In situ imaging of bacterial outer membrane projections and associated protein complexes using electron cryo-tomography
Abstract
The ability to produce outer membrane projections in the form of tubular membrane extensions (MEs) and membrane vesicles (MVs) is a widespread phenomenon among diderm bacteria. Despite this, our knowledge of the ultrastructure of these extensions and their associated protein complexes remains limited. Here, we surveyed the ultrastructure and formation of MEs and MVs, and their associated protein complexes, in tens of thousands of electron cryo-tomograms of ~90 bacterial species that we have collected for various projects over the past 15 years (Jensen lab database), in addition to data generated in the Briegel lab. We identified outer MEs and MVs in 13 diderm bacterial species and classified several major ultrastructures: (1) tubes with a uniform diameter (with or without an internal scaffold), (2) tubes with irregular diameter, (3) tubes with a vesicular dilation at their tip, (4) pearling tubes, (5) connected chains of vesicles (with or without neck-like connectors), (6) budding vesicles and nanopods. We also identified several protein complexes associated with these MEs and MVs which were distributed either randomly or exclusively at the tip. These complexes include a secretin-like structure and a novel crown-shaped structure observed primarily in vesicles from lysed cells. In total, this work helps to characterize the diversity of bacterial membrane projections and lays the groundwork for future research in this field.
Introduction
Membrane extensions and vesicles (henceforth referred to as MEs and MVs) have been described in many types of bacteria. They are best characterized in diderms, where they stem mainly from the outer membrane (OM; we thus refer to OMEs and OMVs) and perform a variety of functions (Schwechheimer and Kuehn, 2015; Jan, 2017; D’Souza et al., 2018; Toyofuku et al., 2019). For example, the OMEs of Shewanella oneidensis (aka nanowires) are involved in extracellular electron transfer (Pirbadian et al., 2014; Subramanian et al., 2018). The OM tubes of Myxococcus xanthus are involved in the intra-species transfer of periplasmic and OM-associated material between different cells that is essential for the complex social behavior of this species (Ducret et al., 2013; Wei et al., 2014; Remis et al., 2014). The OMVs of Vibrio cholerae act as a defense mechanism, helping the bacterium circumvent phage infection (Reyes-Robles et al., 2018). A marine Flavobacterium affiliated with the genus Formosa (strain Hel3_A1_48) extrudes membrane tubes and vesicles that contain the type IX secretion system and digestive enzymes (Fischer et al., 2019). OMVs often function in pathogenesis. The OM blebs and vesicles of Flavobacterium psychrophilum have proteolytic activities that help release nutrients from the environment and impede the host immune system (Møller et al., 2005). The OMVs of Francisella novicida contain virulence factors, suggesting they are involved in pathogenesis (McCaig et al., 2013). Similarly, the virulence of Flavobacterium columnare is associated with the secretion of OMVs (Laanto et al., 2014), and membrane tubes and secreted vesicles have been observed in other, human pathogens like Helicobacter pylori and Vibrio vulnificus (Chang et al., 2018; Hampton et al., 2017).
MEs and MVs are also produced by monoderm bacteria and archaea. MVs stemming from the cytoplasmic membrane of Gram-positive bacteria have been reported to encapsulate DNA (see Brown et al., 2015 and references therein). Membrane nanotubes were recently discovered in the Gram-positive Bacillus subtilis, as well as the Gram-negative Escherichia coli. These nanotubes were found to connect two different bacterial cells and are involved in the transfer of cytoplasmic material between bacterial cells of the same and different species, and even to eukaryotic cells (Bhattacharya et al., 2019; Dubey and Ben-Yehuda, 2011; Baidya et al., 2018; Pande et al., 2015; Benomar et al., 2015; Baidya et al., 2020; Pal et al., 2019). In addition, a recent study suggested that nanotubes assist the growth of Pseudomonas aeruginosa on periodic nano-pillar surfaces (Cao et al., 2020).
The structures of MEs and MVs are as varied as their functions. While S. oneidensis nanowires are chains of interconnected OMVs with variable diameter and decorated with cytochromes (Subramanian et al., 2018), OM tubes of H. pylori have a fixed diameter of ~40 nm and are characterized by an inner scaffold and lateral ports (Chang et al., 2018). V. vulnificus produces tubes from which vesicles ultimately pinch off by biopearling, forming a regular concentric pattern surrounding the cell (Hampton et al., 2017). Cells with an external surface layer (S-layer) can produce structures known as ‘nanopods’, which consist of MVs inside a sheath of S-layer. These have been reported in the soil-residing bacterium Delftia sp. Cs1–4 (Shetty et al., 2011) and archaea of the order Thermococcales (Marguet et al., 2013). Finally, some diderms produce DNA-containing MVs consisting of both IM and OM (see Toyofuku et al., 2019 and references therein).
Different models have been proposed for how MEs and MVs form. In diderms, membrane blebbing may occur due to changes in the periplasmic turgor pressure, lipopolysaccharide repulsion, or alterations in the contacts between the OM and the peptidoglycan cell wall (Toyofuku et al., 2019). Chains of interconnected vesicles are often observed, either as a result of direct vesicular budding from the OM or due to biopearling of membrane tubes (Subramanian et al., 2018; Fischer et al., 2019). Formation of tubes is thought to be a stabilizing factor as it results in smaller vesicles, with tubes pearling into distal chains of vesicles that eventually disconnect (Bar-Ziv and Moses, 1994). Other extensions may be formed by dedicated machinery. Interestingly, nanotubes involved in cytoplasmic exchange have been reported to be dependent on a conserved set of proteins involved in assembly of the flagellar motor known as the type III secretion system core complex (CORE): FliP/O/Q/R and FlhA/B (Bhattacharya et al., 2019; Pal et al., 2019). Recently, it was also shown that the formation of bacterial nanotubes significantly increases under stress conditions or in dying cells, caused by biophysical forces resulting from the action of the cell wall hydrolases LytE and LytF (Pospíšil et al., 2020).
Structural studies of MEs and MVs have relied mainly on scanning electron microscopy (SEM), conventional transmission electron microscopy (TEM), and light (fluorescence) microscopy. While these methods have significantly advanced our understanding, they are limited in terms of the information they can provide. For instance, in SEM and conventional TEM, sample preparation such as fixation, dehydration, and staining disrupt membrane ultrastructure. While light microscopy can reveal important information about the dynamics and timescales on which MEs and MVs form (e.g. Bos et al., 2021), no ultrastructural details can be resolved; MEs and MVs of different morphology appear identical. Currently, only electron cryo-tomography (cryo-ET) allows visualization of structures in a near-native state inside intact (frozen-hydrated) cells with macromolecular (~5 nm) resolution. However, this capability is limited to thin samples (few hundred nanometers thick, like individual bacterial cells of many species) while thicker samples like the central part of eukaryotic cells, thick bacterial cells, or clusters of bacterial cells are not amenable for direct cryo-ET imaging. Such thick samples can be rendered suitable for cryo-ET experiments by thinning them first using different methods including focused ion beam milling and cryosectioning (Kaplan et al., 2021a). Cryo-ET has already been invaluable in revealing the structures of several MEs, including S. oneidensis nanowires (Subramanian et al., 2018), H. pylori tubes (Chang et al., 2018), Delftia acidovorans nanopods (Shetty et al., 2011), V. vulnificus OMV chains (Hampton et al., 2017), and more recently cell-cell bridges in the archaeon Haloferax volcanii (Sivabalasarma et al., 2020).
