Contractile force assessment methods for in vitro skeletal muscle tissues
Abstract
Over the last few years, there has been growing interest in measuring the contractile force (CF) of engineered muscle tissues to evaluate their functionality. However, there are still no standards available for selecting the most suitable experimental platform, measuring system, culture protocol, or stimulation patterns. Consequently, the high variability of published data hinders any comparison between different studies. We have identified that cantilever deflection, post deflection, and force transducers are the most commonly used configurations for CF assessment in 2D and 3D models. Additionally, we have discussed the most relevant emerging technologies that would greatly complement CF evaluation with intracellular and localized analysis. This review provides a comprehensive analysis of the most significant advances in CF evaluation and its critical parameters. In order to compare contractile performance across experimental platforms, we have used the specific force (sF, kN/m2), CF normalized to the calculated cross-sectional area (CSA). However, this parameter presents a high variability throughout the different studies, which indicates the need to identify additional parameters and complementary analysis suitable for proper comparison. We propose that future contractility studies in skeletal muscle constructs report detailed information about construct size, contractile area, maturity level, sarcomere length, and, ideally, the tetanus-to-twitch ratio. These studies will hopefully shed light on the relative impact of these variables on muscle force performance of engineered muscle constructs. Prospective advances in muscle tissue engineering, particularly in muscle disease models, will require a joint effort to develop standardized methodologies for assessing CF of engineered muscle tissues.
Introduction
The main function of skeletal muscle is to produce contractile force (CF) (Vandenburgh et al., 2008; Shadrin et al., 2016), which is necessary for locomotion, respiration, and metabolic processes (Kaji et al., 2010; Qazi et al., 2015; Wang et al., 2019; Yusuf and Brand-Saberi, 2012). CF may be compromised during aging or due to major injuries or genetic mutations, such as in muscular dystrophies. Calcium (Ca2+) homeostasis, which is usually dysregulated in these conditions, has a profound impact on force production (Vallejo-Illarramendi et al., 2014; Al Tanoury et al., 2021). Muscular contraction is initiated by motoneurons, which release acetylcholine (ACh) upon activation by action potentials. ACh causes sarcolemmal depolarization and opening of calcium channels at the sarcolemma and the sarcoplasmic reticulum. Ca2+ diffuses throughout the sarcomere and initiates contraction by a cross-bridge cycle between actin and myosin (Widmaier and Raff, 2008; Fernández-Costa et al., 2021; Santoso et al., 2021).
For years, standard 2D cellular cultures and animal models have been used to understand muscle physiopathology and to develop novel treatments for muscle diseases. Despite the many discoveries regarding those models, a comparison with native human muscle suggests that major challenges remain in order to obtain a fully representative or trustworthy model (Horvath et al., 2016; Santoso et al., 2021; Wang et al., 2019). Characteristics such as size, drug diffusion, or immune response are difficult to match with the ones occurring in the human body (Fernández-Costa et al., 2021; Khodabukus, 2021). For instance, the muscular dystrophy X-linked mouse (mdx) is a well-accepted preclinical model for Duchenne Muscular Dystrophy (DMD) (Bersini et al., 2018; Lasa-Fernandez et al., 2020; Al Tanoury et al., 2021). However, mdx mice present substantial differences compared to Duchenne patients regarding muscle regeneration, Ca2+ handling, and life expectancy (Raymackers et al., 2003; Toral-Ojeda et al., 2018; Yoshioka et al., 2021b). Other DMD animal models (dogs or pigs) seem to better recapitulate the human disease, but they entail substantial drawbacks or challenges such as higher variability in disease progression, longer experimental times, and, obviously, much higher costs (Smith et al., 2016; Kornegay, 2017).
