Palatal morphology predicts the paleobiology of early salamanders
Abstract
Ecological preferences and life history strategies have enormous impacts on the evolution and phenotypic diversity of salamanders, but the yet established reliable ecological indicators from bony skeletons hinder investigations into the paleobiology of early salamanders. Here, we statistically demonstrate by using time-calibrated cladograms and geometric morphometric analysis on 71 specimens in 36 species, that both the shape of the palate and many non-shape covariates particularly associated with vomerine teeth are ecologically informative in early stem- and basal crown-group salamanders. Disparity patterns within the morphospace of the palate in ecological preferences, life history strategies, and taxonomic affiliations were analyzed in detail, and evolutionary rates and ancestral states of the palate were reconstructed. Our results show that the palate is heavily impacted by convergence constrained by feeding mechanisms and also exhibits clear stepwise evolutionary patterns with alternative phenotypic configurations to cope with similar functional demand. Salamanders are diversified ecologically before the Middle Jurassic and achieved all their present ecological preferences in the Early Cretaceous. Our results reveal that the last common ancestor of all salamanders share with other modern amphibians a unified biphasic ecological preference, and metamorphosis is significant in the expansion of ecomorphospace of the palate in early salamanders.
Editor's evaluation
This paper is a valuable contribution to evolutionary ecomorphology in extant and extinct tetrapods, and of interest to vertebrate paleontologists and other evolutionary biologists interested in the early evolution of amphibians. Using geometric morphometric analysis, the authors demonstrate that both the shape of the palate and several non-shape variables (particularly associated with vomerine teeth) are ecologically informative in early stem- and basal crown-group salamanders. The study also reveals that metamorphosis is significant in the expansion of ecomorphospace of the palate in early salamanders.
https://doi.org/10.7554/eLife.76864.sa0Introduction
Salamanders, anurans, and caecilians are highly distinctive from one another in their morphology in both living species and their respective oldest known relatives from the Triassic (Ivachnenko, 1978; Ascarrunz et al., 2016; Pardo et al., 2017a; Schoch et al., 2020; Kligman et al., 2021). As a result, the evolutionary origin(s) of modern amphibians have remained controversial since the late 19th century (Haeckel, 1866), with a number of extinct tetrapod groups in different ecological types at adult stages having been hypothesized as their purported ancestors, including: amphibamid (terrestrial) and branchiosaurid (terrestrial and aquatic) dissorophoid temnospondyls (Laurin et al., 2004; Fröbisch and Schoch, 2009; Maddin and Anderson, 2012; Pardo et al., 2017b), stereospondylian (semiaquatic/aquatic) temnospondyls (Schoch and Milner, 2014; Pardo et al., 2017a), and several groups of lepospondyls (aquatic, semiaquatic or terrestrial; Marjanović and Laurin, 2013; Marjanović and Laurin, 2019; Jansen and Marjanović, 2021; Laurin et al., 2022). The specialized morphologies in modern amphibians are greatly impacted by ecology and their complex life history strategies (e.g. Wake, 2009), for example, even the earliest anuran Triadobatrachus and the possible caecilian Chinlestegophis from the Triassic display several morphological specializations as their living relatives for aboveground and subterranean terrestrial living settings, respectively. Salamanders (or Caudata, the total group), on the other hand, have a more conservative body plan and more diversified ecological preferences when compared to anurans and caecilians (Deban and Wake, 2000; Bonett and Blair, 2017; Fabre et al., 2020), and have been frequently used as comparative analogues for inferring the paleoecology of extinct tetrapods (Schoch and Fröbisch, 2006; Fröbisch and Schoch, 2009). However, the evolutionary paleoecology in modern amphibians and particularly in early salamanders has received insufficient attention.
Cryptobranchoidea is the sister group of all other crown group salamanders (Urodela) and contains two subclades: Pancryptobrancha (total group cryptobranchids; Vasilyan et al., 2013) and Panhynobia (total group hynobiids; Jia et al., 2021a). The two subclades are united by a set of synapomorphies (Dunn, 1922; Estes, 1981; Jia et al., 2021a), but are different from each other in life history strategies and ecological preferences at their respective adult stage: most pancryptobranchans are neotenic or partially metamorphosed and live in water permanently by retaining larval features (e.g. gill slits), albeit the pancryptobranchan Aviturus from the Paleocene was interpreted as semiaquatic with an unknown life history strategy (Vasilyan and Böhme, 2012; but see Skutschas et al., 2018). In contrast, panhynobians are predominantly metamorphosed, except that the stem hynobiid Regalerpeton from Early Cretaceous (Rong, 2018) and some populations of the living hynobiid Batrachuperus londongensis are neotenic (Jiang et al., 2018). Postmetamorphosed hynobiids have lost larval features and are characterized by an anterolaterally directed palatal ramus of the pterygoid, and are able to live in water (e.g. Paradactylodon), on land (e.g. Hynobius) or are semiaquatic (Ranodon) outside of the breeding season (Kuzmin and Thiesmeier, 2001; Fei et al., 2006; Materials and methods).
Cryptobranchoidea are critical in understanding the paleoecology of early salamanders because the earliest known cryptobranchoids from the Middle Jurassic (Bathonian) have higher disparities in both life history strategies and ecological preferences than stem urodeles, and represent the oldest known crown urodeles, including ‘Kirtlington salamander B’ from the UK, Kiyatriton krasnolutskii from Russia, and Chunerpeton, Neimengtriton, and Jeholotriton from China (Evans and Milner, 1994; Gao et al., 2013; Skutschas, 2015; Jia et al., 2021a). Both Chunerpeton and Jeholotriton are neotenic as confirmed by the presence of external gills and a tall caudal dorsal fin in adult specimens (Gao and Shubin, 2003; Wang and Rose, 2005), whereas Neimengtriton is the oldest metamorphosed and semiaquatic cryptobranchoid (Jia et al., 2021a; see below). The ‘Kirtlington salamander B’ and K. krasnolutskii are both represented by fragmentary materials and their paleoecology unfortunately remains unknown. In contrast, other contemporaries (e.g. Kokartus, Marmorerpeton) from the Middle Jurassic (Bathonian) of UK, Russia, and Kyrgyzstan are all neotenic and aquatic at their adult stage, and have been classified as stem urodeles by the absence of spinal nerve foramina in the atlas that characterizes Urodela (Ivachnenko, 1978; Evans et al., 1988; Skutschas and Krasnolutskii, 2011; Skutschas and Martin, 2011; Skutschas, 2013; Skutschas et al., 2020). The only known pre-Jurassic stem urodele, Triassurus from the Middle/Upper Triassic of Kyrgyzstan, is merely represented by two larval specimens with no clue to its paleoecology at adult stage (Schoch et al., 2020).
To date, seven other basal cryptobranchoids have been reported from the Upper Jurassic to Lower Cretaceous of northern China: Laccotriton, Liaoxitriton, Linglongtriton, Nuominerpeton, Pangerpeton, Regalerpeton, and Sinerpeton, most of which are represented by articulated specimens and have been recently recovered as stem hynobiids or hynobiid-like taxa (see Gao et al., 2013; Jia and Gao, 2016; Jia and Gao, 2019; Jia et al., 2021a). Besides the neotenic Regalerpeton as aforementioned, habitat preferences of these metamorphosed taxa and paleoecological disparity patterns of Cryptobranchoidea remain largely unexplored mainly due to yet established osteological indicators for ecology (see Discussion). The configuration of vomerine teeth has long been identified as useful for the classification of living cryptobranchoids (Zhao and Hu, 1984) and was recently claimed to be ecologically informative (Jia et al., 2021b), but such statements have not received rigorous tests with inclusion of fossil taxa.
Our series of studies on living and fossil cryptobranchoids noticed that besides the vomerine teeth, the palate varies in shape and proportion, and could potentially serve as an indicator for paleoecological reconstruction (Jiang et al., 2018; Jia et al., 2019; Jia et al., 2021b; Figure 1 and Figure 1—figure supplements 1–23). To test these hypotheses and to address the constraints underlying the morphological disparity of the palate, here we conducted a 2D landmark-based geometric morphometric analysis on the palate of all living and most aforementioned fossil genera of cryptobranchoids, stem, and other basal crown urodeles based primarily on micro-CT scanned specimens. We statistically investigated disparity patterns within the morphospace of the palate with respect to ecological preferences, life history strategies, and taxonomic affiliations. Based on a time-calibrated cladogram we established for fossil and living cryptobranchoids (Jetz and Pyron, 2018; Jia et al., 2021a), we further quantified the evolutionary rate of the palate and reconstructed the ancestral states for ecological preferences, life history strategies, palate shape, and vomerine tooth configurations of the respective last common ancestor of Panhynobia, Pancryptobrancha, Cryptobranchoidea, Urodela, and Caudata. We demonstrate that the palate is a reliable proxy in ecological reconstructions for early salamanders, and the morphospace of the palate is predominantly shaped by ecological constraints and also displays a stepwise evolutionary pattern.