To understand what MEs exist in bacterial cells and how they might form, we undertook a survey of ~90 bacterial species, drawing on a database of tens of thousands of electron cryo-tomograms of intact cells collected by our group for various projects over the past 15 years (Ding et al., 2015; Ortega et al., 2019), in addition to data generated in the Briegel lab. Our survey revealed OM projections in 13 diderm bacterial species. These projections took various forms: (1) tubes with a uniform diameter and with an internal scaffold, (2) tubes with a uniform diameter and without a clear internal scaffold, (3) tubes with a vesicular dilation at their tip (teardrop-like extensions), (4) tubes with irregular diameter or pearling tubes, (5) interconnected chains of vesicles with uniform neck-like connectors, (6) budding or detached OMVs, and (7) nanopods. We also identified protein complexes associated with MEs and MVs in these species. These complexes were either seemingly randomly distributed on the MEs and MVs or exhibited a preferred localization at their tip.
Results
We examined tens of thousands of electron cryo-tomograms of ~90 bacterial species collected in the Jensen lab for various projects over the past 15 years together with tomograms collected in the Briegel lab. Most cells were intact, but some had naturally lysed. Note that we make this classification based on the cells’ appearance in tomograms; intact cells have an unbroken cell envelope, uniform periplasmic width, and consistently dense cytoplasm. In addition to cryo-tomograms of cells, this dataset also included naturally shed vesicles purified from S. oneidensis. In all, we identified OMEs and OMVs in 13 bacterial species (summarized in Table 1, Table 2).
A summary of the species included in this study and the major membrane structures identified in each species.
Note that the approximation symbol before the number of cells indicates that in many tomograms we only see a part of the cell(s).
Species | Class | No. of cells | Features observed | |||||||
---|---|---|---|---|---|---|---|---|---|---|
Tubes | Vesicle chains | Budding/ vesicles | Nanopods | |||||||
Uniform diameter – scaffold | Uniform diameter – no scaffold | Variable diameter | Pearling | Connectors | No connectors | |||||
Shewanella oneidensis | Gammaproteobacteria | ~700 | See Subramanian et al., 2018 | > 100 | ||||||
Pseudoalteromonas luteoviolacea | Gammaproteobacteria | ~67 | ~100 | ~10 | ||||||
Hylemonella gracilis | Betaproteobacteria | ~105 | 3 | 4 | 15 | |||||
Delftia acidovorans | Betaproteobacteria | n.a. | See Shetty et al., 2011 | |||||||
Magnetospirillum magneticum | Alphaproteobacteria | ~56 | 49 | |||||||
Caulobacter crescentus | Alphaproteobacteria | ~464 | 53 | |||||||
Helicobacter hepaticus | Epsilonproteobacteria | ~28 | 2 | |||||||
Helicobacter pylori | Epsilonproteobacteria | ~883 | >100 | >100 | ||||||
Myxococcus xanthus | Deltaproteobacteria | ~2000 | >100 | >100 | >100 | |||||
Borrelia burgdorferi | Spirochaetes | ~61 | 9 | 19 | 16 | |||||
Flavobacterium johnsoniae | Flavobacteria | ~203 | ~45 | ~15 | >100 | |||||
Flavobacterium anhuiense | Flavobacteria | ~49 | 5 | 7 | 4 | >100 (including the teardrop-like extensions) | ||||
Chitinophaga pinensis | Chitinophagia | ~61 | 11 | 12 | 3 | 81 |
The different bacterial strains used in this study.
Species | Strain | Relevant references |
---|---|---|
Shewanella oneidensis | MR-1 211,586 | Subramanian et al., 2018; Kaplan et al., 2019a; Kaplan et al., 2019b |
Pseudoaltermonas luteoviolacea | 43,657 | Shikuma et al., 2014 |
Hylemonella gracilis | ATCC 19624 887,062 | Kaplan et al., 2020; Chen et al., 2011; Kaplan et al., 2021b |
Delftia acidovorans | Cs1-4 80,866 | Shetty et al., 2011 |
Magnetospirillum magneticum | AMB-1 342,108 | Cornejo et al., 2016 |
Caulobacter crescentus | NA1000 | Kaplan et al., 2021c |
Helicobacter hepaticus | ATCC 51449 235,279 | Chen et al., 2011 |
Helicobacter pylori | 26,695 | Chang et al., 2018 |
Myxococcus xanthus | DK1622 | Chang et al., 2016 |
Borrelia burgdorferi | B31 224,326 | Briegel et al., 2009; Chen et al., 2011 |
Flavobacterium johnsoniae | CJ2618 | This study |
Flavobacterium anhuiense | 98 | Carrión et al., 2019 |
Chitinophaga pinensis | 94 | Carrión et al., 2019 |
I – The diverse forms of bacterial membrane structures
Based on their features, we classified membrane projections into the following categories: (1) tubular extensions with a uniform diameter and with an internal scaffold (Figure 1a and b and Figure 1—figure supplements 1 and 2); (2) tubular extensions with a uniform diameter and without a clear internal scaffold (Figure 1c–g and Figure 1—figure supplement 3); (3) tubular extensions with a vesicular dilation at the tip (a teardrop-like structure) and irregular dark densities inside (Figure 1h); (4) tubular extensions with irregular diameter or pearling tubes (Figure 2a–g); (5) interconnected chains of vesicles with uniform neck-like connectors (Figure 2h & i); (6) budding or detached vesicles: budding vesicles were still attached to the membrane, while detached vesicles were observed near a cell and could have budded directly or from a tube that pearled (Figure 3a–d and Figure 3—figure supplement 1); (7) nanopods: tubes of S-layer containing OMVs (Figure 3e–i). See Table 1 for a summary of these observations.
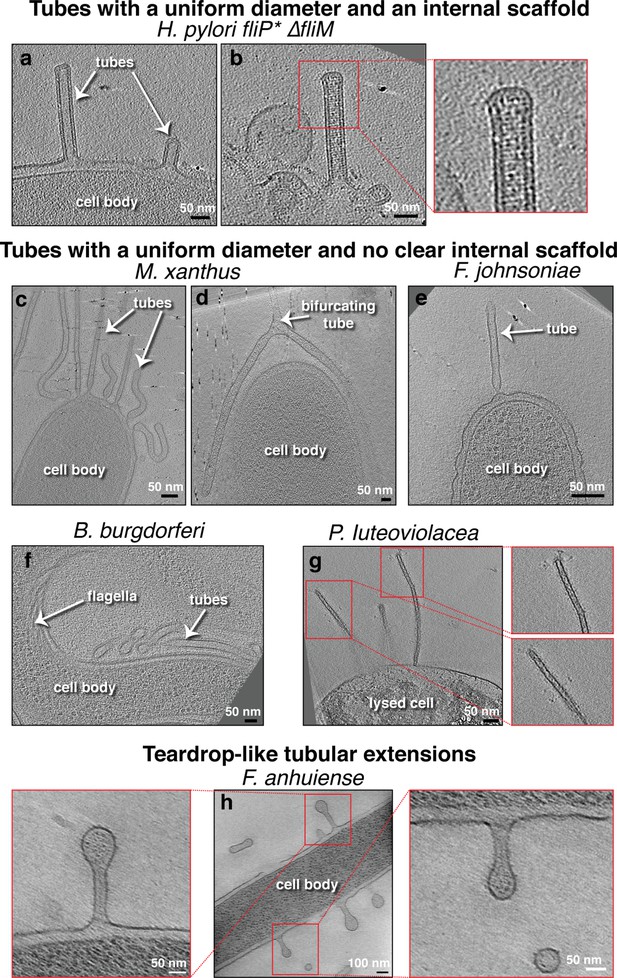
Membrane tubes with a uniform diameter, either with or without an internal scaffold.
Slices through electron cryo-tomograms of the indicated bacterial species highlighting the presence of outer membrane extensions (OMEs) with uniform diameters and either with (a–b) or without (c–g) an internal scaffold, and teardrop-like extensions (h). In this and all subsequent figures, red boxes indicate enlarged views of the same slice. Scale bars are 50 nm, except in main panel (h) 100 nm.
