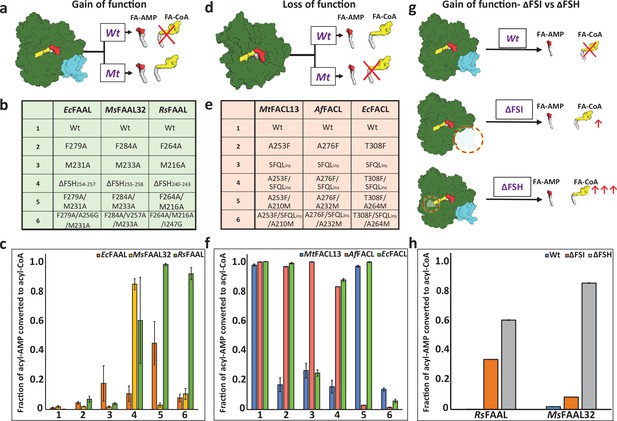
Biochemical analysis of ‘gain of function’ mutants of fatty acyl-AMP ligases (FAALs) and ‘loss of function’ mutants of fatty acyl/aryl-CoA ligases (FACLs).
(a) The biochemical activity of a wild-type (Wt) FAAL as compared to its mutants (Mt) is schematically represented. (b) Various ‘gain of function’ mutations of FAALs (EcFAAL, MsFAAL32, and RsFAAL) are tabulated as the following: row 1: wild-type protein; rows 2 and 3: single-point mutations; row 4: deletion of FAAL-specific helix (FSH); rows 5 and 6: combinations of these mutations. (c) The fraction of acyl-AMP converted to acyl-CoA by wild-type and various mutants (numbered on the x-axis according to b) of FAALs is represented as a bar graph along with standard error of the mean. (d) The biochemical activity of a wild-type (Wt) FACL as compared to its mutants (Mt) is schematically represented. (e) The various ‘loss of function’ mutations generated in FACLs (MtFACL, AfFACL, and EcFACL) are tabulated as the following: row 1: wild-type protein; row 2: single-point mutations; row 3: insertion of FSH; rows 4–6: combinations of these mutations. (f) The fraction of acyl-AMP converted to acyl-CoA by wild-type and various mutants (numbered on the x-axis according to e) of FACLs is represented as a bar graph along with standard error of the mean. (g) A comparison of the gain of function from ΔFSH mutation with ΔFSI mutation in FAALs is schematically represented. (h) The fraction of acyl-AMP converted to acyl-CoA by wild-type, ΔFSH, and ΔFSI mutants of RsFAAL and MsFAAL32 (represented on the x-axis) is represented as a bar graph along with standard error of the mean. The original uncropped images of these experiments are presented as Figure 2—source data 1. The intensity values used to compute the bar graphs are provided as an Excel file as the following: c = Figure 2—source data 2 f = Figure 2—source data 3; h = Figure 2—source data 4.
-
Figure 2—source data 1
The original uncropped radio-TLC images presented here were used to assess the ‘gain of function’ and ‘loss of function’ of fatty acyl-AMP ligases (FAALs) and fatty acyl/aryl-CoA ligases (FACLs) respectively following mutations in their canonical coenzyme A (CoA)-binding pocket.
It was also used to compare the gain of function obtained from deletion of the previously annotated FAAL-specific insertion (ΔFSI) and FAAL-specific helix (ΔFSH). Wild-type FAAL, wild-type FACL, and reaction lacking any protein were used as controls. All the TLCs were marked at the origin, where the reaction mix containing 1-14C fatty acids was spotted. The products acyl-CoA band and the acyl-AMP band along with the free fatty acid band are visualized owing to the radio-labeled fatty acid. (A) A representative image of TLC showing the canonical pocket mutations leading to the gain of function in EcFAAL. (B) A representative image of TLC showing the canonical pocket mutations leading to the gain of function in MsFAAL32. (C) A representative image of TLC showing the canonical pocket mutations leading to the gain of function in RsFAAL. (D) A representative image of TLC showing the canonical pocket mutations leading to the loss of function in MtFACL13. (E) A representative image of TLC showing the canonical pocket mutations leading to the loss of function in AfFACL. (F) A representative image of TLC showing the canonical pocket mutations leading to the loss of function in EcFACL. (G) A representative image of TLC showing the gain of function by ΔFSI mutation in RsFAAL. (H) A representative image of TLC showing the gain of function by ΔFSI mutation in MsFAAL32. Several mutations were generated in this study, which had multiple issues including protein stability, poor or complete loss of biochemical activity, etc., hence were not analyzed further and such mutations are marked by a red asterisk as ‘not part of the study.’ These original uncropped images of radio-TLC are source data for Figure 2.
- https://cdn.elifesciences.org/articles/70067/elife-70067-fig2-data1-v2.pdf
-
Figure 2—source data 2
The excel file is a tabulation of the raw values of the acyl-AMP and acyl-CoA formed in the gain-of-function experiments in EcFAAL, MsFAAL32, and RsFAAL, which are then converted to fraction of the total acyl-AMP (TA) formed to the remaining acyl-AMP (A) and the acyl-AMP converted to acyl-CoA (T).
These values were obtained from densitometric quantification of the TLC images, which were used to plot Figure 2c.
- https://cdn.elifesciences.org/articles/70067/elife-70067-fig2-data2-v2.xlsx
-
Figure 2—source data 3
The excel file is a tabulation of the raw values of the acyl-AMP and acyl-CoA formed in the loss-of-function experiments in MtFACL13, AfFACL, and EcFACL, which are then converted to fraction of the total acyl-AMP (TA) formed to the remaining acyl-AMP (A) and the acyl-AMP converted to acyl-CoA (T).
These values were obtained from densitometric quantification of the TLC images, which were used to plot Figure 2f.
- https://cdn.elifesciences.org/articles/70067/elife-70067-fig2-data3-v2.xlsx
-
Figure 2—source data 4
The excel file is a tabulation of the raw values of the acyl-AMP and acyl-CoA formed in the experiment comparing the gain of function in fatty acyl-AMP ligases (FAALs) with deletion of FAAL-specific insertion (ΔFSI) to deletion of FAAL-specific helix (ΔFSH).
The values are then converted to fraction of the total acyl-AMP (TA) formed to the remaining acyl-AMP (A) and the acyl-AMP converted to acyl-CoA (T).
These values were obtained from densitometric quantification of the TLC images, which were used to plot Figure 2h.
- https://cdn.elifesciences.org/articles/70067/elife-70067-fig2-data4-v2.xlsx
-
Figure 2—source data 5
The original uncropped radio-TLC showing ‘gain of function’ and ‘loss of function’ of FAALs and FACLs.