In vitro models, as an alternative to animal models, are already providing promising results in different applications such as disease modeling (Shimizu et al., 2017; Nesmith et al., 2016; Badu-Mensah et al., 2020; Fernández-Garibay et al., 2021), drug discovery (Vandenburgh, 2010; Ikeda et al., 2017; Afshar et al., 2020; Alave Reyes-Furrer et al., 2021), gene therapy (Powell et al., 1999; Vandenburgh et al., 1996), reconstructive surgery (Brady et al., 2008; Kim et al., 2018; Gilbert-Honick et al., 2020), or even as bio-actuators in biohybrid systems (Yamamoto et al., 2011; Cvetkovic et al., 2014; Gao et al., 2021). Indeed, the latest technological advances in tissue engineering such as 3D bioprinting or the development of novel biomaterials and culture platforms (Capel et al., 2019; Denes et al., 2019; Costantini et al., 2021; Gupta et al., 2021; Peper et al., 2021) are facilitating the development of better in vitro skeletal muscle constructs. To this end, researchers have recapitulated many native skeletal muscle features, including contraction (Madden et al., 2015; Urciuolo et al., 2020; Akiyama et al., 2021; Alave Reyes-Furrer et al., 2021), vascularization (van der Schaft et al., 2011; Bersini et al., 2018; Osaki et al., 2018a; Gilbert-Honick et al., 2018), mechanical tension (Okano and Matsuda, 1998; Vandenburgh and Karlisch, 1989), neuromuscular junction (NMJ) (Afshar Bakooshli et al., 2019; Yoshioka et al., 2020; Vila et al., 2021), and muscle stiffness (Urciuolo et al., 2020). These features, represented in Figure 1 (top), are considered key requirements for generating functional and mature skeletal muscle constructs.
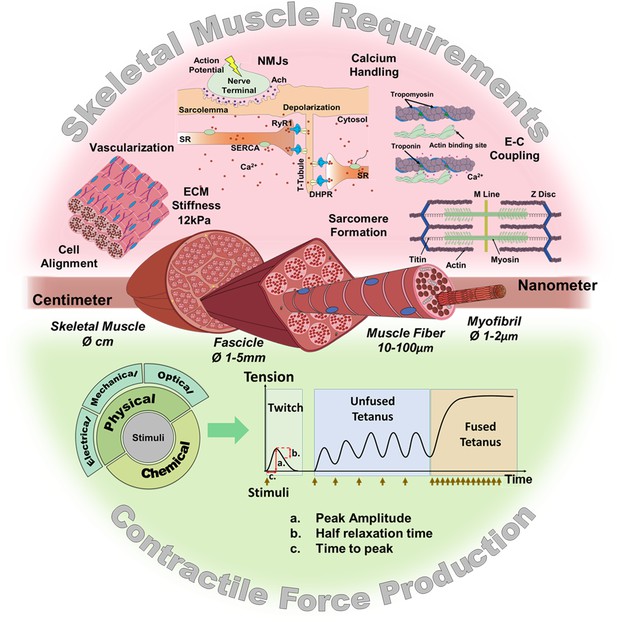
Skeletal muscle structure and requirements for contractile force production.
(Top) Summary of the main requirements to enable contractility in skeletal muscle in vitro models. (Middle) Representation of the muscular hierarchy and (bottom) summary of contractile stimuli and contraction profiles.
The current gold standard for the characterization of in vitro muscle models includes expression analysis of myogenic markers, determination of the fusion index, and evaluation of striation pattern and ACh receptor aggregation (Bajaj et al., 2011; Toral-Ojeda et al., 2016; Toral-Ojeda et al., 2018). Whereas this information is helpful for understanding the basic differentiation status of the culture, CF provides further physiological information about the degree of tissue maturation and functionality (Madden et al., 2015; Ikeda et al., 2016; Juhas et al., 2016; Nagashima et al., 2020). Indeed, CF evaluation has already established its usefulness as a primary outcome measure in drug screening platforms (Ikeda et al., 2017; Shimizu et al., 2017; Vandenburgh et al., 2009), analyzing the performance of different cellular sources (Nagashima et al., 2020; Shimizu et al., 2020), assessing the effect of scaffold composition (Boontheekul et al., 2007; Hinds et al., 2011), or even evaluating the influence of heat stress (Takagi et al., 2018), and media supplements on muscle development (Martin et al., 2017; Mills et al., 2019; Xu et al., 2019).