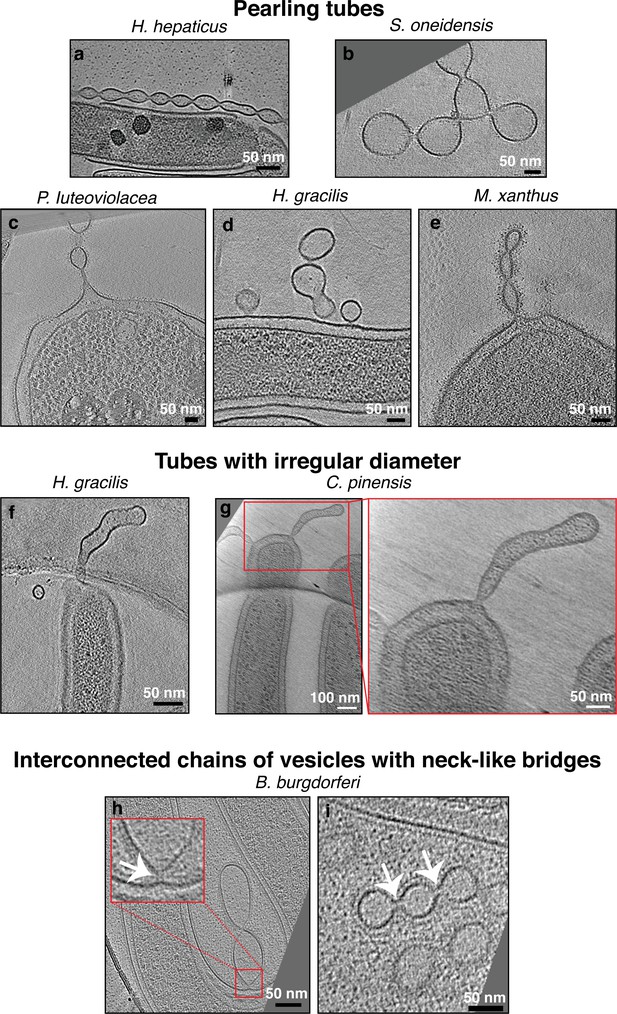
Pearling tubes, tubes with irregular diameter, and vesicle chains with neck-like connections.
Slices through electron cryo-tomograms of the indicated bacterial species highlighting the presence of pearling tubes (a–e), tubes with irregular diameter (f–g), or outer membrane vesicle (OMV) chains connected by neck-like bridges (h–i). White arrows in the enlargement in (h) and in panel (i) point to the 14 nm connectors in Borrelia burgdorferi. Scale bars are 50 nm, except in main panel (g) 100 nm.
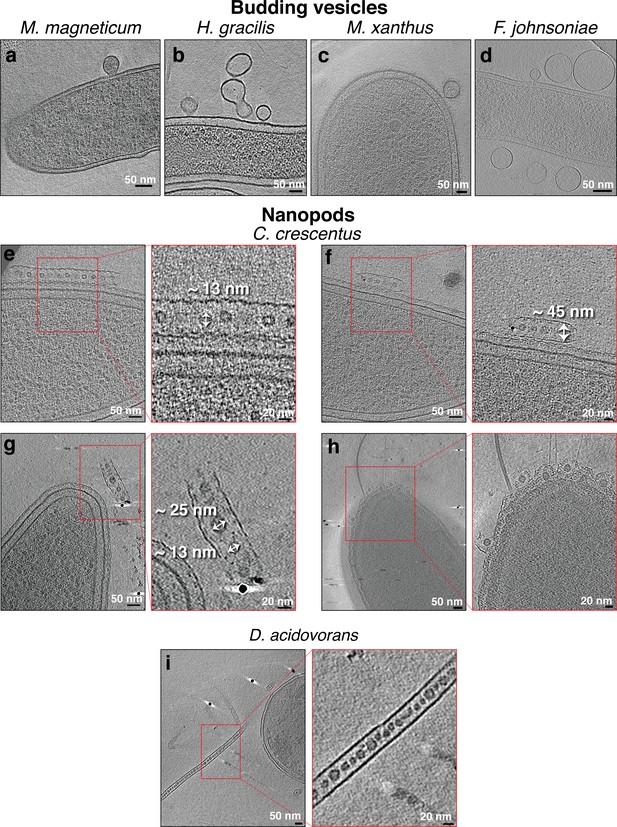
Budding outer membrane vesicles (OMVs) and nanopods.
Slices through electron cryo-tomograms of the indicated bacterial species highlighting the presence of budding vesicles (a–d) or nanopods (e– i). Scale bars are 50 nm in main panels and 20 nm in enlargements.
Scaffolded membrane tubes were observed only in H. pylori and had a uniform diameter of 40 nm. The H. pylori strain imaged (fliP*) contains a naturally occurring point mutation that disrupts the function of FliP, the platform upon which other CORE proteins assemble (Fukumura et al., 2017; Fabiani et al., 2017; Minamino et al., 2019). In addition, the dataset contained other mutants in this fliP* background including additional CORE proteins (ΔfliO and ΔfliQ), flagellar basal body proteins (ΔfliM and ΔfliG), and the tyrosine kinase required for expression of the class II flagellar genes (ΔflgS) (Lertsethtakarn et al., 2011; Figures 1a–b–4, Figure 1—figure supplement 1 and Table 3). This suggests that the H. pylori membrane tubes are unrelated to the CORE-dependent nanotubes that mediate cytoplasmic exchange in B. subtilis and other species (Bhattacharya et al., 2019; Pal et al., 2019).
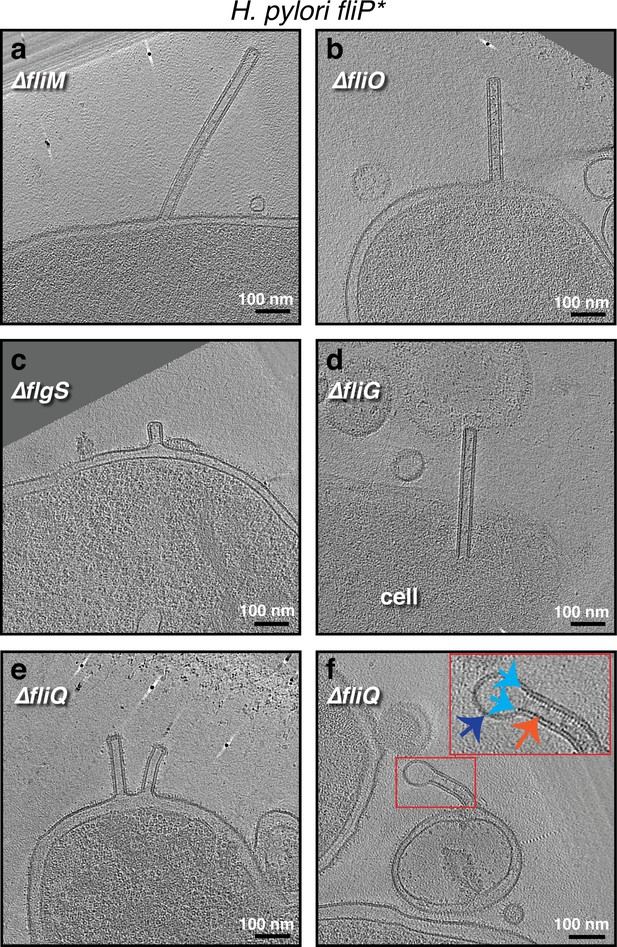
The formation of outer membrane (OM) tubes persists in various Helicobacter pylori mutants, including CORE mutants.
Slices through electron cryo-tomograms of the indicated H. pylori mutants (all in the fliP* background) showing the presence of membrane tubes. The enlargement in (f) highlights a dilation at the end of the tube (dark blue arrow) due to the absence of the scaffold (orange arrow). Light blue arrows indicate the end points of the scaffold. Scale bar is 100 nm.
Numbers of tubes identified in different Helicobacter pylori mutants.
Note that the approximation symbol before the number of cells indicates that in many tomograms we only see a part of the cell(s).