- https://cdn.elifesciences.org/articles/70067/elife-70067-fig2-data5-v2.zip
It was previously identified that deletion of FAAL-specific insertion (ΔFSI) can lead to gain of function in FAALs (Arora, 2009). Current results indicate that mutations in the canonical pocket alone are sufficient to introduce CoA production ability in FAALs. A comparison of acyl-CoA production of the ΔFSI with FSH deletion mutant (ΔFSH) (Figure 2g) reveals that RsFAALΔFSH produces 2-fold excess acyl-CoA as compared to RsFAALΔFSI while MsFAAL32ΔFSH produces 10-fold excess acyl-CoA as compared to MsFAAL32ΔFSI (Figure 2h). Therefore, it can be concluded that the FSH and other CoA- rejection elements around the canonical CoA-binding pocket significantly deter acyl-CoA production, which supersedes the inhibition of acyl-CoA production by FSI in FAALs. Overall, these observations lead to the conclusion that the available space in the canonical CoA-binding pocket of FAALs is very small to accommodate even the 4'-PPant moiety. Mutations that increase the pocket space enhance the acyl-CoA production in FAALs, while the opposite is observed in the case of FACLs. Therefore, the bulky residues in the putative canonical CoA-binding pocket of FAALs itself act as a negative selection gate to sterically exclude CoA. The rejection mechanism operational at the canonical CoA-binding pocket in FAALs is relatively more effective in rejecting CoA than the FSI-based rejection.
Identification of an alternative 4'-PPant-binding pocket in FAALs
The steric occlusion of the 4'-PPant binding in the canonical pocket would not only result in prevention of CoA-binding but also restrict the access to the 4'-PPant tethered holo-ACP. Previous studies have highlighted that FAALs work in coordination with holo-ACP’s, stand-alone or fused to polyketide synthase (PKS) or nonribosomal peptide synthetase (NRPS) modules, to produce various bioactive molecules such as complex lipids of Mycobacterium (Gavalda, 2009; Leger, 2009; Simeone, 2010; Trivedi, 2005; Vats, 2012), lipopeptides of Ralstonia (Spraker et al., 2016; Kreutzer, 2011; Kage et al., 2013), and other cyanobacterial species (Mares et al., 2014; Zhu et al., 2018; Edwards, 2004; Kleigrewe, 2015; Humbert, 2013). Since the canonical pocket is rendered inaccessible, it immediately prompts the question, ‘How then FAALs are able to transfer the activated fatty acids to the 4'-PPant arm of the holo-ACP?’ We, therefore, sought to identify if FAALs have evolved an alternative mechanism to accommodate the 4'-PPant arm to allow binding and subsequent catalysis. Analysis of the crystal structures of the N-terminal domains of FAALs using various pocket search algorithms such as MOLE 2.0 (Pravda, 2018), DOGSiteScorer (Volkamer et al., 2012; Fahrrolfes, 2017), PyVOL (Smith et al., 2019), KVFinder (Oliveira, 2014), and POCASA (Yu et al., 2010) helped us in identifying a novel cavity in the N-terminal domain of FAALs (Figure 3a) but not identifiable in any of the known crystal structures of FACLs.
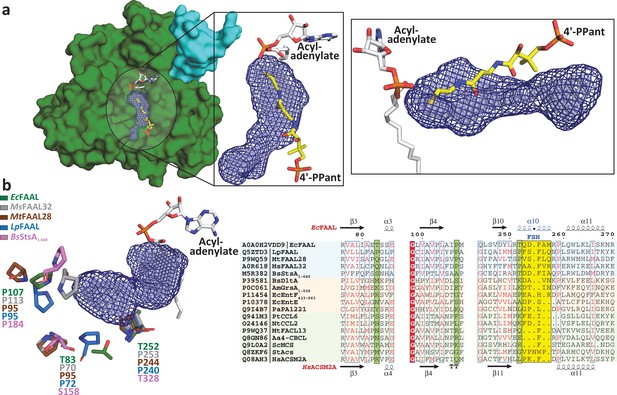
Fatty acyl-AMP ligases (FAALs) have an alternative pocket in the N-terminal domain distinct from the canonical coenzyme A (CoA)-binding pocket and lined by conserved prolines.
(a) The analysis of N-terminal domain of EcFAAL (PDB: 3PBK) (green: surface representation) with pocket finding algorithms identified a new pocket (dark blue: mesh representation; light blue: spheres representation). Also shown are the FAAL-specific insertion (cyan), an EcFAAL-bound lauryl-adenylate (gray: sticks representation) and the 4'-PPant moiety (yellow: sticks representation) of a CoA bound to HsFACL (PDB: 3EQ6) in the canonical pocket. The inset shows two different orientations (top view and lateral view) of the distinct alternative pocket poised against the acyl-adenylate and compared to the 4'-PPant of a CoA in canonical pocket. (b) Various residues (stick representation) of FAALs residing at the entrance of the unique pocket (dark blue: mesh representation; light blue: spheres representation) are identified through structural comparison. A structure-based sequence alignment of these residues (highlighted in pale green) with other representative members of the ANL superfamily reveals that FAALs have a higher frequency of prolines (occasionally Thr/Ser) than other members of the superfamily.
The entrance of the distinct tunnel is on the N-terminal side of the FSH, while the canonical pocket to accommodate CoA is on the C-terminal side of the FSH. The approach towards the active site in both cases is not on the same plane, but they coincide near the active site near the β-alanine of the 4'-PPant. The longest length along this pocket is aligned at ~25° to the canonical CoA-binding pocket. The canonical pocket is mainly formed after the rotation of the C-terminal domain in the thioesterification state (T-state) bringing the A8-motif near the active site. The space generated between the A8-motif (from the C-terminal domain) and the subdomain-B of the N-terminal domain constitutes the canonical CoA-binding pocket. In contrast, the newly identified pocket is the space between the loops in subdomain-A and the FSH region of subdomain-B of FAALs. These loop regions are highly variable in length but rich in prolines (occasionally threonine or serine) some of which are conserved in FAALs such as P226 and P107 in EcFAAL (Figure 3b), while the FSH has a unique secondary structure characteristic of FAALs. The structurally analogous sites in FACLs show a high degree of variability with occasional prolines, but the frequency of prolines at the indicated positions is poor, as compared to FAALs, and often replaced by Asn/Leu (Figure 3—figure supplement 1). The ability to consistently identify the tunnel with regions enriched in prolines around the opening led to the proposition that this alternative pocket is perhaps a universal attribute of FAALs that has evolved to accommodate the 4'-PPant arm.
The alternative pocket in FAALs is functional and accepts a 4'-PPant-tethered ACP
The ability of the alternative pocket to accommodate 4'-PPant arm tethered to ACP was tested using structure-guided mutagenesis. Bulkier residues (Phe/Arg) were introduced at the entrance of the tunnel that could potentially block the accessibility of the pocket for the incoming 4'-PPant arm. The biochemical analysis of these mutants requires an assay system to monitor the transfer of acyl-AMP to the 4'-PPant arm of holo-ACP. Typically, such acyl-transfers are assessed using SDS-PAGE (Trivedi, 2004; Kuhn, 2016) or conformationally sensitive urea-PAGE (CS-PAGE; Post-Beittenmiller et al., 1991). The ACPs that accept the acyl-chain from FAALs used in this study presented multiple complications such as poor conversion from apo-ACP to holo-ACP (Figure 4—figure supplement 1a) and lack of separation on a CS-PAGE (Figure 4—figure supplement 1b). Therefore, the CS-PAGE assay was modified for enhanced detection of the FAAL-dependent acyl-transfer on holo-ACP for the first time using the radiolabeled fatty acids. We tested the efficacy of the modified radio-CS-PAGE assay to probe three pairs of FAAL-ACP systems from diverse organisms (Figure 4a), viz., FAAL-ACP pairs from E. coli (EcFAAL-EcACP), Myxococcus xanthus (MxFAAL-MxACP), and Ralstonia solanacearum (RsFAAL-RsACP). The appearance of bright bands on the radio-CS-PAGE indicates that the radiolabeled fatty acid tethered to ACP, which is absent when apo-ACP is used, or ATP is omitted in the reaction. Thus, the assay system can enable the simultaneous probing of multiple FAAL-ACP pairs along with their mutations and facilitate similar studies in the future.