However, it is quite challenging to compare CF magnitudes obtained under different experimental conditions in which crucial key factors differ such as culture platforms (Anene-Nzelu et al., 2013; Khodabukus and Baar, 2016), culturing methods, cell lines, scaffold materials (Zhuang et al., 2020; Gilbert-Honick et al., 2020; Perez-Puyana et al., 2021) or even construct size. Consequently, there is a wide range of results from different research laboratories worldwide that are difficult to compare or interpret. This fact raises the need to define homogeneous units and to standardize the methodology for CF assessment in order to facilitate proper comparison among studies.
Contractile force induction
Several stimulation mechanisms may be used to trigger contraction of in vitro muscle constructs at specific paces: CF may be induced by chemical (Osaki et al., 2018b; Afshar et al., 2020; Yoshioka et al., 2020; Hofemeier et al., 2021), or physical stimuli (Figure 1, bottom). As for physical stimuli, they comprise electrical (Juhas and Bursac, 2014; Madden et al., 2015; Santoso et al., 2021), mechanical (Kim et al., 2019), and optical stimulation (Neal et al., 2015; Mills et al., 2019; Uchimura et al., 2021; Hofemeier et al., 2021).
Electrical stimulation is the most frequently used mechanism to induce CF in engineered muscles by mimicking motoneuron activity on these tissues (Cheng et al., 2014b; Rangarajan et al., 2014; Ikeda et al., 2016; Akiyama et al., 2021). It has also been used during muscle development to improve muscle size, sarcomere formation, Ca2+ transients, and CF production (Park et al., 2008; Langelaan et al., 2011; Khodabukus et al., 2019). Electrical stimulation causes depolarization of the muscle cell membrane, which triggers excitation-contraction coupling (Merrill et al., 2005; Uchimura et al., 2021). It is essential to optimize the electrical parameters for each experimental setup and culture stage, since inadequate stimulation can result in culture damage or untoward responses, including fatigue, electrochemical damage, or electroporation effect, leading to increased membrane permeability and impaired cell function (Nikolić et al., 2017; Khodabukus and Baar, 2012; Mills et al., 2019; Ruzgys et al., 2019; Nagamine et al., 2011; Khodabukus, 2021).
Optical stimulation or photo-stimulation entails the incorporation of light-sensitive proteins such as Channelrhodopsin-2 (ChR2) by gene delivery. ChR2 is a blue-light-sensitive opsin that triggers membrane depolarization and downstream signaling cascades (Mahmoudi et al., 2017). This approach allows for an accurate spatial and temporal control of the contractile activity in a non-invasive manner, with minimal side effects to the constructs (Bruegmann et al., 2010; Asano et al., 2012; Neal et al., 2015; Vila et al., 2021). The performance of this technique depends on efficient gene delivery into the cells (Zhang et al., 2006). In particular, gene transduction with lentiviral particles has been shown to effectively generate mature 2D and 3D muscle constructs with the capability to trigger contractions optogenetically (Osaki et al., 2018b; Hofemeier et al., 2021; Cheesbrough et al., 2022).
Mechanical stimulation aims to improve muscular development by evoking the stimuli given by the skeletal system during human embryogenesis (Powell et al., 2002; Moon et al., 2008; Candiani et al., 2010). Its mechanism is based on the generation of tensile stress on the tissue construct, which triggers cellular responses mediated by mechanotransduction pathways. Depending on the parameters used, mechanical stimulation may lead to tissue enhancement and rescue of atrophy, with increased myotube number, myofiber diameter, and fusion index (Vandenburgh et al., 1991; Aguilar-Agon et al., 2019; Kim et al., 2019). However, inadequate mechanical stimulation may also induce pathological changes in muscle constructs, such as poor tissue maturation as revealed by decreased sarcomeric proteins (Akimoto et al., 2001; Boonen et al., 2010).