Mutant | Number of cells | Number of tubes |
---|---|---|
H. pylori ∆fliG fliP* | ~47 | 12 |
H. pylori ∆fliM fliP* | ~265 | 88 |
H. pylori ∆fliO fliP* | ~267 | 49 |
H. pylori ∆fliQ fliP* | ~220 | 55 |
H. pylori ∆flgS fliP* | ~84 | 15 |
Previously, H. pylori tubes were described as forming in the presence of eukaryotic host cells (Chang et al., 2018). Here, however, we observed tubes in H. pylori grown on agar plates in the absence of eukaryotic cells, suggesting that they also form in the absence of host cells. We observed some differences, though, from the tubes formed in the presence of host cells: the tube ends were closed, no clear lateral ports were seen, and the tubes were usually straight. While some of these tubes extended more than 0.5 μm, we never observed pearling. However, in some tubes, the internal scaffold did not extend all the way to the tip, and its absence caused the tube to dilate (from 40 nm in the presence of the scaffold to 66 nm in its absence, see Figure 4f and examples in Figure 1—figure supplement 1b and d). In some cases we also observed tubes stemming from vesicles resulting from cell lysis (Figure 4f, Figure 1—figure supplement 1b and d, Figure 1—figure supplement 2), and dark densities could be seen at the base of many of these tubes associated with vesicles (Figure 1—figure supplement 1d).
In Flavobacterium anhuiense and Chitinophaga pinensis, which are both endophytic species extracted from sugar beet roots, in addition to tubes with irregular diameter and OMVs (Figure 2g), tubular extensions with a uniform diameter, and a vesicular dilation (teardrop-like structure) were observed stemming from the sides of the cell in F. anhuiense (Figure 1h). Interestingly, irregular dark densities were observed inside these teardrop-like extensions (Figure 1h). Chains of vesicles connected by neck-like bridges were similarly observed in a single species: Borrelia burgdorferi. The bridges were consistently ~14 nm in length and ~8 nm in width. Where chains were seen attached to the OM, a neck-like connection was present at the budding site (Figure 2h). Vesicles in each chain were of a uniform size, usually 35–40 nm wide (e.g. Figure 2i), but occasionally larger (e.g. Figure 2h).
When both tubes and vesicles were observed in the same species, the tubes generally had a more uniform diameter than the vesicles, which were of variable sizes and often had larger diameters than the tubes (Figure 3—figure supplement 1 and Figure 3—figure supplement 2). In addition, when a tube pearled into vesicles, there was no clear correlation between the length of the tube and the initiation point of pearling, with some tubes extending for many micrometers without pearling while other, shorter tubes were in the process of forming vesicles (Video 1, Video 2, Video 3, and Figure 2). As usually only one (or part of a) cell is present in the cryo-tomogram, we cannot exclude that differences in the extracellular environments, like the presence of a cluster of cells in the vicinity of the individual cells with pearling tubes, might play a role in this observation. Pearling tubes differ from tubes with irregular diameter by the presence of a deep constriction in some part of the tube, while chains of vesicles are entirely made up of semi-circular vesicles connected by thin constrictions suggesting different mechanisms are responsible for the formation of these different extensions. While most pearling was seen at the tips of tubes, pearling occasionally occurred simultaneously at both proximal and distal ends of the same tube (Video 3). With one exception, pearling was seen in all species with tubes of uniform diameter and no internal scaffold. The exception was lysed Pseudoalteromonas luteoviolacea, which had narrow tubes only 20 nm in diameter (Figure 1g). Some lysed P. luteoviolacea contained wider, pearling tubes (Figure 2c). Interestingly, the tubes of various M. xanthus strains (see Materials and methods) and P. luteoviolacea could bifurcate into branches, each of which had a uniform diameter similar to that of the main branch (Video 4 and Figure 1d and Figure 1—figure supplement 3).
An electron cryo-tomogram of an Myxococcus xanthus cell with multiple outer membrane tubes stemming from the cell.
An electron cryo-tomogram of an Flavobacterium johnsoniae cell with outer membrane tubes stemming from the cell.
Note the wavy outer membrane of the cell.
An electron cryo-tomogram of an Myxococcus xanthus cell with a pearling outer membrane tube stemming from the cell.
An electron cryo-tomogram of an Myxococcus xanthus cell with multiple branched outer membrane tubes stemming from the cell.
In Caulobacter crescentus tomograms, we identified structures very similar to the ‘nanopod’ extensions previously reported in D. acidovorans (Shetty et al., 2011). These structures consist of a tube made of the S-layer encasing equally spaced OMVs (Figure 3e–h and Video 5). The diameter of the S-layer tubes was ~45 nm and vesicles exhibited diameters ranging from ~13 to 25 nm. The nanopods were seen either detached from the cell (Figure 3e–g) or budding from the pole of C. crescentus (Figure 3h).
An electron cryo-tomogram of a Caulobacter crescentus cell with a nanopod (black arrow) close to the cell.
II – Protein complexes associated with membrane structures
Next, we examined protein complexes associated with OMEs and OMVs that we could identify in our cryo-tomograms. These complexes fell into three categories: (1) seemingly randomly located complexes found on OMEs, OMVs, and cells; (2) seemingly randomly located complexes observed only on OMEs and OMVs; and (3) complexes exclusively located at the tip of OMEs/OMVs.
In the first category, we observed what appeared to be the OM-associated portion of the empty basal body of the type IVa pilus (T4aP) machinery in OMEs of M. xanthus. These complexes, which were also found in the OM of intact cells, did not exhibit a preferred localization or regular arrangement within the tube at least within the fields of view provided by our cryo-tomograms (Figure 5).
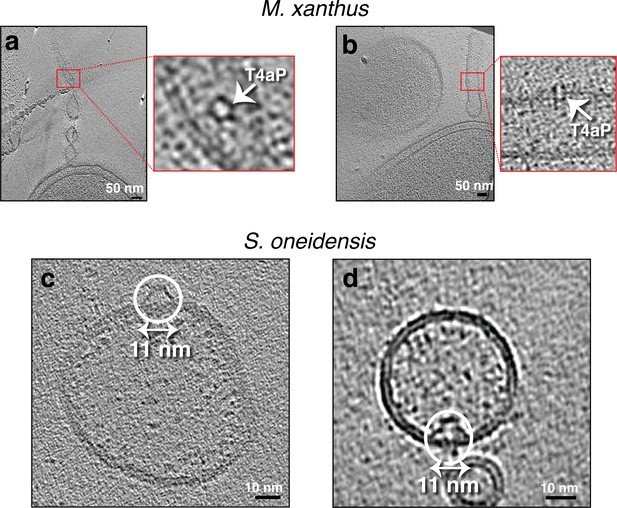
Seemingly randomly located protein complexes on outer membrane extensions (OMEs) of Myxococcus xanthus and purified membrane vesicles (MVs) of Shewanella oneidensis.
(a and b) Slices through electron cryo-tomograms of M. xanthus indicating the presence of pearling tubes with top (a) and side (b) views of type IVa pilus basal bodies (T4aP). Scale bar is 50 nm. (c and d) Slices through electron cryo-tomograms of purified S. oneidensis naturally shed MEs and MVs highlighting the presence of trapezoidal structures on the outside (c) and inside (d) of vesicles. Scale bar is 10 nm.