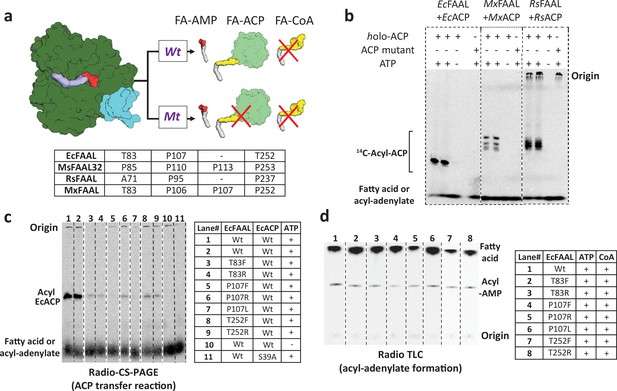
The alternative pocket identified in fatty acyl-AMP ligases (FAALs) is a functional pocket that assists in catalysis by accommodating 4'-PPant tethered to ACP.
(a) A schematic representation of the acyl-transfer function in a wild-type (Wt) FAAL is compared to a mutant protein (Mt) that blocks the alternative pocket. (b) A representative radio conformationally sensitive urea-PAGE (radio-CS-PAGE) gel shows the acyl-transfer reaction by wild-type FAALs on their respective holo-ACP. The radio-CS-PAGE gel shows the successful transfer of the activated fatty acyl-AMP to holo-ACP using three pairs of FAAL-ACP systems (EcFAAL-EcACP, MxFAAL-MxACP, and RsFAAL-RsACP), which is observed as a band due to the incorporated radiolabeled fatty acid. The absence of a band is indicative of failure to transfer as seen in the control reactions lacking ATP or the presence of a mutant ACP (conserved serine mutated to alanine) lacking the 4'-PPant arm. Samples are loaded at the point marked origin and the bands below contain either unreacted radiolabeled fatty acid or fatty acyl-AMP. (c) The radio-CS-PAGE shows that mutations of the residues lining the alternative pocket to Arg/Phe show a reduced or negligible acyl-ACP formation as the bands are diminished or absent. (d) A radio-TLC shows the acyl-AMP formation ability of various FAAL mutants indicated by the bright band in the center after the sample was loaded at the origin and developed until the edge of TLC where unused radiolabeled fatty acid is observed. The radio-TLC shows that loss of acyl-ACP formation is not due to affected acyl-adenylate formation. The original uncropped images of these experiments are presented as Figure 4—source data 1.
-
Figure 4—source data 1
The radio conformationally sensitive urea-PAGE (radio-CS-PAGE) images presented here were used to show the optimization of fatty acyl-AMP ligase (FAAL)-dependent acyl transfer on holo-ACP using three different FAAL-ACP systems (EcFAAL-EcACP, RsFAAL-RsACP, and MxFAAL-MxACP).
These were also used to assess the impact of mutations in the alternative pocket of EcFAAL to form acyl-EcACP. Wild-type FAAL, reaction lacking ATP, and reaction containing a mutant ACP, lacking 4'-phosphopantetheine arm, were used as controls. All the radio-CS-PAGE were marked at the origin, where the reaction mix containing 1-14C fatty acids was loaded without boiling. The acyl-ACP(s), along with unreacted fatty acids or acyl-AMP(s), could only be visualized owing to the radiolabeled fatty acid. The unreacted fatty acids or acyl-AMP(s) appeared as a diffused band at the bottom of the radio-CS-PAGE. The radio-TLC images presented here were used to assess the ability of EcFAAL and its mutations in the alternate pocket to form acyl-AMP. Wild-type EcFAAL, wild-type MtFACL13, and reaction lacking any protein were used as controls. All the TLCs were marked at the origin, where the reaction mix containing 1-14C fatty acids was spotted. The products, acyl-CoA band and the acyl-AMP band, along with the free fatty acid band, were visualized owing to the radiolabeled fatty acid. (A) A representative image showing the optimization and validation of the modified radio-CS-PAGE in three pairs of FAAL-ACP systems, namely EcFAAL-EcACP, RsFAAL-RsACP, and MxFAAL-MxACP. (B) A representative TLC image showing that alternate pocket mutations of EcFAAL have minimal or no effect on the acyl-AMP formation. (C) A representative image of radio-CS-PAGE showing that acyl-EcACP formation is severely attenuated by mutations in the alternate pocket of EcFAAL. Several mutations of the alternate pocket of EcFAAL were generated in this study, which had multiple issues including protein stability, poor or complete loss of biochemical activity, etc., hence were not analyzed further, and these mutations are marked by a red asterisk as ‘not part of the study.’ These original uncropped images of radio-CS-PAGE and radio-TLC are source data for Figure 4.
- https://cdn.elifesciences.org/articles/70067/elife-70067-fig4-data1-v2.pdf
The modified radio-CS-PAGE was then used to test if mutating prolines (occasionally a threonine) guarding the entrance of the pocket to bulkier residues can cause abrogation of the acyl-transfer activity (Figure 4c). It was found that even a single-point mutation, T83F or T83R, T252F or T252R, and P107F or P107R in EcFAAL, can almost abrogate the acyl-transfer reaction on holo-EcACP (Figure 4c). It should be noted that these mutations did not affect the adenylation ability of the proteins (Figure 4d). The mutations in other FAAL-ACP pairs, MxFAAL-MxACP (Figure 4—figure supplement 2a and b) and RsFAAL-RsACP (Figure 4—figure supplement 2c and d), also resulted in similar abrogation of the acyl-transfer ability on their respective cognate holo-ACPs. An additional FAAL-PKS pair of MsFAAL32-MsPKS131-1042 was also mutated and probed biochemically using the traditional radio-SDS-PAGE (Figure 4—figure supplement 2e and f). The mutations of residues guarding the alternative pocket to bulkier residues in the MsFAAL32-MsPKS131-1042 system also resulted in diminished acyl-transfer ability. These results indicate that blocking the entrance of the alternative pocket prevents the entry of the incoming 4'-PPant arm tethered to ACP. Therefore, in FAALs, the identified alternative pocket is fully functional and distinct from the non-functional canonical pocket. Such a unique pocket facilitates the entry of the 4'-PPant arm of the ACP to approach the active site and catalyze the acyl-transfer reaction, a feature absent in other members of the superfamily.