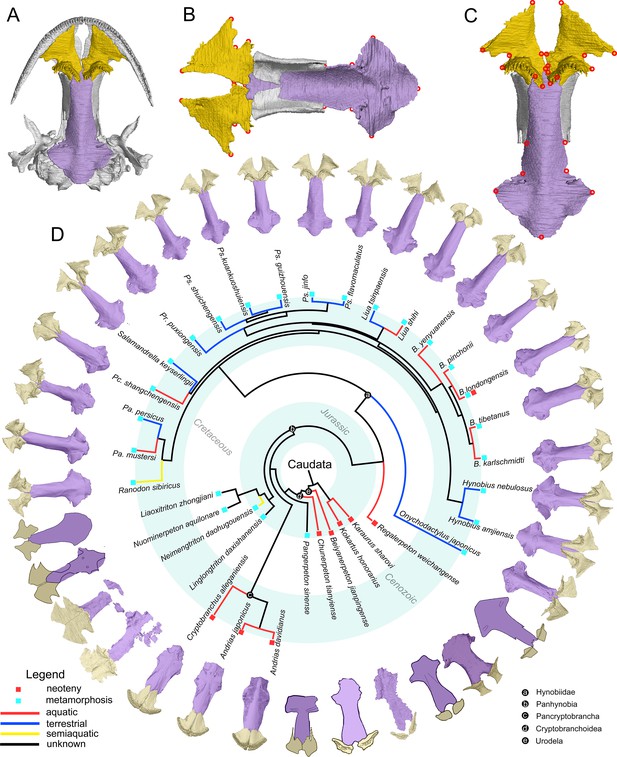
The palate and phylogenic relationships of early salamanders.
(A) The vomer (gold) and parasphenoid (purple) of the palate in ventral view of the skull in living hynobiid Pseudohynobius flavomaculatus. (B) Dorsal view of the palate showing the articulation patterns with the paired orbitosphenoid (whitish). (C) Enlarged view of the palate in ventral view with red circles corresponding to the 24 landmarks used for the geometric morphometric analysis. (D) Palatal configurations of early salamanders in ventral view, with color-coded life history strategies (square block) and ecological preferences (line) plotted on the time-calibrated tree modified from Jetz and Pyron, 2018 and Jia et al., 2021a.
Results
In ventral view of the palate, the anteromedial fenestra is present between the vomer and the upper jaw in most early salamanders and is only absent in living cryptobranchids (Figure 1—figure supplements 1–23). The paired vomers medially articulate with each other in most taxa and posteriorly overlap to different extents, the anterior part of the cultriform process of the parasphenoid and/or the orbitosphenoid. The teeth are closely packed as a continuous tooth row positioned along the anterolateral periphery of the vomer in cryptobranchids but have diversified configurations in other taxa. The parasphenoid is a sword-like, azygous bony plate with its anterior part articulating dorsally with the orbitosphenoid and its posterior part flooring the otic capsule.
Morphospace and shape disparity patterns of the palate in early salamanders
The palate is symmetric about the mid-sagittal plane of the skull with symmetric shape components accounting for 96.15% of the total shape variation in 70 specimens, and the left-right asymmetry accounting for the remaining 3.85%. The shape and the size of the palate with the latter represented by the centroid size (CS), are significantly correlated as revealed by the standard multivariate regression between log (CS) (independent variable) and symmetric shape components (dependent variable) across 70 specimens (R2 = 9.3331%; p<0.001; F = 6.9977; Z = 3.918) and 34 species (R2 = 13.276%; p<0.001; F = 4.8985; Z = 3.1691). However, when phylogenetic relationships of the 34 species were factored in, the association between size and shape of the palate is no longer significant as shown in the evolutionary allometry analysis (p=0.1583). Such inconsistency between standard and evolutionary allometry analyses is related to the fact that the CS of the palate has a strong phylogenetic signal (Blomberg’s K = 0.997, p=0.001, Z = 4.1921) and, hence, the CS accounts for an even smaller amount of shape variations of the palate (R2 = 4.494%; F = 1.5059; Z = 1.0141) when evolutionary history among species was counterbalanced in the evolutionary allometry analysis. To eliminate impacts from both asymmetry and allometry on the spatial patterns of the palate, residuals from the multivariate regression of symmetric shape components on log (CS) were retained for downstream statistical analyses.
To visualize the spatial patterns of the palate in the morphospace, on the basis of the size-corrected (allometry-free) 24-landmark dataset, we conducted a standard principal component analysis (PCA) across 70 specimens; and we also conducted three other types of PCA across 34 species with the time-calibrated cladogram and ancestral internal nodes projected into the morphospace (Figure 2—figure supplements 1–3), including: a phylomorphospace analysis (PA), phylogenetic principal component analysis (Phylo-PCA) and a phylogenetically-aligned components analysis (PaCA). The first three PC axes in each of the four PCAs collectively measure up to about 70% of total shape variances (Supplementary file 1A). Within the phylomorphospace defined by principal components (PCs) 1–2 (Figure 2B and Figure 2—figure supplement 1b), aquatic and terrestrial living species of Cryptobranchoidea are generally located along the positive and negative interval of the PC 2 axis (21.35%), respectively. Most taxa are ecologically exclusive at the genus level, except that Liua and Paradactylodon are the only two living hynobiid genera with species occupying both the aquatic (Liua shihi and Paradactylodon mustersi) and terrestrial (Liua tsinpaensis and Paradactylodon persicus) zones. The living semiaquatic hynobiid Ranodon sibiricus occupies at a location intermediate between the aquatic and terrestrial zones. Shape changes of the palate relative to the mean shape of all terminal and internal taxa along the positive values of PC 2 (aquatic zone) involve an anteromedial extension of the vomer and therefore a reduction of the anteromedial fenestra, a shrinkage of the anterolateral and posterolateral borders of the vomer and the width of the cultriform process of the parasphenoid, and an anterior extension of the parasphenoid. In contrast, the negative values of PC 2 (terrestrial zone) characterize a shrinkage of the anteromedial border of the vomer and therefore a posterolaterally expanding anteromedial fenestra, a laterally widening retrochoanal process of the vomer and the cultriform process of the parasphenoid, and an anteroposteriorly shortening parasphenoid. On the other hand, basalmost crown urodeles (e.g. Beiyanerpeton, Chunerpeton, and Pangerpeton) and karaurids are largely separated from living taxa along the PC 1 axis (37.49%), with stem hynobiids widely scattered in the phylomorphospace and lying within either the aquatic (Liaoxitriton) or terrestrial (Linglongtriton and Nuominerpeton) zone. The only known aquatic stem hynobiid Regalerpeton is situated at a region of the aquatic zone occupied by other aquatic fossil taxa (e.g. Beiyanerpeton and Chunerpeton), whereas the semiaquatic stem hynobiid Neimengtriton occupies a location closer to terrestrial than either aquatic zone or the semiaquatic zone of extant hynobiids. From the largest to the smallest value of PC 1, both the vomer and the parasphenoid have an anteroposterior extension and the cultriform process of the parasphenoid changes from an anteriorly widened plate with an indented anterior edge into a bilaterally narrowed plate with a pointed anterior edge.
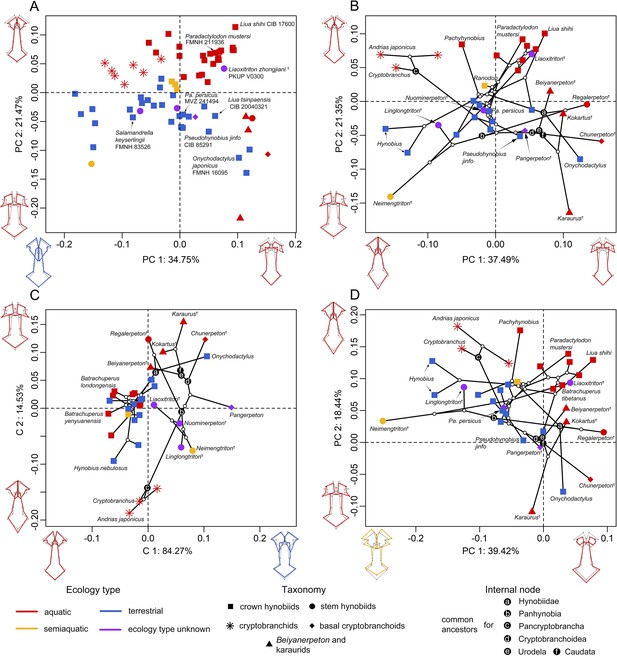
Spatial patterns of the palatal shape in the morphospace defined by the first two components generated from four principal component analyses (PCA).
(A) Standard PCA across 70 specimens, (B) phylomorphospace analysis, (C) phylogenetically aligned components analysis, and (D) phylogenetic PCA across 34 species with ancestral states for internal nodes (open circles) and phylogenetic relationships (black lines) plotted in the morphospace. The color and shape of each point represent the ecological type and taxonomic affiliation, respectively. Extreme values of the palatal shape along both principal components (PCs) 1 and 2 are represented by wireframes color-coded to ecological types against the mean shape (gray) of both terminal and internal taxa.