Finally, chemical stimulation may induce contractility of the muscle construct by direct exposure to certain biochemical compounds such as KCl, caffeine, glutamic acid, and acetylcholine (Osaki et al., 2018b; Yoshioka et al., 2020; Hofemeier et al., 2021). In addition to contraction, chemical stimulation is commonly used to induce other cellular responses, including changes in Ca2+ fluxes and cell size (Madden et al., 2015; Shimizu et al., 2017).
Contractile force analysis
Specific force
CF or tension, which is expressed in Newtons (N) is not a very informative parameter for engineered muscles, because it does not relate to the size and condition of the tissue construct. Instead, the Specific Force (sF), also referred to as mechanical stress, is the CF normalized to the cross-sectional area (CSA) of the construct, and it is expressed in Pascals (Pa = N/m2) (Bottinelli and Reggiani, 2000; Maganaris et al., 2001; Pirozzi et al., 2013; Miller et al., 2015). CF is proportional to the number of muscle fibers and thus to the cross-sectional area of the construct (Pirozzi et al., 2013). Hence, CF can be easily converted into sF following Equation 1 below.
A major drawback concerning this parameter is the wide diversity of criteria in the literature for defining and quantifying CSA of muscle constructs (Figure 2). In 2D models, CSA can be estimated as a circular shape, or calculated as an elliptical shape from the thickness and the width of the myotubes (Pirozzi et al., 2013; Jeon et al., 2019). As for 3D constructs, the CSA has been approximated to circular (Hinds et al., 2011; Juhas and Bursac, 2014; Nagashima et al., 2020), rectangular (Donnelly et al., 2010), or elliptical (Akiyama et al., 2021) shapes. Moreover, CSA may also be determined from immunohistochemical sections. In this case, most works consider the whole area of the engineered tissue (total CSA). However, non-contractile areas can account for up to 90% of the whole CSA (Sakar et al., 2012; Juhas and Bursac, 2014; Ebrahimi et al., 2021), which has a substantial impact on sF calculations. Thus, other studies consider only the area occupied by the myotubes, which is referred to as effective-CSA (Hinds et al., 2011; Sakar et al., 2012; Sato et al., 2013; Ebrahimi et al., 2021). Other authors normalize CF by cell number (mN/cell) or cell density (mN/cell*mm–2), of the muscle construct (Xu et al., 2019).
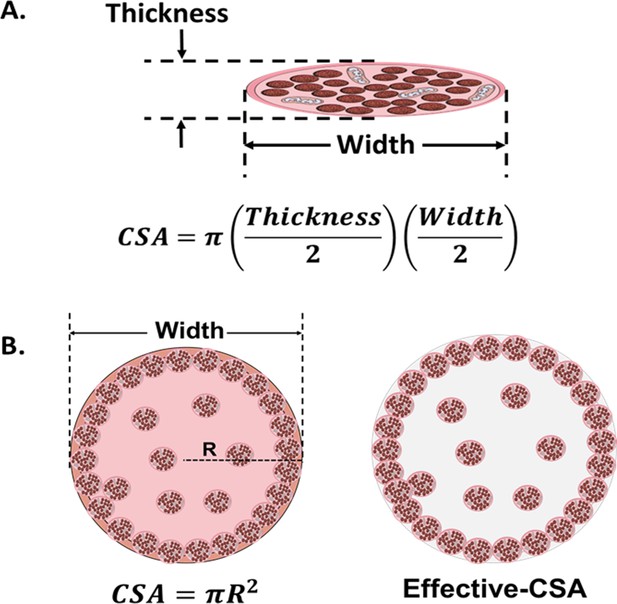
Cross-sectional area (CSA) in 2D and 3D muscle models.