A universal mechanism for rejection of highly abundant CoA in the alternative pocket
The primary attribute of a functional alternative pocket in FAALs is to discriminate and reject CoA from the chemically identical 4'-PPant of holo-ACP. Therefore, the pocket should allow the entry of 4'-PPant into the tunnel but not the additional ‘head group,’ adenosine 3',5'-bisphosphate moiety, containing CoA. Structural analysis of eight CoA-bound protein structures (constituting 14 protomers) of the ANL superfamily reveals the variability or degrees of freedom available for adenosine 3',5'-bisphosphate moiety (head group). The analysis reveals that the 4'-PPant arm remains largely rigid in an extended form and the major source of variability is the conformational freedom around the 4'-phosphate (Figure 5—figure supplement 1a). Such a variability has previously been used to classify the conformations of CoA as extended conformations (e.g., SeACS, HsFACL) or bent conformations (e.g., Aa4'-CBL) (Chen, 2017). Limited variabilities in the conformations of the adenine ring and ribose are clustered in a small zone and therefore can be ignored (Figure 5—figure supplement 1a). The length of the 4'-PPant arm is around 18–20 Å (Leibundgut et al., 2007) while it is around 15–16 Å (Mitchell et al., 2012) as seen in the crystal structures of various ANL superfamily members, which is comparable to the length of predicted pockets (17–18 Å). The biochemical evidence (Figure 4c) along with length considerations indicates that the 4'-PPant arm can be accommodated within the predicted pocket. In the absence of structural information, the orientation of the 4'-PPant arm is random but limited to space with the identified pocket such that the 4'-phosphate is at the entry of the tunnel and the thiol near the active site.
Based on the above considerations, a CoA molecule in ANL superfamily members can be visualized as ‘flag hoisted on a mast.’ The extended 4'-PPant arm can be considered as the ‘mast’ and the adenosine 3',5'-bisphosphate moiety as the ‘flag.’ The rotation of the ‘flag’ around the ‘mast’ placed within the pocket is comparable to the degree of freedom available for the ‘flag’ (Figure 5—figure supplement 1b). We, therefore, rotated the ‘flag’ around the ‘mast’ to generate conformations at an angular sampling rate of 1° per conformation for each of the four known conformations of CoA (Figure 5a). It was found that main-chain atoms and Cβ atoms of the FAAL protein, irrespective of the conformation of the C-terminal domain (A-state or T-state), show an average of 28 clashes (van der Waals overlap >0.25 Å) (Figure 5b, Figure 5—figure supplement 2a). The adenosine 3',5'-bisphosphate or the ‘flag’ would encounter severe clashes because the entry of the alternative pocket is deeply embedded within the subdomain-A and -B of the N-terminal domain. It is unlike the entry of the canonical pocket, which is entirely open with the subdomain-B forming the base of the pocket (Figure 4). Therefore, the N-terminal domain of FAALs itself forms a strong deterrent for accommodating adenosine 3',5'-bisphosphate and hence prevents CoA binding (Figure 5—figure supplement 2b). Interestingly, the structural elements resisting the adenosine 3',5'-bisphosphate are unique to FAALs such as the FSH (T252-R258 in EcFAAL) and the loop harboring proline residues that guard the opening of the tunnel. Therefore, a strong negative selection and the absence of any positive selection make accommodation of adenosine 3',5'-bisphosphate containing CoA unlikely. In contrast, the adenosine 3',5'-bisphosphate lacking 4'-PPant of holo-ACP can easily access the pocket and participate in the acyl-transfer reaction. This essentially forms the structural basis for the CoA-rejection mechanism in FAALs (Figure 5c).
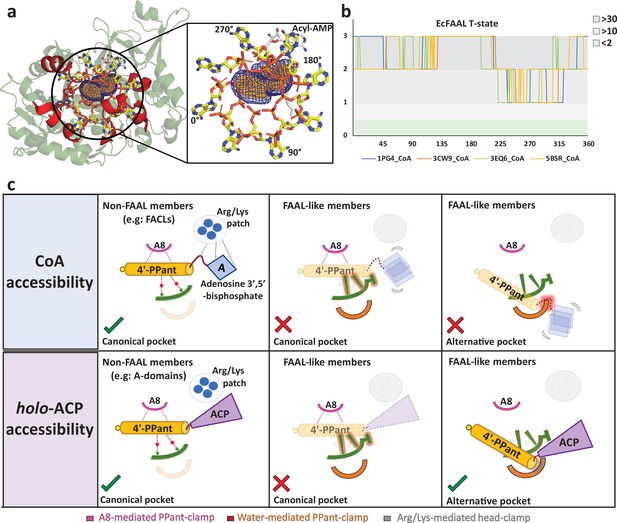
The alternative pocket in fatty acyl-AMP ligases (FAALs) is highly selective, and its unique architecture negatively selects for coenzyme A (CoA).
(a) A ‘mast’ (the 4’-PPant arm) is aligned along the longest length of the predicted pocket (blue: mesh representation) and the ‘flag’ (adenosine 3',5'-bisphosphate) is rotated to generate theoretical orientations of the ‘flag.’ These conformations of HsFACL (PDB: 3EQ6) are shown (yellow: stick format) at 45° intervals. The regions of the EcFAAL (cartoon representation) showing clashes are highlighted (red). (b) The count of atoms (C, N, O, Cα, and Cβ) of EcFAAL showing van der Waals short contacts (≤0.25 Å) with the conformations of the ‘flag’ is scored (short contacts ≥30 = 3; ≥10 = 2; ≥2 = 1 and <2 = 0). These scores (y-axis) are plotted against the specific rotation angles of the conformation, where the short contact is observed (x-axis). (c) The universal CoA-rejection mechanism is schematically summarized. The 4’-PPant (light orange) of both CoA and holo-ACP (purple) bind to the canonical pocket of non-FAAL members through multiple interactions (dotted lines). Interactions between Arg/Lys patch (circle: blue) and the adenosine 3',5'-bisphosphate moiety (rhombus: blue) acts as positive selection. The negative selection elements of the canonical pocket clash (highlighted in red) with the adenosine 3',5'-bisphosphate in the alternative pocket. The absence of Arg/Lys patch (dotted circle) fails to provide stability to the adenosine 3',5'-bisphosphate moiety (dotted rhombus; blue). The 4'-PPant (yellow) tethered to ACP (purple) is only accepted in the alternative pocket, which is absent in non-FAAL members, as it shows no clashes. The ranked count of atoms used to generate the plot 5b is provided as an Excel file, Figure 5—source data 1.
-
Figure 5—source data 1
The Excel sheet tabulates the number of clashes observed between various conformations of coenzyme A (CoA) and fatty acyl-AMP ligases (FAALs) (main-chain atoms: N, C, O, Cα, and Cβ).
The bound CoA from the crystal structures of SeACS (PDB: 1PG4), AaCBCL (PDB: 3CW9), HsFACL (PDB: 3EQ6), and NtCCL (PDB: 5BSR) were used for the starting conformation of the head group of CoA. These crystal structures were then superimposed over the crystal structures of representative FAALs in adenylation competent state (A-state), EcFAAL (PDB: 3PBK), and MsFAAL32 (PDB: 5ICR), which were also used to generate the thioesterification competent state (T-state) by superimposing the C-terminal domain separately. The number of clashes was computed for every 1° rotation of the head group and then ranked as 3 if number ≥30, as 2 if number ≥10, and as 0 if number ≥1. These ranked values were used to plot Figure 5b and Figure 5—figure supplement 2a.