The second category of protein complexes, observed only on MEs and not on cells, contained two structures. The first was a trapezoidal structure observed on purified OMVs of S. oneidensis. The structure was ~11 nm wide at its base at the membrane and was seen sometimes on the outside (Figure 5c) and sometimes the inside of vesicles (Figure 5d). The second structure was a large crown-like complex. We first observed these complexes on the outer surface of MVs associated with lysed M. xanthus cells (Figure 6a). Occasionally, they were also present on what appeared to be the inner leaflet of the inner membrane of lysed cells (Figure 6b). The exact topology is difficult to determine, however, since the arrangement of IM and OM can be confounded by cell lysis. The structure of this complex was consistent enough to produce a subtomogram average from nine examples, improving the signal-to-noise ratio and revealing greater detail (Figure 6c). These crown-like complexes are ~40 nm tall with a concave top and a base ~35 nm wide at the membrane (Figure 6c). No such complexes were seen on OMEs and OMVs associated with intact M. xanthus cells. We identified a morphologically similar crown-like complex on the outside of some tubes and vesicles purified from S. oneidensis (Figure 6d–f). However, this complex was smaller, ~15 nm tall and ~20 nm wide at its base. As these MEs/MVs from S. oneidensis were purified, we cannot know whether they stemmed from lysed or intact cells. Interestingly, we found a similar large crown-like structure associated with lysed cells of two other species in which we did not identify MEs, namely Pseudomonas flexibilis and P. aeruginosa (Figure 6g–j and Figure 6—figure supplement 1).
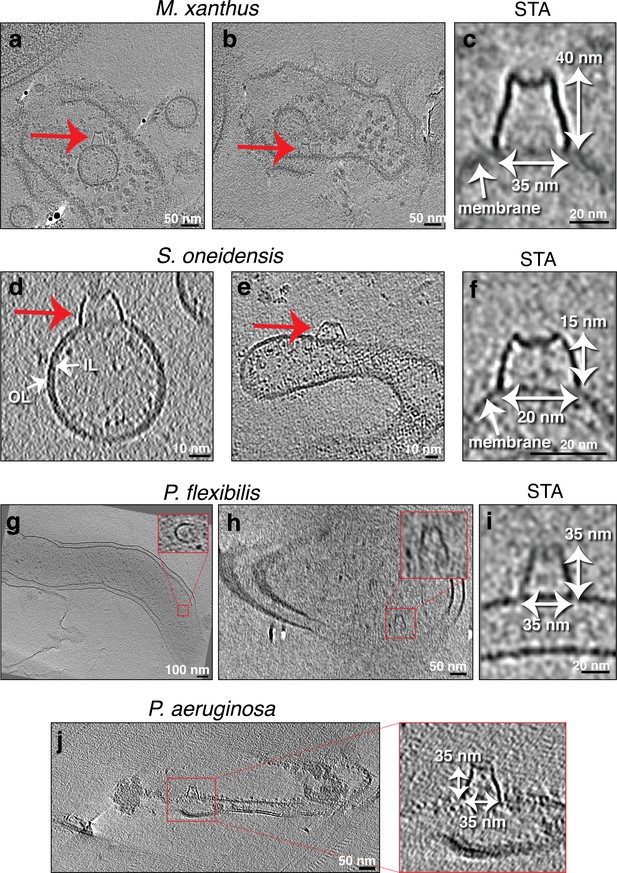
Seemingly randomly located protein complexes associated with lysed cells.
Slices through electron cryo-tomograms of lysed cells (a, b, g, h, and j) or purified membrane extensions (MEs) and membrane vesicles (MVs) (d and e) showing the presence of MVs and lysed membranes with a crown-like complex (red arrows and red boxed enlargements). Scale bars: 50 nm (a, b, h, and j), 100 nm (g), 10 nm (d and e). (c, f, and i) Central slices through subtomogram averages (with twofold symmetry along the Y-axis applied) of nine particles (c), four particles, (f), or three particles (i) of the crown-like complex in the indicated species. Scale bar is 20 nm. OL = outer leaflet, IL = inner leaflet.
In the third category, we observed a secretin-like complex in many tubes and vesicles of F. johnsoniae. Secretins are proteins that form a pore in the OM and are associated with many secretion systems like type IV pili and type II secretion systems (T2SS) (Chang et al., 2016; Ghosal et al., 2019; Gold et al., 2015). In tubes attached to the cell, the complex was always located at the distal tip (Figure 7, Figure 7—figure supplement 1, and Video 6). From 35 membrane tubes seen attached to cells, we identified a secretin-like complex at the tip of 25 of them (~70%). In OMEs disconnected from the cell, the secretin-like complex was always located at one end (Figure 7b & e). In total, we identified 88 secretin-like particles in 198 tomograms, none of which were located in the middle of a tube. As the MEs are less crowded than cellular periplasm and usually thinner than intact cells, we could clearly distinguish an extracellular density and three periplasmic densities in side views (red and purple arrows, respectively, in Figure 7a). Top views showed a plug in the center of the upper part of the complex (yellow arrows in Figure 7g & h). Subtomogram averaging revealed details of the complex, including the plug and a distinct lower periplasmic ring (Figure 7i & j & Figure 7—figure supplement 2). While the upper two periplasmic rings were clearly distinguishable in many of the individual particles (e.g. Figure 7a), they did not resolve as individual densities in the subtomogram average (Figure 7i). The extracellular density was not resolved at all in the average, suggesting flexibility in this part.
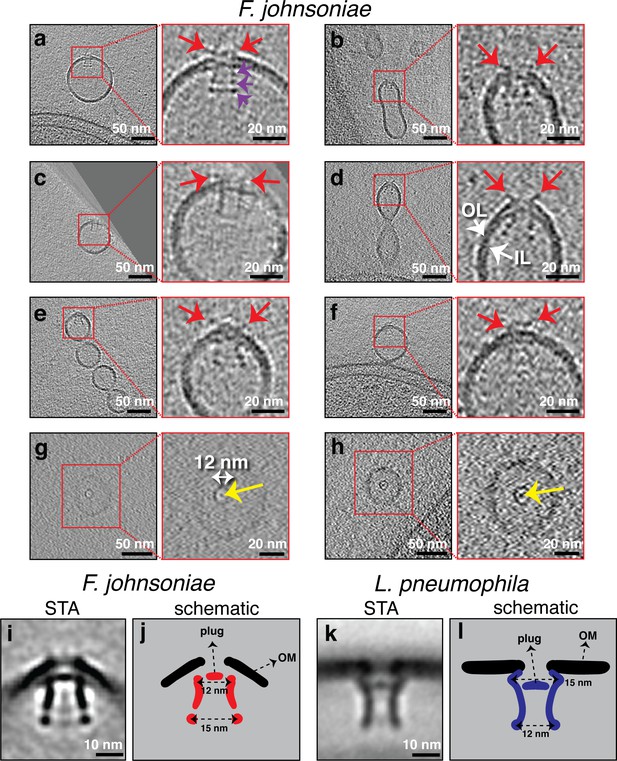
Secretin-like complexes located at the tip of outer membrane extensions (OMEs) and outer membrane vesicles (OMVs) in Flavobacterium johnsoniae.
Slices through electron cryo-tomograms of F. johnsoniae illustrating the presence of secretin-like complexes (side views in a–f), top views in (g and h) with yellow arrows pointing to the plug in OMEs and OMVs of F. johnsoniae. Red arrows point to the extracellular part of the complex. Purple arrows in the enlargement in (a) point to the three periplasmic densities. Scale bars are 50 nm in main panels and 20 nm in enlargements. (i) A central slice through the subtomogram average of 88 particles of the secretin-like complex (with twofold symmetry along the Y-axis applied). Scale bar is 10 nm. (j) A schematic representation of the STA shown in (i). (k) A central slice through the subtomogram average of the secretin of the type II secretion systems (T2SS) of Legionella pneumophila (EMD 20713, see Ghosal et al., 2019). Scale bar is 10 nm. (l) A schematic representation of the STA shown in (k).
An electron cryo-tomogram of an Flavobacterium johnsoniae cell highlighting the presence of secretin-like particles at the tips of outer membrane tubes.