The evolutionary history among species has a moderate but significant contribution in the formation of the spatial patterns in the phylomorphospace (phylogenetic signal: observed Kmult = 0.4154, p=0.001, Z = 4.9856). When the multivariate shape data of the palate were maximally aligned with phylogenetic signal (Figure 2C and Figure 2—figure supplement 1c), fossil taxa can be roughly divided from living taxa along PaCA-C 1. Both crown and stem taxa of Panhynobia are more compactly clustered than that seen in the phylomorphospace created by PA, and are distinct from the Pancryptobrancha and the region occupied by karaurids, basal cryptobranchoids and salamandroids; but taxa in different types of ecological preference are mixed together. By contrast, when the phylogenetic signals of the palate were eliminated by the Phylo-PCA (Figure 2D and Figure 2—figure supplement 1d), the overall spatial patterns among species are essentially preserved, albeit slightly rotated clockwise, as observed in the phylomorphospace. In this phylogeny-free morphospace, the last common ancestors of Pancryptobrancha and of Hynobiidae lie within the aquatic zone and are tightly associated with living cryptobranchids and the stem urodele Kokartus, respectively. In contrast, the last common ancestors of Panhynobia, Cryptobranchoidea, Urodela, and Caudata lie within the terrestrial zone and are adjacent to three highly derived living hynobiids, respectively, Pseudohynobius shuichengensis, Pseudohynobius jinfo, and Liua tsinpaensis.
Our pairwise comparison (Supplementary file 1B–E) and phylogenetic Procrustes ANOVA reinforce that the shape of the palate is significantly different among groups of species that are classified by ecological preference (R2 = 28.079%; p=0.001; F = 4.3740; Z = 3.4838) and life history strategy (R2 = 6.797%; p=0.006; F = 3.1766; Z = 2.4673), but not by taxonomic affiliations, neither at the genus (R2 = 68.711%; p=0.248; F = 1.2549; Z = 0.78492) nor family (R2 = 12.858%; p=0.581; F = 0.8263; Z = –0.21234) level. With regard to the life history strategy, living cryptobranchids are separated from all Mesozoic neotenic taxa including Beiyanerpeton, Chunerpeton, karaurids, and Regalerpeton by the vast majority of the metamorphosed taxa, which occupy most of the morphospace of the palate (Figure 2—figure supplement 4). The single living neotenic hynobiid B. londongensis is situated between the two neotenic groups as aforementioned and lies alongside its metamorphosed conspecifics and those all collectively overlap with other aquatic hynobiids. When the seven-landmark-dataset for the right vomer was analyzed following the same procedure, the purported semiaquatic basal pancryptobranchan Aviturus is nested within the terrestrial zone along with living metamorphosed taxa (Figure 2—figure supplement 5).
Evolutionary rates of the palate
The palate exhibits considerable differences in morphological disparity and evolutionary rates across regions represented by the 24-landmark points as calculated under a Brownian motion evolutionary model (Figure 3; Supplementary file 1B–H). The vomer evolves about two times faster than the parasphenoid, and such a pattern is also supported by the fact that the vomer has a higher phylogenetic signal (Kmult = 0.6407) than that of the palate (Kmult = 0.4154). The posterior border of the vomer and the medial-most part of the choanal notch are the fastest and the slowest evolving parts in the palate, respectively. In the parasphenoid, however, the highest evolutionary rates are concentrated on the anterior end, posterior end and the lateral alae, and the lowest evolutionary rates are located at the posterior contact between the cultriform process and the orbitosphenoid and the junction area where the cultriform process merges with the lateral alae.
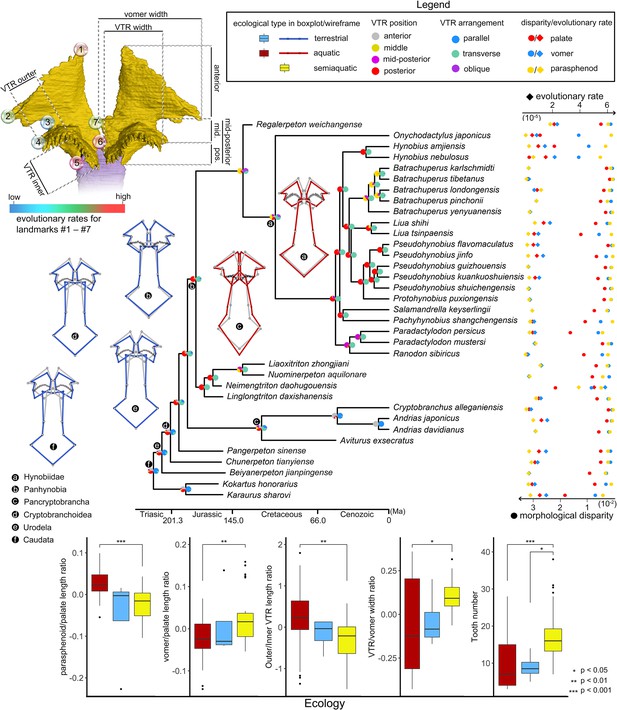
Evolutionary patterns of both the shape and non-shape covariates of the palate and their association with ecological disparity in early salamanders.
Ancestral shape (wireframes color-coded to ecological types superimposed with mean shape [gray]) and vomerine tooth row (zigzag black lines) configurations are reconstructed for respective last common ancestors of Hynobiidae, Panhynobia, Pancryptobrancha, Cryptobranchoidea, Urodela, and Caudata. A complete list of evolutionary rates for each of the 24 landmarks, the vomer, parasphenoid, the palate and the continuous covariates of the vomer across 34 species is available in Supplementary file 1GH and J. The two pie charts at each internal node of the time-calibrated cladogram are likelihoods of the position (left) and arrangement (right) of the vomerine tooth row reconstructed in this study. Continuous covariates of the vomer were subjected to Mann-Whitney U test for their association with the three ecological groups with corresponding p values labeled above the boxplots.
Species in different ecological (p=0.002, Z = 2.6284) and taxonomic (p=0.035, Z = 1.7594) groups vary greatly in evolutionary rate of the palate (Supplementary file 1H). Namely, aquatic and terrestrial taxa are comparable with each other in the evolutionary rate of the palate, the vomer and the parasphenoid, and both of them evolve at a rate that is less than half of that in semiaquatic taxa championed by Neimengtriton. Stem hynobiids have the highest evolutionary rates, followed successively by basal cryptobranchoids, pancryptobranchans, crown hynobiids, and non-cryptobranchoid basal salamanders. Neotenic and metamorphosed taxa are not significantly different from each other in evolutionary rates (p=0.843, Z = –1.1341).
Non-shape covariates from the vomer
Similar to shape variables, allometry has a significant impact on the first 4 of the 5 continuous non-shape covariates of the palate (length ratios between parasphenoid and palate, and that between vomer and palate; width ratios between vomerine tooth row [VTR] and vomer, and between outer and inner branches of the VTR; and vomerine tooth number) when each of them is statistically regressed on the log (CS). We thus use residuals from the regression for boxplots to visualize the distribution patterns of the covariates in three ecological groups across 70 specimens, and use the Mann-Whitney U-test to analyze if the covariates are reliable ecological indicators (Figure 3). In line with shape changes revealed from PCA, aquatic and terrestrial species are significantly different in all of the five covariates, with aquatic taxa having proportionally a longer parasphenoid (median: ~0.84), a shorter vomer (median: ~0.28), a higher ratio in outer/inner branch of the VTR (median: ~1.6), a narrower VTR (except neotenic taxa; median: ~0.37) and fewer vomerine teeth (median: 7) than that of terrestrial taxa (i.e. ~0.78, ~0.33, ~0.58, ~0.64, and 16). Semiaquatic species cannot be confidently differentiated from aquatic or terrestrial taxa in any of the five covariates, but interestingly their parasphenoid length and VTR width resemble those in terrestrial taxa and their vomer length, outer/inner branch VTR width ratio and teeth numbers are more similar to aquatic taxa. The contingency table and Cochran-Mantel-Haenszel statistical test (Figure 3—figure supplement 1 and Supplementary file 1I) show that the arrangement and position of VTR are both significantly correlated to ecological preference and life history strategy. The VTR in anterior and middle position of the vomer or being arranged obliquely or parallel to the marginal tooth row are only present in aquatic taxa, with the VTR located anteriorly exclusively present in neotenic species. The VTR in the mid-posterior position of the vomer or when it is transversely arranged is only present in metamorphosed taxa with all kinds of ecological preferences. Species with VTR in the posterior position or transversely arranged are more likely to be terrestrial than aquatic or semiaquatic.
Ancestral states of the palate
The ancestral states in both the shape and non-shape covariates of the palate were reconstructed for the last common ancestors of Hynobiidae, Panhynobia, Pancryptobrancha, Cryptobranchoidea, Urodela, and Caudata, respectively, using maximum likelihood (Supplementary file 1J). When compared to the mean shape of the palate, the configurations of the palate in the respective last common ancestors of Caudata, Urodela, and Cryptobranchoidea are characterized by a shortened parasphenoid with an anteriorly widened cultriform process and an anteriorly shortened but posteriorly widened vomer. This configuration coincides with our reconstructions of the respective last common ancestors of Caudata, Urodela, and Cryptobranchoidea is parallel to the marginal tooth row and is located in the posterior part of the vomer with the outer longer than the inner branch. The VTR retained a similar configuration, except for being transversely arranged in the last common ancestors of Panhynobia and Pancryptobrancha and then shifting to the middle part of the vomer in the last common ancestor of Hynobiidae.