- https://cdn.elifesciences.org/articles/70067/elife-70067-fig5-data1-v2.xlsx
Identification of FAAL-like proteins across different forms of life
Previous studies show that FAALs can be identified based on the presence of insertion and its anchorage to the N-terminal domain through hydrophobic interactions. A lack of sequence conservation in the FSI and only a single structural template for further analysis complicated the search procedure. Therefore, early genome mining efforts resulted in a sparse identification of FAALs in lineages of actinobacteria, cyanobacteria, and proteobacteria along with some eukaryotes such as humans and mouse (Goyal et al., 2012). The conserved sequence features from this study such as FSH, a blocked canonical pocket, and a proline-lined 4'-PPant binding pocket led to the identification of FAAL-like proteins with greater confidence. The analysis revealed a ubiquitous distribution of FAALs across different forms of life including bacteria, plants, fungi, and animals, except archaea (Figure 6a). The phylogenetic analysis reveals that all the FAAL-like proteins diverge and cluster away from FACLs or A-domains. FAAL-like proteins show three subgroups, viz., bacterial/plant FAALs group, fungal FAAL-like protein group, and animal FAAL-like protein group (Figure 6a). Typical FAALs are bacterial FAALs, which are always found in a genomic context with PKS and PKS/NRPS hybrid genes either as a stand-alone domain or as a didomain fused to ACP or multidomain fused to the entire PKS/NRPS gene (Supplementary file 3). Occasionally, the stand-alone bacterial FAALs are found to be interspersed with additional domains (Figure 6—figure supplement 1a), mainly with dehydrogenases and oxygenases. Most plant FAAL-like domains resemble the bacterial FAALs from their sequence identity and domain organization. However, unique domain organizations are also found in plant FAAL-like domains, where an uncharacterized protein has FAAL-like domains sandwiched between HemY domains and catalase domains or amino acid oxidase domains (Figure 6—figure supplement 1a). To the best of our knowledge, this is the first report of the presence of FAAL-like domains in plants and also the fusion of alternate domains to FAALs at their N-terminus.
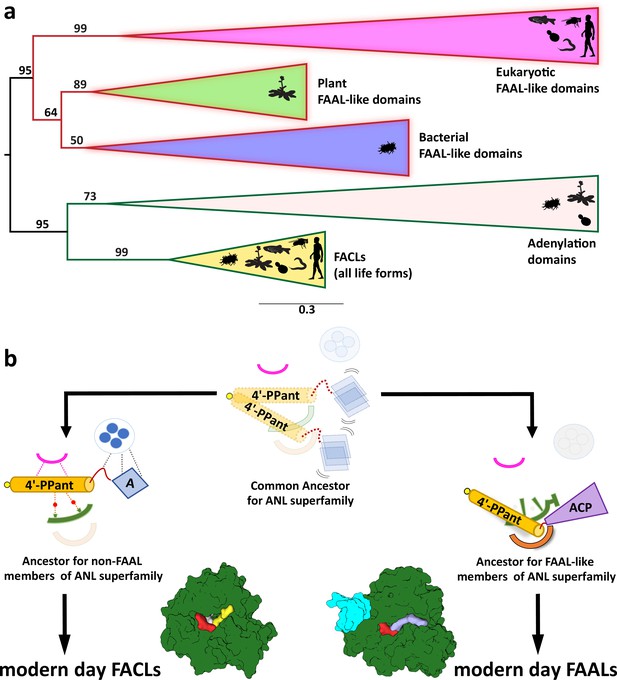
The coenzyme A (CoA)-rejection elements in fatty acyl-AMP ligases (FAALs) are conserved in all forms of life, and therefore, FAALs and fatty acyl/aryl-CoA ligases (FACLs) have parallelly evolved from a common ancestor of the ANL superfamily.
(a) A clustering diagram with the bootstrapping values for all the FAAL-like sequences is presented. The ANL superfamily members have two major divergent classes, viz., CoA-rejecting FAAL-like sequences and CoA-accepting non-FAAL members (FACLs and A-domains). A graphical representation of the distribution of these members is shown using pictorial representation of the organisms, which include bacteria, plants, yeast, worm, fly, and human. (b) The ancestral fold of the ANL superfamily may have been a loose organization of peptide scaffolds working with thiol-containing molecules such as pantetheine, etc. The ancestral fold accumulated various mutations, resulting into the ancestor of modern-day acceptor promiscuity lacking FAAL-like forms and acceptor promiscuity containing non-FAAL members. The evolution of acceptor promiscuity spectrum may have been driven based on their participation in bulk metabolic reactions. FAALs did not participate in bulk metabolic reactions and hence dedicated themselves to their cognate ACP partners to redirect small molecules to specific pathways. The non-FAAL members of the ANL superfamily such as FACLs participate in bulk reactions, where minor cross-reaction products are observed.
Fungal and animal FAAL-like domains tend to cluster together with bacterial/plant FAAL-like domains in bioinformatic analysis (Figure 6a, Figure 6—figure supplement 1b) because of the conservation of unique FAAL-specific features. Despite their lower sequence identity, the FSI (Figure 6—figure supplement 1c and d) supported by a hydrophobic patch and the FSH and CoA-rejecting features (Figure 6—figure supplement 2a and b) are clearly identifiable. Such conserved FAAL-specific features indicate that FAAL-like modules are recruited in eukaryotes for specific metabolic processes owing to their unique ability to reject CoA. Interestingly, fungal and animal FAAL-like domains share a highly conserved three-domain architecture, and such an architecture is not seen in any of the prokaryotes and plants (Supplementary file 4). The conserved three-domain protein consists of a N-terminal DMAP1-binding domain followed by a tandemly fused two FAAL-like domains as found in fungi to animals. The eukaryotic metabolic context for avoiding a reaction with CoA through these FAAL-like domains and their unique domain organization remains to be explored. Based on these observations, FAAL-like domains are more widespread than previously anticipated and their omnipresence is comparable to the universally present FACLs. Therefore, we propose that FAALs may not have descended from FACLs and they rather share a parallel evolutionary history. The ancestral ANL fold could have diverged as non-promiscuous FAAL-like members and promiscuous nonFAAL-like members simultaneously in the last universal common ancestor (Figure 6b).
Conclusions
The study uncovers the mechanistic basis of how FAALs strictly reject CoA, which is highly abundant and almost chemically identical to their actual substrate, holo-ACP. The rejection mechanism relies on a discriminatory 4'-PPant-accepting pocket while avoiding the promiscuous canonical CoA-binding pocket that is rendered non-functional. It has been achieved through bulky hydrophobic residues in the pocket and a unique secondary structural element, FSH, at the entrance. The discriminatory 4'-PPant-accepting pocket in FAALs, on the other hand, has a unique architecture that negatively selects adenosine 3',5'-bisphosphate moiety and also lacks Arg/Lys residues for positive selection. Interestingly, these rejection criteria are not only conserved in bacteria but also in all forms of life (excluding archaea). The unique remodeling of pockets to ensure acceptor discrimination probably allowed the evolutionary recruitment of FAAL-like domains in metabolic crossroads for redirecting the fate of molecules to specific pathways. Such a widespread conservation of FAAL-like proteins puts the evolutionary origin of FAALs parallel with FACLs in the last universal common ancestor and not as a subset of FACLs.