Previous studies showed that a species which belongs to the same phylum as F. johnsoniae, namely Cytophaga hutchinsonii, uses a putative T2SS to degrade cellulose (Wang et al., 2017). Since F. johnsoniae also degrades polysaccharides and other polymers, we BLASTed the sequence of the well-characterized V. cholerae T2SS secretin protein, GspD (UniProt ID P45779), against the genome of F. johnsoniae and found a hit, GspD-like T2SS secretin protein (A5FMB4), with an e-value of 1e–9. This result and the general morphological similarity of this secretin to the published structure of the T2SS (Ghosal et al., 2019) suggested that the complex we observed might be the secretin of a T2SS. We therefore compared our subtomogram average with the only available in situ structure of a T2SS, a recent subtomogram average of the Legionella pneumophila T2SS (Ghosal et al., 2019; Figure 7i–l). The two structures were generally similar in length and both had a plug in the upper part of the complex. However, we also observed differences between the two structures. In L. pneumophila, the widest part of the secretin (15 nm) is located near the plug close to the OM, and the lower end of the complex is narrower (12 nm). In F. johnsoniae, this topology is reversed, with the narrowest part near the plug and OM (Figure 7i–l). Additionally, the lowest domain of the L. pneumophila secretin did not resolve into a distinct ring as we saw in F. johnsoniae and no extracellular density was observed in L. pneumophila, either in the subtomogram average or single particles (Ghosal et al., 2019).
Discussion
Our results highlight the diversity of MEs’ and MVs’ structures that bacteria can form even within a single species (Figure 8). For example, we saw two types of membrane tubes in lysed P. luteoviolacea cells: one narrower with a uniform diameter of 20 nm which did not pearl into vesicles, and one wider with a variable diameter that did pearl into vesicles (Figures 1g and 2c), a distinction which suggests that these extensions play different roles. Similarly, interspecies differences likely reflect different functions. For instance, the tubes of M. xanthus were on average longer, more abundant, and more branched than the MEs of other species (Videos 1 and 4), which is likely related to their role in communication between cells of this highly social species. However, one interesting observation in all the species we investigated here is that there was no clear distinctive molecular machine at the base of the membrane projections, raising the question of what drives their formation. This observation is consistent with a recent study which showed that liquid-like assemblies of proteins in membranes can lead to the formation of tubular extensions without the need for solid scaffolds (Yuan et al., 2021). In addition, differences in the lipid compositions among the various species investigated here might also play a role in the formation of these different forms of projections.
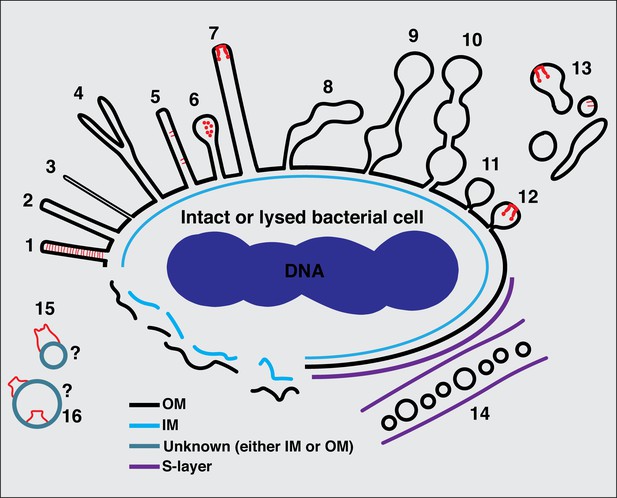
Summary of types of membrane extensions (MEs) and membrane vesicles (MVs) identified in this study.
(1) Tubes with a uniform diameter and with an internal scaffold; (2 and 3) tubes with a uniform diameter but without an internal scaffold; (4) bifurcating tubes; (5) tubes with seemingly randomly located protein complexes (type IVa pilus [T4aP]); (6) teardrop-like extensions; (7) tubes with a secretin-like complex at their tip; (8) tubes with irregular diameter; (9) pearling tubes; (10) interconnected chains of vesicles with 14 nm connectors; (11) budding vesicles; (12) budding vesicles with a secretin-like complex at their tip; (13) various disconnected membrane structures in the vicinity of bacterial cells; (14) nanopods in species with an inner membrane (IM), outer membrane (OM), and S-layer; (15) membrane structures with a crown-like complex from lysed cells; (16) purified outer MVs (OMVs) with trapezoidal complexes. The question marks in (15) and (16) indicate the difficulty of determining whether a membrane structure from lysed cells or purified vesicles originated from the IM or the OM or is in its original topology.
The scaffolded uniform tubes of H. pylori that we observed were formed in samples not incubated with eukaryotic cells, indicating that they can also form in their absence. However, the tubes we found had closed ends and no clear lateral ports, while some of the previously reported tubes (formed in the presence of eukaryotic host cells) had open ends and prominent ports (Chang et al., 2018). It is possible that such features are formed only when H. pylori are in the vicinity of host cells. Moreover, while it was previously hypothesized that the formation of membrane tubes in H. pylori (when they are in the vicinity of eukaryotic cells) is dependent on the cag T4SS (Chang et al., 2018), we could not identify any clear correlation between the emanation of membrane tubes and cag T4SS particles in our samples where H. pylori was not incubated with host cells. We also show that the tubes of H. pylori are CORE-independent, indicating that they are different from the CORE-dependent nanotubes described in other species.
A recent study showed that the formation of bacterial tubes significantly increases when cells are stressed or dying (Pospíšil et al., 2020). Consistent with this, in our cryo-tomograms we saw many MEs and MVs associated with lysed cells (such as in H. pylori, Helicobacter hepaticus, and P. luteoviolacea). We also saw tubes and vesicles stemming from intact cells. Given the nature of cryo-ET snapshots, we cannot tell whether a cell that appears intact is stressed, nor can we know whether MEs/MVs formed before or after a cell lysed. One observation which might be related to this issue comes from F. johnsoniae where tubes with regular diameters were seen stemming mainly from cells with a noticeably wavy OM (45 examples), while pearling tubes and OMVs stemmed primarily from cells with a smooth OM (>100 examples). Compare, for example, the cells in Figure 1e and Figure 7—figure supplement 1 and Videos 2 and 6 (wavy OM) to those in Figures 3d and 7a and f (smooth OM).
In C. crescentus, we observed for the first time ‘nanopods’, a structure previously reported in D. acidovorans (Shetty et al., 2011). Both of these species are diderms with an S-layer, suggesting that nanopods may be a general form for OMVs in bacteria with this type of cell envelope. Nanopods were proposed to help disperse OMVs in the partially hydrated environment of the soil where D. acidovorans lives; it will be interesting to study their function in aquatic C. crescentus.
Examining protein complexes associated with OMEs and OMVs, some seemed to reflect a continuation of the same complexes found on the membrane from which the extensions stemmed, such as the T4aP basal body in M. xanthus (Chang et al., 2016). Others, however, were only observed on MEs and not on cells. This could be because the complexes are related to the formation of the MEs, or it might simply reflect the fact that these extensions are generally thinner and less crowded than the bacterial periplasm, making the complexes easier to see in cryo-tomograms. Interestingly, the crown-like complex we observed in M. xanthus, P. aeruginosa, and P. flexibilis was exclusively associated with the membranes of lysed cells; we never observed it on OMEs and OMVs stemming from intact cells in M. xanthus. We observed a morphologically similar crown-like structure with different dimensions in purified naturally shed MEs/MVs of S. oneidensis, where we cannot know whether they arose from intact or lysed cells. The crown-like structures are remarkably large and their function remains a mystery. Due to the disruption of membranes in lysed cells, the topology of these complexes is difficult to unravel. However, these structures share a morphological similarity to a membrane-associated dome protein complex recently described on the limiting membrane of the lamellar bodies inside alveolar cells (Klein et al., 2021).