Discussion
Among modern amphibians, anurans, and caecilians undergo metamorphosis or direct development, and the adults of most species are terrestrial. In contrast, salamanders have their ecological preference decoupled from life history strategy, especially in metamorphosed taxa such as living and extinct hynobiids where postmetamorphosed adults live in water, on land or are semiaquatic (Fei et al., 2006; Jia and Gao, 2016; Jia et al., 2021a). The discrepancy in ecological preference between salamanders on one hand and anurans and caecilians on the other hand is unhelpful in understanding the evolutionary paleoecology in the early lissamphibians given that salamanders and anurans are sister-groups. Previous studies on fossil salamanders generally focus on the taxonomy, whereas the paleoecology and the evolutionary history of the ecological decoupling particularly in metamorphosed taxa had received insufficient attention, which is to some extent explained by the insufficient taphonomic analyses on fossil sites with salamander discoveries, such as the Daohugou fossil locality (Wang et al., 2019). The main obstacles are (1) sufficient and reliable ecological indicators in the bony skeleton have not yet been established for extant cryptobranchoids and early salamanders (Xiong et al., 2016; Jia et al., 2021b), and (2) soft anatomical structures (e.g. labial fold and caudal fin) and stomach contents that are ecologically informative, as commonly seen in lacustrine deposits of certain neotenic species (Dong et al., 2011), are rarely preserved in metamorphosed individuals. Here, we quantitively investigate the paleoecological turnover in the earliest known salamanders based on the shape and non-shape variables of the palate. Our results shed light on the interactions between morphology and paleoecology, and the underlying mechanisms governing ecomorphological diversity among early salamanders along the rise of modern amphibians.
As shown in the phylomorphospace, the shape of the palate in early salamanders is heavily impacted by convergence resulting from ecological disparity, as extant cryptobranchoids and fossil taxa in different ecological preferences occupy distinctive zones in the morphospace. Such spatial patterns remain essentially unaltered after phylogenetic signals are eliminated by the Phylo-PCA, along the first axis of which aquatic and terrestrial taxa regardless of their phylogenetic closeness are separated from each other, confirming our hypothesis that the shape of the palate is a reliable ecological indicator in early salamanders as reported in living salamanders (Fabre et al., 2020). As shown above, non-shape covariates of the palate particularly with regard to the VTR are also ecologically informative. The palate in aquatic species is characterized by a reduction of the anteromedial fenestra, a shrinkage of the vomer laterally and anteroposteriorly with fewer vomerine teeth, a high ratio of the outer to the inner branch of the VTR, a narrow VTR, and an elongated parasphenoid. On the other hand, the palate in terrestrial species is characterized by a posterolateral expansion of the anteromedial fenestra, an anteroposteriorly elongated vomer and a widened retrochoanal process with more vomerine teeth, a low ratio of the outer to inner branch of the VTR, a widened VTR, and an anteroposteriorly shortened parasphenoid. The palate in extant semiaquatic taxa is transitional in both the morphospace and many non-shape covariates between aquatic and terrestrial taxa as mentioned above, whereas the palate of the only known fossil semiaquatic salamander, Neimengtriton (Middle Jurassic) is configured more like that of terrestrial taxa as argued in the original study (Jia et al., 2021a), indicating that Neimengtriton is probably more adapted to terrestrial environments despite being semiaquatic. The respective last common ancestors for Caudata, Urodela, Cryptobranchoidea, and Panhynobia, the basal cryptobranchoid Pangerpeton (Late Jurassic) and the stem hynobiids Linglongtriton (Late Jurassic) and Nuominerpeton (Early Cretaceous) are terrestrial, whereas other stem hynobiids Liaoxitriton and Regalerpeton (Early Cretaceous) and the respective last common ancestors for Hynobiidae and Pancryptobrancha are aquatic, demonstrating that ecological shifts occurred frequently in the evolution of early salamanders. The Paleocene pancryptobranchan Aviturus is likely metamorphosed and terrestrial based on the shape and non-shape variables of the vomer, but this hypothesis awaits to be tested by the discovery of a more completely preserved palate.
Morphological adaptations in the palate and perhaps in other cranial features (e.g. hyobranchial apparatus; see Jia et al., 2021b) to aquatic and terrestrial living settings are likely constrained by feeding mechanisms (Regal, 1966; Reilly and Lauder, 1990). In extant cryptobranchoids, terrestrial species grasp prey items with their jaws and/or by their tongue protruding from the mouth; the captured prey is then transported from the snout into the esophagus with the assistance of several cyclical movements of tongue and hyobranchial apparatus that press and reposition the prey against the palate (Deban and Wake, 2000; Wake and Deban, 2000). Such feeding mechanisms in terrestrial cryptobranchoids demand a sticky tongue moisturized by the intermaxillary or internasal gland that is housed above an expanded anteromedial fenestra, a wide snout contributed by lateral expansions of the vomer, many vomerine teeth to efficiently hold the prey in place, and a shortened parasphenoid to reduce the distance of intraoral transportation. The posteriorly elongated inner branch of the VTR as represented by derived terrestrial hynobiids Hynobius and Salamandrella would serve as toothed surfaces that can further facilitate tongue manipulations and transportations of small prey items posteriorly as in more sophisticated terrestrial feeders (e.g. salamandrids and plethodontids; Regal, 1966). By contrast, aquatic salamander species capture their prey primarily by suction feeding in which the prey is carried into the biting range of the jaws by water currents created by retraction and expansion of the buccal walls, and giant salamanders can even perform asymmetrical strikes in water via unilateral jaw and hyobranchial movements (Gillis and Lauder, 1994). Intraoral transport of the prey is more efficient in aquatic than in terrestrial species; and it typically involves repeating the same procedure as in the initial suction strike and is occasionally assisted by tongue manipulations against the palate (Regal, 1966). The limited usage of the tongue in aquatic prey capture diminishes the need for moisturization from the intermaxillary or internasal gland which, in turn, may lead to reduction and closure of the anteromedial fenestra as seen in aquatic species. An anteroposterior expansion of the parasphenoid increases buccal volume, and the shrinkage of the vomer along both the mediolateral and anteroposterior dimensions reduces snout size; both features act in concert to boost the success of feeding attempts by creating a high negative buccal pressure. A narrow VTR with few teeth, most of which are located on the outer branch, likely serves to reduce hindering the influx of water and prey.
Whichever mode of prey capture and intraoral transportation cryptobranchoids perform, the palate undergoes a similar mechanical stress distribution pattern as found in species with different types of ecological preference and life history strategy (Fortuny et al., 2015: Andrias davidianus; Zhou et al., 2017: Salamandrella keyserlingii). Such findings reinforce that alternative morphological configurations of certain features in the palate cope well with similar functional demands, but do not necessarily indicate that other feeding-related features of the palate and other regions of the cranium would also exhibit such extensive convergence. For example, the ceratohyal has been argued to be ecologically informative in extant cryptobranchoids, as the ceratohyal is consistently ossified at its posterior end in aquatic species but remains cartilaginous in terrestrial taxa (Xiong et al., 2016; Jia et al., 2021b); however, the ceratohyal remains cartilaginous in all known early fossil stem and crown urodeles, and ossifications of the ceratohyal must represent a derived feature independently evolved in crown cryptobranchoids (Jia et al., 2021b). Interestingly, the palate of extant cryptobranchoids as shown in biomechanical analyses (Fortuny et al., 2015; Zhou et al., 2017) has the highest stress level concentrated in the most posterior part of the vomer and its sutural area with the parasphenoid and orbitosphenoid, which surprisingly correspond to the place with the highest evolutionary rate in the palate as revealed by our study. Here, we recognize a clear stepwise evolutionary pattern at the vomer-parasphenoid-orbitosphenoid sutural area in early salamanders (Figure 4). In stem (Karaurus and Kokartus) and many basal crown (Beiyanerpeton, Chunerpeton, Jeholotriton, Neimengtriton, Pangerpeton, and Qinglongtriton) urodeles from the Jurassic and the reconstructed respective last common ancestors for Panhynobia, Cryptobranchoidea, Urodela, and Caudata, the vomer has a limited contact with the parasphenoid, the cultriform process of the parasphenoid is anteriorly expanded bilaterally, and the orbitosphenoid has no anteroventral processes (see below) or in the case of Qinglongtriton remains completely cartilaginous. In three stem hynobiids (Liaoxitriton, Nuominerpeton, and Regalerpeton) and the reconstructed ancestors for Hynobiidae and Pancryptobrancha from the Cretaceous and another stem hynobiid Linglongtriton from the Late Jurassic, the vomer has an increased contact with the parasphenoid, the cultriform process of the parasphenoid is anteriorly constricted, and the orbitosphenoid lacks the anteroventral process. In living cryptobranchoids, the vomer is in more extensive contact with the parasphenoid and orbitosphenoid (Trueb, 1993: Cryptobranchus; Kuzmin and Thiesmeier, 2001: Ranodon; Jiang et al., 2018: Batrachuperus; Jia et al., 2021b: Pseudohynobius), the cultriform process of the parasphenoid is anteriorly constricted bilaterally, and the orbitosphenoid has a thick anteroventral process ossified from neighboring cartilages in the nasal capsule that projects medially and firmly overlaps the cultriform process of the parasphenoid. If similar biomechanical stress patterns existed in early salamanders, the stress and strain applying to the vomer/parasphenoid sutural area would be equally offset by either a wide and thin cultriform process of the parasphenoid with limited support from the vomer and the absence of anteroventral process of the orbitosphenoid as seen in Jurassic taxa, or a narrow cultriform process thickened by more extensive overlapping with the vomer as seen in Cretaceous and living taxa, and by ossification of the anteroventral process of the orbitosphenoid as seen solely in living taxa.