The scaffold of the ANL superfamily of enzymes is known to be promiscuous not only for the substrates they act on (Arora et al., 2005), but also the final acceptor of the acyl adenylates. Surprisingly, billions of years of evolution have not prevailed upon the substrate-promiscuity problem as well as the acceptor-promiscuity problem. The probability of acceptor promiscuity influencing the erroneous product formation is high as some of the acceptors such as CoA and pantetheine are the most abundant molecules in the cell. The problem is greatly amplified as it can redirect the fate of metabolites from one pathway to another such as primary metabolism to secondary metabolism. Our analysis shows that the CoA-bound structures of ANL superfamily members exhibit non-conformity in the binding mode of 4'-PPant or the adenosine 3',5’-bisphosphate moiety (Reger et al., 2008; Zahn, 2019). The variability in the 4'-PPant binding is also true for the A-domain:ACP complexes (Mitchell et al., 2012; Sundlov et al., 2012; Sundlov and Gulick, 2013). The variability could be resulting from highly divergent residues lining the CoA-binding site in FACLs and their analogous sites in FAALs (Supplementary file 5), which possibly explains the observed differential activity of certain loss-of-function and gain-of-function mutations (Supplementary file 5). Hence, it is evident that a defined CoA-binding pocket is lacking in ANL superfamily members, which is commensurate with the failure to identify the pocket in FACLs using various pocket search algorithms. Therefore, a promiscuous access to the active site without any selection determinants is the likely cause for the lack of discrimination for final acceptors in these enzymes.
It is not clear if the observed spectrum of latent activities in these enzymes’ design is an evolutionary relic or has any physiological relevance in a specific cellular context. The persistence of acceptor promiscuity can only have two explanations: viz., the cross-reaction products are beneficial, or pocket modification is not possible without compromising the basic function. In this context, FAALs are surprisingly high-fidelity enzymes representing the extreme end of the promiscuity spectrum, offering no cross-reaction with CoA as acceptors of the acyl adenylates (Trivedi, 2004; Arora, 2009; Goyal et al., 2012; Priyadarshan and Sankaranarayanan, 2018). A functional and discriminatory 4'-PPant binding in FAALs has established them as an alternative enzymatic bridge between FA synthesis, exogenous fatty acids import, and PKS/NRPS machinery that produce diverse bioactive natural products such as bacillomycin (Lu, 2019; Koumoutsi, 2004), mycosubtilin (Duitman, 1999), daptomycin (Wittmann et al., 2008; Baltz, 2021), phenylnannolone (Bouhired, 2014), alkylresorcylic acid (Hayashi et al., 2011), caryoynencin (Ross et al., 2014), vioprolide (Auerbach et al., 2018), taromycin A (Yamanaka, 2014), tambjamine (Marchetti et al., 2018), ambruticin (Hemmerling et al., 2018; Julien et al., 2006), , to name a few (Supplementary file 3). The relevance of the lack of acceptor promiscuity in FAALs has been demonstrated in Mycobacteria as being responsible for dictating the fate of free fatty acids in producing virulent lipids (Arora, 2009).
The conserved sequence and structural features of bacterial FAALs enabled better identification of FAAL-like domains across all forms of life, except archaea. FAALs may have been recruited to specifically ‘load’ fatty acids on PKS/NRPS but the possibility of lack of promiscuity in FAALs driving their over-representation cannot be ignored. Their characteristic absence in archaea perhaps can be explained by the abysmal frequency or absence of PKS or NRPS systems. FAAL-like domains of bacteria and plants exhibit remarkable sequence similarity, which is expected because of the presence of canonical PKS/NRPS enzymology in both systems. A few of the identified FAAL-like domains in bacteria show unique domain organizations such as a fusion with lysophospholipid acyltransferases and alpha aminoadipate reductases or found interspersed in operons with dehydrogenases, decarboxylase, and ketoacyl synthases. Unique domain architectures are also found in plants, where FAAL-like domains are sandwiched between HemY and catalase domains and the function of these conserved proteins remain unknown. These unique architectures are evolutionary instances of the recruitment of FAAL-like domains for PKS/NRPS-independent functions.
Interestingly, unique domain architectures are found in FAAL-like domain-containing proteins of fungi and animals, the majority of which are largely PKS/NRPS-free systems. In these organisms, two tandemly fused FAAL-like domains are found at the C-terminus and a DMAP-1-binding domain at the N-terminus of a highly conserved protein. These proteins are annotated and characterized as the virulent factor CPS1 in few fungal pathogens such as Magnaporthe oryzae (Wang, 2016), Cochliobolus heterostrophus (Lu, 2003), and Coccidioides posadasii (Narra, 2016). Therefore, they are proposed as a target for potential fungicides and as a vaccine candidate in plant and animal pathogens, respectively (Wang, 2016; Lu, 2003; Narra, 2016). In invertebrates and vertebrates, these are known as DIP2 and are important for proper axon bifurcation and guidance, suggesting their physiological importance (Nitta et al., 2017; Noblett, 2019). Recently, we showed that the mice lacking one of the eukaryotic FAAL-like proteins, DIP2A, show a diet-dependent growth anomaly such as obesity pointing to their importance in eukaryotic lipid metabolism (Kinatukara, 2020). The precise molecular and biochemical details of these proteins are yet to be elucidated. Given the extent of sequence divergence in various motifs of eukaryotic FAAL-like domains (Figure 6—figure supplement 3a–d), it is difficult to predict the processes they may be involved in. However, the presence of several elements of the CoA-rejection mechanism and their close identity to FAALs allow us to put forth the hypothesis that eukaryotic FAAL-like domains were recruited for their high acceptor-fidelity property. It is also possible that these divergences have some functional relevance in the context of eukaryotic metabolism, which may represent an additional member in the spectrum of biochemical activities represented in the ANL superfamily.
The current work identifying FAAL-like enzymology using a universal CoA rejection mechanism may form the platform for further studies to delineate why they have been recruited in fungal and animal systems. The study provides new structural and sequence attributes to confirm the identity of FAALs, many of which remain misannotated and uncharacterized. The study opens new avenues in combinatorial engineering of PKS/NRPS by using FAALs as a unique module to load fatty acids or use preexisting knowledge to engineer them to load unique molecules (Clark et al., 2018) with exceptional fidelity. Thus, FAALs can be exploited to produce novel bioactive molecules by virtue of their unique acceptor-fidelity property.
Materials and methods
Cloning, expression, and purification of proteins
Request a detailed protocolEcFAAL (A0A0H2VDD9), EcACP (A0A2X1NC35), EcFACL (P69451), MsFAAL32 (A0R618), MsPKS13 (A0R617; 1–1042 residues), RsFAAL (Q8XRP4), RsACP (Q8XRP0), AfFACL (O30147), MxFAAL (Q1CXX0), MxACP (Q1CXW9), and MtFACL13 (P9WQ37) were amplified by PCR from their respective genomic DNA using Phusion polymerase (Thermo Scientific) using specific primers (Supplementary file 6). These genes were expressed as hexahistidine-tagged proteins after the induction with IPTG using the E. coli BL21(DE3) expression system. The mutants were generated using quick-change site-directed mutagenesis. All the proteins including mutants were expressed and purified to homogeneity using Ni-NTA affinity chromatography followed by size-exclusion chromatography at 4°C. They were flash-frozen in liquid nitrogen and stored at –80°C until further use.