Similarly, regarding the different, trapezoidal structure in S. oneidensis, the fact that it was seen on both the outside and inside of purified MVs suggests that some of the purified vesicles adopted an inside-out orientation during purification (a documented phenomenon; Kaplan et al., 2016). Interestingly, the overall architecture and dimensions of this trapezoidal structure are reminiscent of those of a recently solved structure of the E. coli polysaccharide co-polymerase WzzB (Wiseman et al., 2021). We hope future investigation by methods like mass spectrometry will characterize these novel ME/MV-associated protein complexes.
In F. johnsoniae, we observed secretin-like particles at the tip of ~70% of tubes stemming from the OM. This strong spatial correlation suggests a role for the secretin-like complex in the formation of MEs in this species. Based on homology, the GspD-like T2SS secretin is a strong candidate for the complex. Interestingly, though, we did not identify any secretin-like (or full T2SS-like) particles in the main cell envelope of F. johnsoniae cells. While we could have missed them in the denser periplasm compared to the less-crowded OMEs and OMVs, it is possible that the structures are specifically associated with the formation of OMEs in this species. As these MEs stem only from the OM, there is no IM-embedded energy source for the complex, suggesting that they are not functional secretion systems and raising the question of what function they may serve. It is possible that the OMVs and OMEs form to dispense of the secretin.
These complexes also indicate that MEs/MVs may provide an ideal system to investigate membrane-embedded structures in their native environment at higher resolution. For example, it remains unclear how secretins of various secretion systems are situated within the OM. All high-resolution structures were detergent-solubilized, and most in situ structures have low resolution due to cell thickness (Weaver et al., 2020). Purifying F. johnsoniae OMVs and performing high-resolution subtomogram averaging on the secretin-like complex might shed light on this question.
Early in the history of life, lipid vesicles and elementary protocells likely experienced destabilizing conditions such as repeated cycles of dehydration and rehydration (Damer and Deamer, 2015). The binding of prebiotic amino acids to lipid vesicles can help stabilize them in such conditions (Cornell et al., 2019) and it is conceivable that with billions of years of evolution, variations of these stabilized lipid structures acquired roles that conferred fitness advantages on bacterial species in various environments. Today, the ability of bacteria to extend their membranes to form tubes or vesicles is a widespread phenomenon with many important biological functions. We hope that the structural classification we present here will serve as a helpful reference for future studies in this growing field.
Materials and methods
Strains and growth conditions
Request a detailed protocolHylemonella gracilis cells were grown as described in Kaplan et al., 2020. P. luteoviolacea were grown as described in Shikuma et al., 2014. Magnetospirillum magneticum were grown as described in Cornejo et al., 2016. P. flexibilis 706570 were grown in lactose growth medium. S. oneidensis MR-1 cells were grown, as detailed in Phillips et al., 2020, in Luria Bertani (LB) media under aerobic conditions at 30°C with shaking at 200 rpm until they reached OD600 of ~3. M. xanthus PilY1.3-sfGFP, M. xanthus ΔtsaP, and M. xanthus SA6892 strains were grown as described in Chang et al., 2016. B. burgdorferi B31 ATCC 35210 and H. hepaticus ATCC 51,449 cells were grown in standard media (see Briegel et al., 2009 and references therein).
C. crescentus was cultured in M2G and M2 media (prepared as described in Schrader and Shapiro, 2015); 5 mL of M2G were inoculated with a frozen stock of C. crescentus NA 1000 (wild-type and DpleD mutant cells; see Kaplan et al., 2021c) and grown overnight at 28°C; and 5 mL of the overnight culture was diluted in 15 mL M2G and grown at 28°C with a shaking speed of 200 rpm for ~2 hr until mid-log phase (OD600 0.4–0.5). The sample was then centrifuged at 5200 × g for 6 min at 4°C (same temperature for all subsequent centrifugation steps) and the pellet was resuspended in 1 mL M2 solution. The resuspended cells were transferred into a 2 mL microcentrifuge tube and centrifuged at 5200 × g for 5 min. All but ~250 μL of supernatant was removed, 650 μL M2 was added and the pellet was resuspended, and 900 μL cold Percoll (Sigma Aldrich) was added and the sample was centrifuged at 15,000 × g for 20 min. Samples were taken from the bottom of the tube to select swarmer cells.
Cells of F. johnsoniae strain CJ2618 (a wild-type strain overexpressing FtsZ, ATCC 17061) were taken from a glycerol stock, streaked onto a CYE plate with 10 µg/mL tetracycline and grown at 25°C. Subsequently, 5 mL of motility medium (MM) was inoculated with colonies from the plate and the culture was incubated at 25°C with 80 rpm shaking overnight. Then another 5 mL MM was inoculated with 80 µL of starter culture and placed at 25°C with no shaking until the next day when the cells were harvested and prepared for plunge-freezing.
H. pylori mutants (ΔfliM fliP*, ΔfliO fliP*, ΔflgS fliP*, ΔfliG fliP*, ΔfliQ fliP*) were grown from glycerol stocks on sheep blood agar at 37°C with 5% CO2 for 48 hr and then either plunge-frozen directly or the cells were spread on another plate and left to grow for 24 hr before plunge-freezing. No difference could be discerned between the two samples by cryo-ET.
F. anhuiense (strain 98, see Carrión et al., 2019) and C. pinensis (strain 94, see Carrión et al., 2019) cells were grown overnight in 1/10 TSB at 25°C and 300 rpm shaking in 50 mL cultures. For sample preparation, cells were first concentrated by centrifugation; 3 μL aliquots of the cell suspension were applied to glow-discharged R2/2, 200 mesh copper Quantifoil grids (Quantifoil Micro Tools), the sample was pre-blotted for 30 s, and then blotted for 2.5 s (F. anhuiense) and 1 s (C. pinensis). Grids were pre-blotted and blotted at 20°C and at 95% humidity. Subsequently, the grids were plunge-frozen in liquid ethane using an automated Leica EM GP system (Leica Microsystems) and stored in liquid nitrogen.
Purification of S. oneidensis OMVs
Request a detailed protocolS. oneidensis OMVs were purified as described in Phillips et al., 2020. First, S. oneidensis were grown in LB media until they reached OD600 of 3. Subsequently, the cells were centrifuged at 5000 × g for 20 min at 4°C; the pellet contained whole cells while the supernatant contained the OMVs. To remove any cells present in the supernatant, it was filtered through a 0.45 µm filter. Subsequently, the supernatant was centrifuged at 38,400 × g for 1 hr at 4°C; the OMVs were in the resultant pellet. The pellet was resuspended in 20 mL of 50 mM HEPES pH 6.8 buffer, filtered through a 0.22 µm filter, spun again as described above, and ultimately resuspended in 50 mM HEPES pH 6.8.
Cryo-ET sample preparation and imaging
Request a detailed protocolFor cellular samples, 10 nm gold beads were first coated with BSA (bovine serum albumin) and then mixed with the cells. Subsequently, 4 µL of this mixture was applied to a glow-discharged, thick carbon-coated, R2/2, 200 mesh copper Quantifoil grid (Quantifoil Micro Tools) in an FEI Vitrobot chamber with 100% humidity. Excess fluid was blotted away with filter paper and the grid was plunge-frozen in a mixture of ethane/propane. For the purified OMVs of S. oneidensis, the sample was first diluted to a 0.4 mg/mL concentration before it was applied to the grid (Phillips et al., 2020). Cryo-ET imaging of the samples was done either on an FEI Polara 300 keV field emission gun transmission electron microscope equipped with a Gatan imaging filter and a K2 Summit direct electron detector in counting mode, or a Thermo Fisher Titan Krios 300 keV field emission gun transmission electron microscope equipped with a Gatan imaging filter and a K2 Summit counting electron detector. For data collection, either the UCSF Tomography (Zheng et al., 2007) or SerialEM (Mastronarde, 2005) software was used. For OMVs, tilt series spanned –60° to 60° with an increment of 3°, an underfocus of 1–5 µm, and a cumulative electron dose of 121 e/Å2. For F. johnsoniae, tilt series spanned –55° to 55° with 1° increment, an underfocus of 4 µm, a cumulative electron dose of 100 e/Å2, and a 3.9 Å pixel size. For M. xanthus, tilt-series spanned –60° to 60° with an increment of 1°, an underfocus of 6 µm, and a cumulative electron dose of 180 e/Å2. For B. burgdorferi, tilt series spanned –60° to 60° with 1° increment, an underfocus of 10 µm, and a cumulative electron dose of 160 e/Å2. For H. hepaticus, tilt series spanned –60° to 60° with increments of 1°, an underfocus of 12 µm, and a cumulative electron dose of 165 e/Å2.