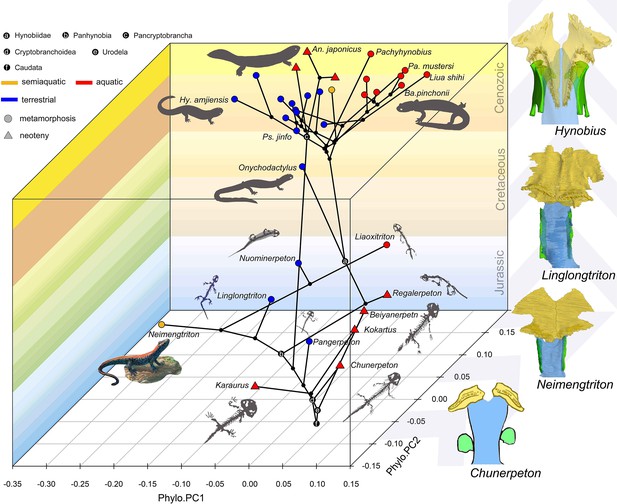
Spatial-temporal patterns of phenotypic diversities of the palate and their associations with ecological preference and life history strategy in early salamanders and the stepwise evolutionary patterns at the sutural area of vomer, parasphenoid, and orbitosphenoid.
The morphospace of the palate is defined within a space formed by geological time scale (Z-axis) and principal components (PCs) 1 (X-axis) and 2 (Y-axis) derived from phylogenetic principal component analysis across 34 species. All silhouettes and images of salamander species are original.
It is worth emphasizing that the phenotypic diversity of the palate is variously affected by neoteny and metamorphosis. Neoteny is notable in producing convergent characters through truncation of normal developmental trajectories and its indirect role associated with constraints from the aquatic environment (Wiens et al., 2005). Most early stem and crown urodeles are neotenic (e.g. Chunerpeton and Kokartus) and their phenotypic diversity of the palate is truly confined to a restricted area in the spatial-temporal morphospace. However, extant neotenic cryptobranchids and the hynobiid B. londongensis bear an increased phenotypic diversity of the palate as compared to fossil neotenic taxa, and are separated from one another within the morphospace with the neotenic B. londongensis even overlapping with its metamorphosed conspecifics, indicating that constraints imposed by ecology have more influence than neoteny in the morphogenesis of the palate. The ecomorphospace of the palate is indeed greatly and rapidly expanded by metamorphosed taxa represented by basal cryptobranchoids and most stem hynobiids as these taxa have the highest evolutionary rate in the palate (Supplementary file 1H). The last common ancestors of Caudata, Urodela, and Cryptobranchoidea are reconstructed to be metamorphosed and terrestrial as evidenced by the shape and non-shape variables of the palate. Disparities of ecological preference among metamorphosed taxa must have taken place before the Middle Jurassic (Bathonian), because one of the oldest known crown urodeles, Neimengtriton, is metamorphosed and semiaquatic as evidenced by the presence of a low but pliable dorsal caudal fin at adult stage. Most of the current scope in the phenotypic diversity of the palate possessed by modern cryptobranchoids were achieved by Early Cretaceous. Our results rigorously show that the shape of the palate and many non-shape covariates particularly associated with vomerine teeth are reliable ecological indicators for paleoecology of early salamanders, and we demonstrate that metamorphosis with biphasic ecological preference (aquatic larvae + terrestrial adults) is not only the ancestral lifestyle in salamanders but also significant for the rise and diversification of modern amphibians.
Materials and methods
Experimental design, specimens, and palate
Request a detailed protocolOur study includes 60 wet specimens (preserved in formalin) that represent 25 living species (29% of Hynobiidae and 75% of Cryptobranchidae) in all 12 living genera of Cryptobranchoidea (Andrias, Batrachuperus, Cryptobranchus, Hynobius, Liua, Onychodactylus, Pachyhynobius, Paradactylodon, Protohynobius, Pseudohynobius, Ranodon, and Salamandrella), and one fossil skeleton for each of five stem hynobiids (Liaoxitriton zhongjiani, Linglongtriton daxishanensis, Neimengtriton daohugouensis, Nuominerpeton aquilonare, and Regalerpeton weichangense), one basal pancryptobranchan (Aviturus exsecratus), two basal cryptobranchoids (Chunerpeton tianyiense and Pangerpeton sinense), one basal salamandroid (Beiyanerpeton jianpingense) and two stem urodeles (Karaurus sharovi and Kokartus honorarius; Dataset in Dryad). Sirenids were not included into this study for the following four reasons: (1) their palate is extremely specialized and is patterned with an enlarged palatine that is absent in most other salamanders and patches of teeth densely arranged on palatine and vomer; (2) sirenids are the only herbivory salamanders with a complex three-dimensional chewing behavior (Schwarz et al., 2020), and their specially configured palate may receive biomechanical patterns different from other salamanders; (3) the palate is incompletely preserved in the earliest known sirenid taxon Habrosaurus from the latest Early Cretaceous (Gardner, 2003); and (4) the existence of a ~90 Ma fossil gap between Habrosaurus and the earliest known salamandroids (Beiyanerpeton and Qinglongtriton) greatly impede our understanding of their early evolution of the palate. To keep the gender of species names consistent with that of genus names as per ICZN codes, we replaced the feminine/masculine species ending (‘-is’) by corresponding neuter forms (‘-e’) for genus names (e.g. Nuominerpeton) ending in the neuter noun ‘herpeton’ or ‘ἑρπετόν’ in Greek as suggested in Rong et al., 2021. In this study, each species is represented by one to three specimens, except for the only facultatively neotenic cryptobranchoid B. londongensis where both neotenic and metamorphosed populations are each represented by three specimens. Most of the specimens are accessioned in the following seven institutional collections: Capital Normal University (CNU), Beijing, China; Chengdu Institute of Biology (CIB), Chengdu, Sichuan Province, China; Field Museum of Natural History (FMNH), Chicago, USA; Liupanshui Normal University (HNUL), Liupanshui, Guizhou Province, China; Peking University of Paleontological Collections (PKUP), Beijing, China; Zhejiang Museum of Natural History (ZMNH), Hangzhou, Zhejiang Province, China; and Zunyi Medical University (ZMU), Zunyi, Guizhou Province, China. For specimens that we were not able to examine firsthand, we used publicly available micro-CT scan data of 12 living cryptobranchoid specimens (Dataset in Dryad) from the MorphoSource platform (MorphoSource.org) and published images for five fossil taxa, including Aviturus (Vasilyan and Böhme, 2012: Figure 3), Chunerpeton (Gao and Shubin, 2003: Figure 1), Karaurus (Ivachnenko, 1978: Figure 1), Kokartus (Skutschas and Martin, 2011: Figure 9), and Regalerpeton (Rong, 2018: Figure 5).
Besides the CT scan data obtained from MorphoSource, four fossil and all living specimens were micro-CT scanned using the following three high-resolution CT scanners: a Nikon XT H 320 LC scanner in the Industrial Micro-CT Laboratory at China University of Geosciences (Beijing); a GE Phoenix v/tome/x 240kv/180kv scanner in the PaleoCT Lab at The University of Chicago; and a Quantum GX micro-CT Imaging System (PerkinElmer, Waltham, USA) at CIB (Dataset in Dryad). No filter was used because beam hardening artifacts were not encountered during CT scanning. The voxel size of the volume files generated from CT scans ranges between 14.52–87.32 μm (see detailed parameters in Dataset in Dryad). File processing including segmentation and rendering were accomplished by VG Studio Max (version 2.2; Volume Graphics, Heidelberg, Germany), and images in jpeg format showing the ventral view of the skull were exported for landmark acquisition after digital removal of the mandible and the hyobranchium from the virtual models.
The palate in adult specimens of cryptobranchoids consists of the partes palatinae of the premaxilla and maxilla, paired vomers and a single median parasphenoid. An independently ossified palatine is present in few fossil taxa and is absent in the remaining, and is therefore not included in this study as a compromise between taxa sampling and landmarks collection. The pars palatina of both the premaxilla and maxilla is a narrow bony ledge and invariably contributes to a small portion of the palate by posteriorly articulating with the vomer, and hence is not considered in this study (Figure 1A). Both the vomer and parasphenoid are dorsoventrally flattened bony plates and are homologous across species studied here, and thus are ideal for landmark collections for both living and fossil specimens, considering that the palate in all available fossil cryptobranchoids is dorsoventrally preserved. All anatomical terms used here follow Trueb, 1993 unless otherwise stated.