Biochemical analysis of FAALs and FACLs
Request a detailed protocolThe acyl-AMP and acyl-CoA formation by FAALs and FACLs was performed by previously described methods (Trivedi, 2004). Briefly, the reaction was carried out in a 15 μL reaction volume with 50 mM Tris (pH 8.0) containing 8 mM MgCl2, 176 μM fatty acid, and 24 μM 1–14C fatty acid. Lauric acid (C12) was used for all enzymes except MsFAAL32 where palmitic acid (C16) was used as the substrate. 2 mM CoASH was either included or excluded from the reaction according to the reaction process being assessed. 2 mM ATP was used to initiate the reaction and incubated at 30°C for 15 min on the water bath. The reaction was quenched using 5 μL of 10% acetic acid. The entire 20 μL reaction mixture was directly spotted on a silica gel 60 F254 TLC plate and allowed to dry for 2 hr. Subsequently the products were resolved using n-butanol/acetic acid/water (80:25:40; v/v) solvent system at 4°C. All FACLs (MtFACL13, EcFACL, AfFACL), EcFAAL and MsFAAL32 were used at 5 μM concentration while MxFAAL and RsFAAL were used at 7.5 μM. The 14C-fatty acids allowed detection of the products on a phosphorimager (Amersham Typhoon FLA 9000), which were then quantified by densitometry using Image Lab (Bio-Rad Laboratories Inc). All experiments were performed as triplicates. The percentage of acyl-AMP converted to acyl-CoA from the total acyl-AMP formed is used to plot and compare the activity, along with the standard error of the mean, of wild-type against the respective mutants.
Conversion of apo-ACP to holo-ACP
Request a detailed protocolAll the purified ACPs were converted to holo-ACP before being flash-frozen using previously described protocols (Lambalot, 1996). Briefly, after Ni-NTA purification, they were buffer exchanged to the phosphopantetheinylation buffer (20 mM Tris pH 8.8, 10 mM MgCl2, and 10 mM dithiothreitol). Approximately 250 μM of ACP was then incubated with 1.25 μM of a non-specific phosphopantetheinyl transferase Sfp from B. subtilis in a 300 μL reaction mixture along with 10 mM DTT, 10 mM MgCl2, and 1 mM CoASH. The reaction mixture was incubated at 25°C for 12–16 hr and further purified using size-exclusion chromatography at 4°C. The holo-ACP was flash-frozen in liquid nitrogen and stored at –80°C until further use. The conserved serine on which the 4'-PPant moiety is post-translationally modified was mutated to alanine, and the resulting protein was used as apo-ACP for all assays.
Loading-activated acyl chains on holo-ACP by FAALs
Request a detailed protocolThe transfer of activated acyl-chain to stand-alone holo-ACP by FAALs was assessed using radiolabeled fatty acids combined with CS-PAGE (Post-Beittenmiller et al., 1991). The following ratio of FAAL to holo-ACP was used: 1 μM of EcFAAL with 20 μM of EcACP, 4 μM of MxFAAL with 8 μM of MxACP, 5 μM of RsFAAL with 20 μM RsACP and 1 μM of MsFAAL32 with 12.5 μM of MsPKS13∆C. These proteins were incubated in a typical 15 μL reaction mixture composed of 50 mM HEPES pH 7.2, 5 mM MgCl2, 0.01% w/v Tween-20, 0.003% DMSO, 2 mM ATP, and 9 μM of 14C labeled-lauric acid (C12). The reactions of MxFAAL-MxACP and RsFAAL-RsACP consisted of 20 mM Tris pH 8.0 and lacked DMSO. The reaction mixture was incubated for 2 hr at 30°C and quenched with an equal volume of urea loading dye (25 mM Tris pH 6.8, 1.25 M urea, 20% glycerol, and 0.08% bromophenol blue). The contents were immediately loaded and separated on a 15% PAGE containing 2.5 M urea. The acyl-transfer on holo-MsPKS131-1042 by MsFAAL32 was carried out as previously described (Kuhn, 2016). Briefly, 14C labeled-palmitic acid (C16) was used to assess the transfer on holo-MsPKS131-1042 on an 8% SDS-PAGE. All gels were then dried using a gel drier (Bio-Rad gel dryer 583) and the radiolabeled acyl-ACP was detected using the phosphorimager (Amersham Typhoon FLA 9000).
Sequence and structural analysis
Request a detailed protocolAll sequences were identified and retrieved from the NCBI sequence database using EcFAAL as template and BLAST search algorithm. A structure-based sequence alignment was generated using msTALI (Shealy and Valafar, 2012) and then other sequences were added to the alignment in MAFFT (Katoh et al., 2019). The sequence alignments were rendered using ESPript (Robert and Gouet, 2014). The phylogenetic analysis of these sequences was carried out using both neighbor-joining as implemented in MAFFT (Katoh et al., 2019) and maximum-likelihood as implemented in IQTREE (Nguyen et al., 2015; Hoang et al., 2018; ) using default parameters. The phylogenetic tree was rendered in MixtureTree Annotator (Chen and Ogata, 2015). A graphical representation of the multiple sequence alignment was converted to sequence logo using WebLogo (Crooks et al., 2004). Images of various organisms were reusable silhouette images of organisms obtained from Phylopic (https://www.phylopic.org) under Creative Commons license. All structural analyses including structural superposition, van der Waals distance measurements, and ligand alignment were carried out with various in-built features of PyMOL (Schrödinger, LLC, 2015). All cavity search programs were run using default parameters.
Data availability
All data generated or analyzed during this study are included in the manuscript and supporting files. Source data files have been provided for Figure 2, Figure 4, Figure 4—figure supplement 1 and Figure 4—figure supplement 2.