F. anhuiense and C. pinensis images were recorded with a Gatan K3 Summit direct electron detector equipped with a Gatan GIF Quantum energy filter with a slit width of 20 eV. Images were taken at magnification corresponding to a pixel size of 3.28 Å (C.pinensis) and 4.4 Å (F. anhuiense). Tilt series were collected using SerialEM with a bidirectional dose-symmetric tilt scheme (–60° to 60°, starting from 0°) with a 2° increment. The defocus was set to – 8 to 10 μm and the cumulative exposure per tilt series was 100 e−/A2. Images were reconstructed with the IMOD software package.
Image processing and subtomogram averaging
Request a detailed protocolReconstruction of tomograms of cellular samples was done using the automatic RAPTOR pipeline implemented in the Jensen lab at Caltech (Ding et al., 2015). Tomograms of purified S. oneidensis OMVs were reconstructed using a combination of ctffind4 (Rohou and Grigorieff, 2015) and the IMOD software package (Kremer et al., 1996). Subtomogram averaging was done using the PEET program (Nicastro, 2006), with twofold symmetry applied along the particle Y-axis.
Data availability
All data generated or analysed during this study are included in the manuscript and supporting files and movies.
References
-
Bacterial nanotubes: a conduit for intercellular molecular tradeCurrent Opinion in Microbiology 42:1–6.https://doi.org/10.1016/j.mib.2017.08.006
-
Through the wall: extracellular vesicles in Gram-positive bacteria, mycobacteria and fungiNature Reviews Microbiology 13:620–630.https://doi.org/10.1038/nrmicro3480
-
The Caltech Tomography Database and Automatic Processing PipelineJournal of Structural Biology 192:279–286.https://doi.org/10.1016/j.jsb.2015.06.016
-
Ecology and evolution of metabolic cross-feeding interactions in bacteriaNatural Product Reports 35:455–488.https://doi.org/10.1039/C8NP00009C
-
Biopearling of Interconnected Outer Membrane Vesicle Chains by a Marine FlavobacteriumApplied and Environmental Microbiology 85:e00829-19.https://doi.org/10.1128/AEM.00829-19
-
Outer Membrane Vesicles (OMVs) of Gram-negative Bacteria: A Perspective UpdateFrontiers in Microbiology 8:1053.https://doi.org/10.3389/fmicb.2017.01053
-
Programmed flagellar ejection in caulobacter crescentus leaves pl-subcomplexesJournal of Molecular Biology 433:167004.https://doi.org/10.1016/j.jmb.2021.167004
-
Computer visualization of three-dimensional image data using IMODJournal of Structural Biology 116:71–76.https://doi.org/10.1006/jsbi.1996.0013
-
Motility and Chemotaxis in Campylobacter and HelicobacterAnnual Review of Microbiology 65:389–410.https://doi.org/10.1146/annurev-micro-090110-102908
-
Membrane vesicles, nanopods and/or nanotubes produced by hyperthermophilic archaea of the genus ThermococcusBiochemical Society Transactions 41:436–442.https://doi.org/10.1042/BST20120293
-
Automated electron microscope tomography using robust prediction of specimen movementsJournal of Structural Biology 152:36–51.https://doi.org/10.1016/j.jsb.2005.07.007
-
Production of Outer Membrane Vesicles and Outer Membrane Tubes by Francisella novicidaJournal of Bacteriology 195:1120–1132.https://doi.org/10.1128/JB.02007-12
-
BookMolecular Organization and Assembly of the Export Apparatus of Flagellar Type III Secretion SystemsBerlin, Heidelberg: Springer Berlin Heidelberg.https://doi.org/10.1007/82_2019_170
-
Characterisation of surface blebbing and membrane vesicles produced by Flavobacterium psychrophilumDiseases of Aquatic Organisms 64:201–209.https://doi.org/10.3354/dao064201
-
Metabolic cross-feeding via intercellular nanotubes among bacteriaNature Communications 6:6238.https://doi.org/10.1038/ncomms7238
-
Bacterial nanotubes as a manifestation of cell deathNature Communications 11:4963.https://doi.org/10.1038/s41467-020-18800-2
-
Vibrio cholerae outer membrane vesicles inhibit bacteriophage infectionJournal of Bacteriology 200:e00792-17.https://doi.org/10.1128/JB.00792-17
-
CTFFIND4: Fast and accurate defocus estimation from electron micrographsJournal of Structural Biology 192:216–221.https://doi.org/10.1016/j.jsb.2015.08.008
-
Synchronization of Caulobacter crescentus for investigation of the bacterial cell cycleJournal of Visualized Experiments 98:52633.https://doi.org/10.3791/52633
-
Outer-membrane vesicles from Gram-negative bacteria: biogenesis and functionsNature Reviews Microbiology 13:605–619.https://doi.org/10.1038/nrmicro3525
-
Types and origins of bacterial membrane vesiclesNature Reviews Microbiology 17:13–24.https://doi.org/10.1038/s41579-018-0112-2
-
Myxobacteria produce outer membrane-enclosed tubes in unstructured environmentsJournal of Bacteriology 196:1807–1814.https://doi.org/10.1128/JB.00850-13
-
Structure of a full-length bacterial polysaccharide co-polymeraseNature Communications 12:369.https://doi.org/10.1038/s41467-020-20579-1
Article and author information
Author details
Funding
National Institutes of Health (R35 GM122588)
- Grant J Jensen
California Institute of Technology (Baxter postdoctoral fellowship)
- Mohammed Kaplan
Nederlandse Organisatie voor Wetenschappelijk Onderzoek (NWO OCENW.GROOT.2019.063)
- Ariane Briegel
National Institutes of Health (P20 GM130456)
- Carrie L Shaffer
Nederlandse Organisatie voor Wetenschappelijk Onderzoek (184.034.014)
- Ariane Briegel
The funders had no role in study design, data collection and interpretation, or the decision to submit the work for publication.
Acknowledgements
This project was funded by the NIH (grant R35 GM122588 to GJJ, and P20 GM130456 to CLS) and a Baxter postdoctoral fellowship from Caltech to MK. Cryo-ET work was done in the Beckman Institute Resource Center for Transmission Electron Microscopy at the California Institute of Technology. We are grateful to Prof. Martin Pilhofer for collecting the P. luteoviolacea data and for critically reading the manuscript. We thank Prof. Elitza I Tocheva for collecting the D. acidovorans data. We thank Prof. Mohamed El-Naggar for insights into preparing S. oneidensis samples and Dr. Yuxi Liu for discussions. Briegel lab data was collected at the Netherlands Center for Electron Nanoscopy with support from Dr Wen Yang. This data was collected with support from the National Roadmap for Large-Scale Research Infrastructure 2017–2018 with project number 184.034.014, which is financed in part by the Dutch Research Council (NWO). This work was also supported by the NWO OCENW.GROOT.2019.063 grant.
Copyright
© 2021, Kaplan et al.
This article is distributed under the terms of the Creative Commons Attribution License, which permits unrestricted use and redistribution provided that the original author and source are credited.
Metrics
-
- 5,736
- views
-
- 712
- downloads
-
- 30
- citations
Views, downloads and citations are aggregated across all versions of this paper published by eLife.
Citations by DOI
-
- 30
- citations for umbrella DOI https://doi.org/10.7554/eLife.73099