Cladograms
Request a detailed protocolFor multivariate phylogenetic comparative analyses (evolutionary allometry analysis, phylogenetic signal analysis, Phylo-PCA, PA, phylogenetic alignment component analysis, and phylogenetic ANOVAs; see below), a maximum clade credibility tree (MCC; a target tree with maximum sum of posterior probabilities on its internal nodes) with 24 living taxa in our dataset was created by the software TreeAnnotator (version 1.10.4; Drummond et al., 2012) based on 10,000 random, time calibrated trees from a recent Bayesian posterior distribution analysis of living amphibians (Jetz and Pyron, 2018). To maximally match the terminal taxa in the MCC tree with our dataset, the living hynobiid Batrachuperus taibaiensis was eliminated from multivariate phylogenetic comparative analyses, considering that B. taibaiensis was recently synonymized to Batrachuperus tibetanus (Fei and Ye, 2016). The MCC tree was configured in Newick format and was read and visualized in the package ‘ape’ (version 5.5; Paradis and Schliep, 2019) for the software R (version 4.0.5; R Development Core Team, 2021). The remaining fossil taxa in our dataset with age ranges determined from published literature (Dataset in Dryad) and the Paleobiology Database (https://www.paleobiodb.org) were incorporated into this MCC tree based on a recent cladogram (Jia et al., 2021a: Figure 6) we constructed for living and fossil cryptobranchoids (Figure 1D). Adding fossil taxa increases the accuracy for multivariate phylogenetic comparative analyses and ancestral state reconstruction (see below; Soul and Wright, 2021), but will inevitably bring zero-length branches when the age of internal nodes was considered equal to that of its immediate oldest descendant, resulting in difficulties in multivariate phylogenetic comparative analyses. To circumvent this problem, any zero-length branch in the cladogram was treated to have a same branch length with its first none zero-length ancestral branch by using the ‘equal’ dating method in the ‘DatePhylo’ function from the R package ‘strap’ (version 1.4; Bell et al., 2015). We followed the suggestion from the ‘strap’ tutorial by setting the root length as 60 Ma by using the age difference between the oldest taxon in the cladogram (Kokartus; ~170 Ma) and the first older outgroup taxon known to date (Triassurus; ~230 Ma). Considering that the palate of Aviturus is only represented by the right vomer, the cladogram (Figure 1—figure supplement 24) including Aviturus was only used for the phylogenetic signal analysis and principal component analysis (PCA; see below) of the right vomer; whereas the cladogram without Aviturus was mapped onto the morphospace for a number of PCA and evolutionary rate analysis.
Data acquisition, geometric morphometrics, and symmetry and asymmetry
Request a detailed protocolThe geometry of the paired vomers and parasphenoid is represented on the ventral view of the palate by 24 type I (intersections of biological structures) and type II (maximum of curvature) 2D landmark points (Figure 1B; Bookstein, 1991; Slice et al., 1996) digitized by using tpsUtil (64 bit; version 1.76; Rohlf, 2015) and tpsDig2 (version 2.31; Rohlf, 2015) based on images of 70 specimens (the 24-raw-landmark dataset thereafter; see above; Dataset in Dryad). Most specimens have completely-preserved palate except that the Paleocene A. exsecratus is known only by a right vomer (Vasilyan and Böhme, 2012: Figure 3). In order to investigate the paleobiology of A. exsecratus, we created a separate dataset with seven corresponding landmarks (#1–#7) for the right vomer across all 71 specimens (termed as the seven-raw-landmark dataset thereafter; Dataset in Dryad). To reduce measurement errors, specimens were arranged alphabetically by the name of the image files, then landmarks in each specimen were digitized by the same author (J.J.) following the same order (landmark 1–24; Supplementary file 1K). Procrustes coordinates (24-Procrustes-landmark dataset and seven-Procrustes-landmark dataset) for the geometry of the palate were obtained in the software MorphoJ (version 1.07 a; Windows platform; Klingenberg, 2011) after superimposing the raw landmark coordinates of all specimens through the Generalized Procrustes Analysis (GPA; Bookstein, 1991; Figure 1—figure supplement 25), which serves to minimize non-shape variations (size, location, and orientation) among specimens by rescaling, repositioning and rotating raw landmark configurations. After superimposition, centroid size of the palate for each specimen is calculated as square root of the sum of squared distances of all Procrustes landmarks of the palate from their corresponding centroid. The mean shape of specimen/species are calculated as the consensus from the superimposed landmark coordinates. Procrustes variances/distance between specimens are calculated as the square root of the sum of the squared distances between corresponding landmarks (Klingenberg, 2016). The mean value for each species (the species mean thereafter) in the composite cladogram was obtained by averaging Procrustes coordinates and centroid sizes when there are more than one representative specimens.
The geometry of the palate shows object symmetry (Mardia et al., 2000) with landmarks #15 and #20 lying in the midsagittal axis of the skull and the remaining 11 pair landmarks being generally symmetric about the mid-sagittal plane (Figure 1A). To calculate respective contributions to the total amount of shape variation from symmetric and asymmetric shape components, we used the function ‘C1v’ in the software R to create a new double 24-raw-landmark dataset that contains all original raw landmark coordinates and their mirrored copy reflected about the midsagittal axis and relabeled to match the original landmark numbers (Savriama, 2018). This doubled 24-raw-landmark dataset was then imported into MorphoJ for superimposition, covariation matrix buildup and PCA. Considering that the symmetric and asymmetric components of shape variations occupy complementary subspaces in the shape tangent space defined by the doubled datasets for landmark configurations with object symmetry (Mardia et al., 2000; Klingenberg et al., 2002), the relative amount of contributions to shape changes from symmetric and asymmetric components equals to the sum of the eigenvalues of their corresponding PCs derived from the PCA. This procedure does not apply to the seven-landmark dataset because the right vomer has no object symmetry.
Allometric analysis
Request a detailed protocolTo verify if allometry, covariation of shape with size, plays a role in shape variations, we first performed a regular multivariate regression (Monteiro, 1999; Klingenberg, 2016) of the symmetric shape components (dependent variables) from the 24-Procrustes-landmark dataset on the log-transformed centroid size (log [CS]; independent variable) across all 70 specimens and the 34 species by using the ‘procD.lm’ function in the ‘geomorph’ R package (version 4.0.0; Adams et al., 2021; Collyer and Adams, 2021). Then we conducted a permutation test of 10,000 iterations using the same function to test the significance of these two regular regressions, respectively, considering that our sample size (n=70 for specimens; n=34 for species) is not considerably larger than the number of variables (n=49) for the 24-Procrustes-landmark dataset. Statistical results from these regular regression analyses may be inaccurate given that variations (or residuals) in the shape components among species are correlated with their evolutionary relationships (‘phylogenetic non-independence’ in Felsenstein, 1985). To account for impacts from the phylogeny, we conducted a phylogenetic regression (or evolutionary allometry analysis) of the shape components on log (CS) across species by using the function ‘procD.pgls’ of ‘geomorph,’ which is a distance-based phylogenetic generalized least squares method (Adams, 2014a) that uses our composite cladogram (without Aviturus) to remove phylogenetic covariances among species under a Brownian motion model of evolution.
To remove impacts from allometric shape components, shape variables (Procrustes-landmark coordinates in shape tangent space) were transformed by using residuals from the regular multivariate regression of shape on log (CS) across 70 specimens and 34 species, respectively, by using the function ‘procD.lm’ in ‘geomorph’ (Klingenberg, 2016). Non-allometric portion of the symmetric shape components are used for downstream statistical analyses.
Phylogenetic signal
Request a detailed protocolThe phylogenetic signal is termed based on a pattern that closely related species tend to have similar character values stemmed from their shared evolutionary history (Felsenstein, 1985; Blomberg et al., 2003; Adams, 2014b). Whether the shape of the palate bears any phylogenetic signal was tested by using the Kmult method in the function ‘physignal’ of ‘geomorph,’ which uses the composite cladogram to account for phylogenetic non-independence under a Brownian motion model of evolutionary divergence, and permutates shape components of terminal taxa against the composite cladogram (without Aviturus). A phylogenetic signal analysis was also conducted for the right vomer (non-allometric seven-Procrustes-landmark dataset) using the composite cladogram including Aviturus. The resulting Kmult value for each of the two analyses measures the phylogenetic signal as a ratio of observed to expected shape variation obtained with and without considering phylogenetic non-independence (Adams and Collyer, 2019). When the Kmult value is larger than and equals to 1, closely related taxa resemble each other phenotypically more than expected under Brownian model, whereas when the value is smaller than 1, closely related taxa are less similar to one another phenotypically than expected under Brownian model (Adams, 2014b).
Morphological disparity and ecological indication of the palate
Request a detailed protocolTaxonomically, the five groups considered here are Pancryptobrancha, Hynobiidae, stem hynobiids, basal cryptobranchoids and non-cryptobranchoid early salamanders. The Paleocene Aviturus was classified into the clade Pancryptobrancha (Gubin, 1991; Vasilyan and Böhme, 2012). The Late Jurassic Beiyanerpeton represents a basal member of Salamandroidea (Gao et al., 2013) and the two stem urodeles Karaurus and Kokartus were classified into the family Karauridae (Skutschas and Martin, 2011). Following Jia et al., 2021a, we apply the name Hynobiidae to the crown group of Panhynobia, and we consider crown and stem taxa of Panhynobia separately. As mentioned above, living hynobiids are mainly metamorphosed because individuals typically go through a short period of development during which larval features (e.g. external gills, gill slits) are resorbed (e.g. Kuzmin and Thiesmeier, 2001; Kami, 2004; Fei et al., 2006; Poyarkov et al., 2012), and B. londongensis represents the single facultatively neotenic hynobiid species; living cryptobranchids are generally referred to as neotenic or partially metamorphosed as their individuals live in water in the adult stage and retain a few paedomorphic features (Deban and Wake, 2000), except that the life history strategy of Aviturus remains unknown (Vasilyan and Böhme, 2012; but see Skutschas et al., 2018). It is noteworthy that many fossil taxa investigated here (e.g. Chunerpeton) bear more apparent neotenic features (e.g. external gills, gill rakers) than living cryptobranchids; however, we did not differentiate subgroups within neotenic taxa due to our sampling scope. Living habitats of cryptobranchoids in the adult stage outside the breeding season have been broadly classified into three types, namely aquatic, semiaquatic, and terrestrial (Fei et al., 2006; Rong, 2018; Fei and Ye, 2016; AmphibiaWeb, 2021). In order to understand if factors like taxonomic affiliations at the genus and family level, life history strategy and living habitat would contribute to morphological disparities of the palate, we conducted phylogenetic Procrustes ANOVAs for the non-allometric symmetric shape components of the 24-Procrustes-landmark dataset using the function ‘procD.pgls’ of ‘geomorph.’ Pair-wise comparisons for morphological disparity (Procrustes variance) for the palate, vomer, parasphenoid (Supplementary file 1B) and each landmark point (Supplementary file 1C–E) across each of the aforementioned category were performed using ‘morphol.disparity’ in ‘geomorph.’