References
-
Promiscuous fatty acyl CoA ligases produce acyl-CoA and acyl-SNAC precursors for polyketide biosynthesisJournal of the American Chemical Society 127:9388–9389.https://doi.org/10.1021/ja052991s
-
Mechanistic and functional insights into fatty acid activation in Mycobacterium tuberculosisNature Chemical Biology 5:166–173.https://doi.org/10.1038/nchembio.143
-
Characterization of an Unusual Glycerate Esterification Process in Vioprolide BiosynthesisACS Chemical Biology 13:3123–3130.https://doi.org/10.1021/acschembio.8b00826
-
Genome mining for drug discovery: cyclic lipopeptides related to daptomycinJournal of Industrial Microbiology & Biotechnology 48:kuab020.https://doi.org/10.1093/jimb/kuab020
-
Crystal structure of the thioesterification conformation of Bacillus subtilis o-succinylbenzoyl-CoA synthetase reveals a distinct substrate-binding modeThe Journal of Biological Chemistry 292:12296–12310.https://doi.org/10.1074/jbc.M117.790410
-
ProteinsPlus: a web portal for structure analysis of macromoleculesNucleic Acids Research 45:W337–W343.https://doi.org/10.1093/nar/gkx333
-
The Pks13/FadD32 crosstalk for the biosynthesis of mycolic acids in Mycobacterium tuberculosisThe Journal of Biological Chemistry 284:19255–19264.https://doi.org/10.1074/jbc.M109.006940
-
Versatile polyketide enzymatic machinery for the biosynthesis of complex mycobacterial lipidsNatural Product Reports 24:267–277.https://doi.org/10.1039/b616817p
-
Insight into Structure-Function Relationships and Inhibition of the Fatty Acyl-AMP Ligase (FadD32) Orthologs from MycobacteriaThe Journal of Biological Chemistry 291:7973–7989.https://doi.org/10.1074/jbc.M115.712612
-
The loading module of mycosubtilin: an adenylation domain with fatty acid selectivityJournal of the American Chemical Society 129:6366–6367.https://doi.org/10.1021/ja070890j
-
UFBoot2: Improving the Ultrafast Bootstrap ApproximationMolecular Biology and Evolution 35:518–522.https://doi.org/10.1093/molbev/msx281
-
Structural snapshots for the conformation-dependent catalysis by human medium-chain acyl-coenzyme A synthetase ACSM2AJournal of Molecular Biology 388:997–1008.https://doi.org/10.1016/j.jmb.2009.03.064
-
Biosynthesis of a complex yersiniabactin-like natural product via the mic locus in phytopathogen Ralstonia solanacearumApplied and Environmental Microbiology 77:6117–6124.https://doi.org/10.1128/AEM.05198-11
-
A new enzyme superfamily - the phosphopantetheinyl transferasesChemistry & Biology 3:923–936.https://doi.org/10.1016/s1074-5521(96)90181-7
-
A comprehensive genomic and growth proteomic analysis of antitumor lipopeptide bacillomycin Lb biosynthesis in Bacillus amyloliquefaciens X030Applied Microbiology and Biotechnology 103:7647–7662.https://doi.org/10.1007/s00253-019-10019-6
-
The carbon chain-selective adenylation enzyme TamA: the missing link between fatty acid and pyrrole natural product biosynthesisOrganic & Biomolecular Chemistry 16:2735–2740.https://doi.org/10.1039/c8ob00441b
-
Structures of a Nonribosomal Peptide Synthetase Module Bound to MbtH-like Proteins Support a Highly Dynamic Domain ArchitectureThe Journal of Biological Chemistry 291:22559–22571.https://doi.org/10.1074/jbc.M116.746297
-
A Coccidioides posadasii CPS1 Deletion Mutant Is Avirulent and Protects Mice from Lethal InfectionInfection and Immunity 84:3007–3016.https://doi.org/10.1128/IAI.00633-16
-
IQ-TREE: a fast and effective stochastic algorithm for estimating maximum-likelihood phylogeniesMolecular Biology and Evolution 32:268–274.https://doi.org/10.1093/molbev/msu300
-
DIP-2 suppresses ectopic neurite sprouting and axonal regeneration in mature neuronsThe Journal of Cell Biology 218:125–133.https://doi.org/10.1083/jcb.201804207
-
In vivo pools of free and acylated acyl carrier proteins in spinach. Evidence for sites of regulation of fatty acid biosynthesisThe Journal of Biological Chemistry 266:1858–1865.https://doi.org/10.1016/S0021-9258(18)52372-3
-
MOLEonline: a web-based tool for analyzing channels, tunnels and pores (2018 updateNucleic Acids Research 46:W368–W373.https://doi.org/10.1093/nar/gky309
-
Fatty Acyl-AMP Ligases as Mechanistic Variants of ANL Superfamily and Molecular Determinants Dictating Substrate SpecificitiesJournal of the Indian Institute of Science 98:261–272.https://doi.org/10.1007/s41745-018-0084-2
-
Deciphering key features in protein structures with the new ENDscript serverNucleic Acids Research 42:W320.https://doi.org/10.1093/nar/gku316
-
The molecular basis of conjugated polyyne biosynthesis in phytopathogenic bacteriaAngewandte Chemie 53:7794–7798.https://doi.org/10.1002/anie.201403344
-
Adenylate-forming enzymesCurrent Opinion in Structural Biology 19:666–671.https://doi.org/10.1016/j.sbi.2009.09.004
-
SoftwareThe PyMOL Molecular Graphics SystemVersion 1.8.
-
Multiple structure alignment with msTALIBMC Bioinformatics 13:105.https://doi.org/10.1186/1471-2105-13-105
-
Structure determination of the functional domain interaction of a chimeric nonribosomal peptide synthetase from a challenging crystal with noncrystallographic translational symmetryActa Crystallographica. Section D, Biological Crystallography 69:1482–1492.https://doi.org/10.1107/S0907444913009372
-
Retrobiosynthetic approach delineates the biosynthetic pathway and the structure of the acyl chain of mycobacterial glycopeptidolipidsThe Journal of Biological Chemistry 287:30677–30687.https://doi.org/10.1074/jbc.M112.384966
-
Harnessing and engineering amide bond forming ligases for the synthesis of amidesCurrent Opinion in Chemical Biology 55:77–85.https://doi.org/10.1016/j.cbpa.2019.12.004
-
Role of DptE and DptF in the lipidation reaction of daptomycinThe FEBS Journal 275:5343–5354.https://doi.org/10.1111/j.1742-4658.2008.06664.x
-
Structural and functional studies of fatty acyl adenylate ligases from E. coli and L. pneumophilaJournal of Molecular Biology 406:313–324.https://doi.org/10.1016/j.jmb.2010.12.011
-
Terminal Olefin Profiles and Phylogenetic Analyses of Olefin Synthases of Diverse Cyanobacterial SpeciesApplied and Environmental Microbiology 84:e00425-18.https://doi.org/10.1128/AEM.00425-18
Article and author information
Author details
Funding
Department of Biotechnology, Ministry of Science and Technology, India
- Gajanan S Patil
Council of Scientific and Industrial Research, Ministry of Science and Technology, India
- Sudipta Mondal
- Rajan Sankaranarayanan
University Grants Commission
- Sakshi Shambhavi
Science and Engineering Research Board, India (J. C. Bose Fellowship)
- Rajan Sankaranarayanan
The funders had no role in study design, data collection and interpretation, or the decision to submit the work for publication.
Acknowledgements
We acknowledge Dr. Pananghat Gayathri (IISER-Pune) and Dr. Raghunand R Tirumalai (CSIR-CCMB) for sharing the genomic DNA samples of Myxococcus xanthus and Mycobacterium smegmatis, respectively.
Copyright
© 2021, Patil et al.
This article is distributed under the terms of the Creative Commons Attribution License, which permits unrestricted use and redistribution provided that the original author and source are credited.
Metrics
-
- 2,964
- views
-
- 358
- downloads
-
- 17
- citations
Views, downloads and citations are aggregated across all versions of this paper published by eLife.
Citations by DOI
-
- 17
- citations for umbrella DOI https://doi.org/10.7554/eLife.70067