(A) Myotube CSA estimated as an elliptical shape from the thickness and the width of the cell. (B) CSA of 3D muscle constructs can be estimated by approximation to different shapes (circle, in left panel), or calculated from immunohistochemical sections. Effective-CSA is known as the area occupied by myotubes (red area in the right panel).
Studies summarized in Table 1 and Source Data 1 indicate a preference for normalizing CF to the whole CSA (calculated as a circular shape). However, as shown above, the use of sF to compare results across laboratories may turn out to be unreliable due to the large differences in the methodology used to calculate CSA. In addition, tissue processing required for immunohistochemical techniques may entail size artifacts that further affect CSA calculations. Along these lines, a previous study in human isolated fibers claims that diameter measurements present less variability than CSA measurements resulting in a more accurate normalization in N/m instead of sF (Krivickas et al., 2011). We believe that normalizing CF to diameter or to a related parameter (i.e. calculated circular CSA) would allow for a more reliable comparison among different studies, as long as similar contractile areas are verified. In any case, in order to compare sF, it is essential to thoroughly evaluate the method used in each study for CF normalization.
Maximum contractile force data from in vitro muscle models measured by the three main platforms.
Cell source | Evaluation time | Size | CSA(mm2) | Twitch contraction | Tetanic contraction | Tetanic-Twitch Ratio* | References | |||
---|---|---|---|---|---|---|---|---|---|---|
CF (µN) | sF (kN/m²) | CF (µN) | sF (kN/m²) | |||||||
Cantilever deflection | C2C12 myoblasts (mouse) | Day 7 | 50 μm (Width)i 33 µm (Thickness)* | 0.001308* | 0.54 ± 0.02 | 0.41* | 1.01 ± 0.14 | 0.77* | 1.87 | Fujita et al., 2010 |
Rat myoblasts (embryonic) | Day 10–13 | 22.5 µm (Width)i 10 µm (Thickness) | 0.000176* | 0.23* | 1.3 | __ | __ | __ | Wilson et al., 2010 | |
C2C12 myoblasts (mouse) | Day 6 | 12.75 µm (Width)* 8–9 µm (Thickness) | 0.0000851* | 0.80* | 9.4 ± 4.6 | __ | __ | __ | Sun et al., 2013 | |
Rat myoblasts (embryonic) | Day 12–14 | 11.7–23.4 μm (Width) 7.9–13 μm (Thickness) | 0.000144* | 0.04–0.26 Stoney’s Eq. 0.03–0.18 FEA | 0.359–1.70 Stoney’s Eq. 0.168–1.17 FEA | __ | __ | __ | Pirozzi et al., 2013 | |
Primary human myoblast | Day 23 | 10 µm (Width)i 6.67 µm (Thickness)* | 0.000052* | 0.14g | 2.69* | __ | __ | __ | Smith et al., 2014 | |
Rat myoblasts (adult) | Day 4–7 | 16.74 µm (Width)* 11.16 µm (Thickness)g | 0.000146* | 0.17g | 1.15* | __ | __ | __ | McAleer et al., 2014 | |