Principal component analyses
Request a detailed protocolTo visualize the patterns of shape changes in the morphospace of palate and to investigate the mechanisms framing up the disparity patterns, we conducted four types of PCA by using the function ‘gm.prcomp’ in ‘geomorph’: a standard PCA, a phylogenetically aligned component analysis (PaCA; Collyer et al., 2020), a phylomorphospace analysis (PA; Rohlf, 2002), and a phylogenetic principal component analysis (Phylo-PCA; Revell, 2009). The standard PCA is based on a covariance matrix of the non-allometric symmetric shape components across all 70 specimens, and the resulting first few PCs (eigenvalues) reveal predominant trends in shape disparity patterns.
The other three PCA are based on the composite cladogram (without Aviturus) and a covariance matrix of the non-allometric symmetric shape components across all 34 species, with the ancestral PC values (eigenvalue) for internal nodes (Figure 1—figure supplement 26) estimated by using the Brownian motion model of evolution. The resulting first few PCs derived from the PA reveal predominant trends in shape disparity patterns with the phylogeny superimposed in the morphospace. On the other hand, the first PC derived from PaCA reveal shape disparity patterns mostly associated with phylogenetic signal, and the first few PCs from Phylo-PCA are phylogenetic independent and therefore reveal factors (e.g. ecological preferences) other than phylogenetic signal that account for disparity patterns of the palate (see Collyer et al., 2020). A same analysis strategy was conducted for the non-allometric seven-Procrustes-landmark dataset to address the paleobiology of Aviturus (Figure 2—figure supplement 5). Visualizations of the results were achieved by using the functions ‘plot.gm.prcomp,’ ‘make_ggplot’ in ‘geomorph,’ and ‘ggplot’ in the package ‘ggplot2’ (version 3.3.5; Wickham, 2016).
Covariates of the palate
Request a detailed protocolConfigurations of VTR have long been identified as informative in the classification of Cryptobranchoidea (Estes, 1981; Zhao and Hu, 1984; Fei et al., 2006) and were recently argued to be ecologically informative (Jia et al., 2021b). To test if non-shape variations of the palate are reliable indicators in reconstructing paleoecology of early salamanders, we chose the following five continuous and two categorical covariates: length ratio of the vomer in the palate; length ratio of the parasphenoid in the palate; vomerine tooth number on a single vomer; width ratio of VTR in the vomer; width ratio of the outer and inner branch of VTR; and both the position (relative to the vomerine plate: anterior, middle, mid-posterior or posterior) and arrangement (oblique, transverse, curved) of VTR. Measurements of these continuous and categorical features were collected by using VG Studio Max and the Fiji platform (version 1.8.0_172; Schneider et al., 2012), with missing values for Aviturus, Kokartus, and Pangerpeton reconstructed by using the function ‘phylopars’ in ‘Rphylopars’ R package (version 0.3.2; Goolsby et al., 2021). Values and states for the five continuous covariates were visualized by boxplot in ‘ggplot2’ and were compared among different ecological groups by using Mann-Whitney U-test (Figure 3). Association of the two categorical covariates with ecology were investigated by contingency table and Cochran-Mantel-Haenszel test (Figure 3—figure supplement 1 and Supplementary file 1I).
Ancestral state reconstruction and evolutionary rate
Request a detailed protocolThe ancestral shapes of the palate for several internal nodes including the respective last common ancestors of Hynobiidae, Panhynobia, Pancryptobrancha, Cryptobranchoidea, Urodela, and Caudata were estimated by using maximum likelihood method in the ‘anc.recon’ function in the ‘Rphylopars’ R package (version 0.3.2; Goolsby et al., 2021). The ancestral value of the five continuous covariates for the internal nodes was estimated by using ‘phylopars’ in the same package (Supplementary file 1J). On the other hand, the ancestral states of the two categorical covariates and life history strategy were estimated using the function ‘ace’ in ‘ape’, with their likelihood for each of the internal node depicted as a pie chart (Figure 3). Wire plots for the ancestral shape of the internal nodes with reference to the mean shape were created by using the functions ‘mshape’ and ‘plotRefToTarget’ in ‘geomorph’.
Evolutionary rates for each of the 24 landmarks collected on the palate, the vomer, the parasphenoid, and the five continuous covariates from the palate were calculated by using the function ‘compare.multi.evol.rates’ in ‘geomorph’. Then evolutionary rates of the palate, the vomer and the parasphenoid among species across groups in ecology, life history strategy and taxonomic affiliation were calculated and compared by using the function ‘compare.evol.rates’ in ‘geomorph’ (Supplementary file 1H).
Results derived from this study were exported from R and were illustrated and assembled in Adobe Photoshop CC. Source codes for R used in this study is available at GitHub (https://github.com/SalamanderGeomorph/Salamander_Palate, copy archived at swh:1:rev:8cbdd82025b0bf987bb6211239a2e7dc56c615d5, Salaman, 2022).
Data availability
All data needed to evaluate the conclusions are included in the manuscript and the Supplementary file 1. Details of specimens, CT parameters and raw landmark coordinates and centroid sizes are available in three CSV files in the online Dryad repository (https://doi.org/10.5061/dryad.c59zw3r8x). Source codes for R and SAS used in this study are available at GitHub (https://github.com/SalamanderGeomorph/Salamander_Palate, copy archived at swh:1:rev:8cbdd82025b0bf987bb6211239a2e7dc56c615d5).
-
Dryad Digital RepositorySpecimen list and landmark coordinates for the palate of early salamanders.https://doi.org/10.5061/dryad.c59zw3r8x
References
-
A method for assessing phylogenetic least squares models for shape and other high-dimensional multivariate dataEvolution; International Journal of Organic Evolution 68:2675–2688.https://doi.org/10.1111/evo.12463
-
Phylogenetic Comparative Methods and the Evolution of Multivariate PhenotypesAnnual Review of Ecology, Evolution, and Systematics 50:405–425.https://doi.org/10.1146/annurev-ecolsys-110218-024555
-
Testing for phylogenetic signal in comparative data: behavioral traits are more labileEvolution; International Journal of Organic Evolution 57:717–745.https://doi.org/10.1111/j.0014-3820.2003.tb00285.x
-
BookMorphometrics Tools for Landmark DataCambridge University Press.https://doi.org/10.1017/CBO9780511573064
-
Phylogenetically aligned component analysisMethods in Ecology and Evolution 12:359–372.https://doi.org/10.1111/2041-210X.13515
-
SoftwareRRPP: linear model evaluation with randomized residuals in a permutation proceduresR Package Version 0.6.2.
-
BookAquatic feeding in salamandersIn: Schwenk K, editors. Feeding: Form, Function and Evolution in Tetrapod Vertebrates. Academic. pp. 65–94.
-
Two Jurassic salamanders with stomach contents from Inner Mongolia, ChinaChinese Science Bulletin 57:72–76.https://doi.org/10.1007/s11434-011-4729-z
-
Bayesian phylogenetics with BEAUti and the BEAST 1.7Molecular Biology and Evolution 29:1969–1973.https://doi.org/10.1093/molbev/mss075
-
The Sound-Transmitting Apparatus of Salamanders and the Phylogeny of the CaudataThe American Naturalist 56:418–427.https://doi.org/10.1086/279882
-
BookEncyclopedia of Paleoherpetology. Part 2, Gymnophiona, CaudataGustav Fischer Verlag.
-
BookMiddle Jurassic microvertebrate assemblages from the British IslesIn: Fraser NC, Sues HD, editors. In the Shadow of the Dinosaurs: Early Mesozoic Tetrapods. Cambridge University Press. pp. 303–321.
-
Metamorphosis shapes cranial diversity and rate of evolution in salamandersNature Ecology & Evolution 4:1129–1140.https://doi.org/10.1038/s41559-020-1225-3
-
Phylogenies and the Comparative MethodThe American Naturalist 125:1–15.https://doi.org/10.1086/284325
-
Taxonomic diversity, stratigraphic range, and exceptional preservation of Juro-Cretaceous salamanders from northern ChinaCanadian Journal of Earth Sciences 50:255–267.https://doi.org/10.1139/e2012-039
-
Aquatic prey transport and the comparative kinematics of ambystoma tigrinum feeding behaviorsThe Journal of Experimental Biology 187:159–179.https://doi.org/10.1242/jeb.187.1.159
-
Urodelans from the Triassic and Jurassic of Soviet Central AsiaPaleontologicheskii Zhurnal 12:362–368.
-
A new stem hynobiid salamander (Urodela, Cryptobranchoidea) from the Upper Jurassic (Oxfordian) of Liaoning Province, ChinaJournal of Vertebrate Paleontology 39:e1588285.https://doi.org/10.1080/02724634.2019.1588285
-
The biology of the Persian Mountain Salamander, Batrachuperus persicus (Amphibia, Caudata, Hynobiidae) in Golestan Province, IranAsiatic Herpetological Research 10:182–190.
-
ConferenceNew Late Triassic stem-caecilian form southwestern North America strengthens evidence for lissamphibian monophyly, and illuminates the anatomical, functional, and geographic origins of living caeciliansThe Society of Vertebrate Paleontology (ed.): virtual meeting conference program, 81st Annual Meeting.
-
Shape analysis of symmetric structures: quantifying variation among individuals and asymmetryEvolution; International Journal of Organic Evolution 56:1909–1920.https://doi.org/10.1111/j.0014-3820.2002.tb00117.x
-
MorphoJ: an integrated software package for geometric morphometricsMolecular Ecology Resources 11:353–357.https://doi.org/10.1111/j.1755-0998.2010.02924.x
-
Size, shape, and form: concepts of allometry in geometric morphometricsDevelopment Genes and Evolution 226:113–137.https://doi.org/10.1007/s00427-016-0539-2
-
What do ossification sequences tell us about the origin of extant amphibians?Peer Community Journal 2:212.https://doi.org/10.24072/pcjournal.89
-
Evolution of the Amphibian Ear with Implications for Lissamphibian Phylogeny: Insight Gained from the Caecilian Inner EarFieldiana Life and Earth Sciences 5:59–76.https://doi.org/10.3158/2158-5520-5.1.59
-
ape 5.0: an environment for modern phylogenetics and evolutionary analyses in RBioinformatics (Oxford, England) 35:526–528.https://doi.org/10.1093/bioinformatics/bty633
-
SoftwareR: A language and environment for statistical computingR Foundation for Statistical Computing, Vienna, Austria.
-
FEEDING SPECIALIZATIONS AND THE CLASSIFICATION OF TERRESTRIAL SALAMANDERSEvolution; International Journal of Organic Evolution 20:392–407.https://doi.org/10.1111/j.1558-5646.1966.tb03374.x
-
THE EVOLUTION OF TETRAPOD FEEDING BEHAVIOR: KINEMATIC HOMOLOGIES IN PREY TRANSPORTEvolution; International Journal of Organic Evolution 44:1542–1557.https://doi.org/10.1111/j.1558-5646.1990.tb03845.x
-
Size-correction and principal components for interspecific comparative studiesEvolution; International Journal of Organic Evolution 63:3258–3268.https://doi.org/10.1111/j.1558-5646.2009.00804.x
-
BookGeometric morphometrics and phylogenyIn: MacLeod N, editors. Morphology, Shape and Phylogeny. Taylor & Francis. pp. 175–193.
-
SoftwareSalamander Palate, version swh:1:rev:8cbdd82025b0bf987bb6211239a2e7dc56c615d5Software Heritage.
-
A Step-by-Step Guide for Geometric Morphometrics of Floral SymmetryFrontiers in Plant Science 9:1433.https://doi.org/10.3389/fpls.2018.01433
-
NIH Image to ImageJ: 25 years of image analysisNature Methods 9:671–675.https://doi.org/10.1038/nmeth.2089
-
Metamorphosis and neoteny: alternative pathways in an extinct amphibian cladeEvolution; International Journal of Organic Evolution 60:1467–1475.https://doi.org/10.1111/j.0014-3820.2006.tb01225.x
-
BookTemnospondyli I. Part 3A2 of Sues H-D (ed.): Handbook of PaleoherpetologyMünchen: Dr. Friedrich Pfeil.
-
A salamander that chews using complex, three-dimensional mandible movementsThe Journal of Experimental Biology 223:jeb220749.https://doi.org/10.1242/jeb.220749
-
A new genus and species of basal salamanders from the Middle Jurassic of Western Siberia, RussiaProceedings of the Zoological Institute RAS 315:167–175.
-
Cranial anatomy of the stem salamander Kokartus honorarius (Amphibia: Caudata) from the Middle Jurassic of KyrgyzstanZoological Journal of the Linnean Society 161:816–838.https://doi.org/10.1111/j.1096-3642.2010.00663.x
-
Mesozoic salamanders and albanerpetontids of Middle Asia, Kazakhstan, and SiberiaPalaeobiodiversity and Palaeoenvironments 93:441–457.https://doi.org/10.1007/s12549-013-0126-8
-
A new crown-group salamander from the Middle Jurassic of Western Siberia, RussiaPalaeobiodiversity and Palaeoenvironments 96:41–48.https://doi.org/10.1007/s12549-015-0216-x
-
Appendix I: a glossary for geometric morphometricsNato Advanced Science Institutes Series A: Life Sciences 284:531–551.
-
BookPhylogenetic Comparative Methods: A User’s Guide for PaleontologistsCambridge University Press.https://doi.org/10.1017/9781108894142
-
BookPatterns of cranial diversity among the LissamphibiaIn: Hanken J, Hall BK, editors. The Skull, Volume 2: Patterns of Structural and Systematic Diversity. The University of Chicago Press. pp. 255–343.
-
A new giant salamander (Urodela, Pancryptobrancha) from the Miocene of Eastern Europe (Grytsiv, UkraineJournal of Vertebrate Paleontology 33:301–318.https://doi.org/10.1080/02724634.2013.722151
-
BookTerrestrial feeding in salamandersIn: Schwenk K, editors. Feeding: Form, Function and Evolution in Tetrapod Vertebrates. Academic, San Diego. pp. 95–116.
-
What Salamanders Have Taught Us About EvolutionAnnual Review of Ecology, Evolution, and Systematics 40:333–352.https://doi.org/10.1146/annurev.ecolsys.39.110707.173552
-
Jeholotriton paradoxus (Amphibia: Caudata) from the Lower Cretaceous of southeastern Inner Mongolia, ChinaJournal of Vertebrate Paleontology 25:523–532.https://doi.org/10.1671/0272-4634(2005)025[0523:JPACFT]2.0.CO;2
-
High-resolution taphonomic and palaeoecological analyses of the Jurassic Yanliao Biota of the Daohugou area, northeastern ChinaPalaeogeography, Palaeoclimatology, Palaeoecology 530:200–216.https://doi.org/10.1016/j.palaeo.2019.05.028
-
BookGgplot2: Elegant Graphics for Data AnalysisCham: Springer-Verlag.https://doi.org/10.1007/978-3-319-24277-4
-
Comparison of skull morphology in two species of genus Liua (Amphibia: Urodela: HynobiidaeAsian Herpetological Research 7:112–121.https://doi.org/10.16373/j.cnki.ahr.150055
-
BookStudies on Chinese Tailed AmphibiansSichuan Scientific and Technical Publishing House.
Article and author information
Author details
Funding
National Natural Science Foundation of China (41702002)
- Jia Jia
National Natural Science Foundation of China (41872008)
- Ke-Qin Gao
State Key Laboratory of Palaeobiology and Stratigraphy (193111)
- Jia Jia
The funders had no role in study design, data collection and interpretation, or the decision to submit the work for publication.
Acknowledgements
We would like to thank C-F Zhou (Shandong University of Science and Technology) for help with field work and stratigraphic data collection; J Anderson (University of Calgary) and J Gardner (Royal Tyrrell Museum) for insightful comments and helpful discussions; J-P Jiang (Chengdu Institute of Biology, [CIB]), A Resetar (Field Museum of Natural History, [FMNH]), C-S Chen (Zhejiang Museum of Natural History), R-C Xiong (Liupanshui Normal University), G Wei (Zunyi Medical University) and S Wang (Capital Normal University) for access to comparative specimens of living salamanders under their curation. We are grateful to Z-X Luo, J Lemberg, A I Neander (all University of Chicago) and M-H Zhang (CIB) for their assistance in CT scanning specimens. We greatly appreciate eLife to generiously waive the publication fee for us, the Senior Editor George Perry, Reviewing Editor and Reviewer Min Zhu and two other Reviewers Pavel Skutschas and David Marjanović for their valuable suggestions. JJ and GL are grateful to J-H JiaLi for her encouragement during the pandemic. JJ is supported by National Natural Science Foundation of China (41702002) and State Key Laboratory of Palaeobiology and Stratigraphy (Nanjing Institute of Geology and Palaeontology, CAS) (193111). K-QG is supported by National Natural Science Foundation of China (41872008).
Copyright
© 2022, Jia et al.
This article is distributed under the terms of the Creative Commons Attribution License, which permits unrestricted use and redistribution provided that the original author and source are credited.
Metrics
-
- 1,529
- views
-
- 220
- downloads
-
- 4
- citations
Views, downloads and citations are aggregated across all versions of this paper published by eLife.
Citations by DOI
-
- 4
- citations for umbrella DOI https://doi.org/10.7554/eLife.76864