Human myoblasts | Day 3–6 | 12.11 μm (Width)g 8.07 µm (Thickness)* | 0.0000767* | 0.78* | 9.98g | __ | __ | __ | Nesmith et al., 2016# | |
Human induced pluripotent stem cell | Day 14 | 11.82 μm (Width)g 10.35 μm (Thickness)g | 0.0000961* | 0.38* | 3.98g | __ | __ | __ | Badu-Mensah et al., 2020 | |
Human induced pluripotent stem cell | Day 10–11 | 9.30 μm (Width)g 6.2 μm (Thickness)* | 0.0000452* | 0.12 ± 0.02 | 2.65* | __ | __ | __ | Guo et al., 2020 | |
Chick myoblasts | 3 weeks | 11.24 µm (Width)g 7.49 μm (Thickness)* | 0.0000661* | 1.44* | 21.89g | 3.31* | 50g | 2.28 | Santoso et al., 2021 | |
C2C12 myoblasts (mouse) | 16.30 µm (Width)g 10.86 μm (Thickness)* | 0.000139* | 0.027 | 0.2g | 0.018 | 0.129g | 0.64 | |||
Human myoblasts | 14.02 µm (Width)g 9.34 μm (Thickness)* | 0.0001028* | 0.020 | 0.2g | 0.019 | 0.182g | 0.91 | |||
Human induced pluripotent stem cell | Day 7–10 | 22.5 μm (Width)* 15 μm (Thickness) | 0.000265* | 0.26* | 0.986g | 0.52 | 1.986g | 2.01 | Al Tanoury et al., 2021 | |
Post deflection | Primary Mouse myoblasts | Day 1–12 | 2 mmi | 3.14* | __ | __ | 42.5g | 13.53* | __ | Vandenburgh et al., 2008# |
C2C12 myoblasts (mouse) | Day 14 | 0.14 ± 0.01 mm | 0.0125 (active) 0.0012 (effective) | 1.4* | 0.11 (active)* 1.12 (effective)* | __ | Sakar et al., 2012 | |||
C2C12 myoblasts (mouse) | Day 6 | 0.32 mmi | 0.079* | __ | __ | 57.5 ± 12.8 | 0.72* | Shimizu et al., 2017# | ||
Primary human myoblasts | Day 11 | 0.85 mm* | 0.566i | 79.44i | 0.14* | 428.57i | 0.76* | 5.42 | Mills et al., 2019 | |
Derived-Myoblasts from Human Dermal Fibroblast | Day 4–10 | 0.30 mmi | 0.120* | __ | __ | 12.2 ± 5.3 | 0.10* | __ | Shimizu et al., 2020 | |
Primary human myoblasts | Day 7–14 | 0.71 mm* | 0.395* | __ | __ | 192* | 0.49* | __ | Afshar et al., 2020 | |
Immortalized human myoblast | Day 8 | 0.4 mm | 0.125* | __ | __ | 28.5 ± 10.5 | 0.23 | __ | Nagashima et al., 2020 | |
Immortalized human myoblast | Day 7–14 | 0.47 mm* | 0.17 ± 0.03 | 200 ± 40 | 1.17 | 1100 ± 300 | 6.47 | 5.52 | Hofemeier et al., 2021 | |
Immortalized human myoblast | Day 10 | 0.49 mmi | 0.189* | 118.01g | 0.62* | 201.89g | 1.07* | 1.72 | Ebrahimi et al., 2021 | |
Force tranducers | Rat myoblasts (adult) | Day 31 ± 4 | 0.49 ± 0.04 mm | 0.188* | 215 ± 26 | 1.14* | 440 ± 45 | 2.9 ± 0.5 | 2.54 | Dennis and Kosnik, Ii, 2000 |
Rat myoblast (Extensor digitorum longus) | Day 32 ± 4 | 0.17 mm* | 0.024 ± 0.009 | 162 ± 125 | 6.75* | 281 ± 218 | 11.70* | 1.73 | Dennis et al., 2001 | |
Rat myoblasts | 3 weeks | 0.18 ± 0.01 mm | 0.0246* | 329 ± 26.3 | 13.37* | 805.8 ± 55 | 32.75 | 2.45 | Huang et al., 2005 | |
Rat myoblasts | Day 16–18 | 0.25 mmg | 0.048* | 102g | 2.12* | 212g | 4.41* | 2.08 | Larkin et al., 2006 | |
C2C12 myoblasts (mouse) | Day 5–8 | 0.21 mm* | 0.0978i | 71.39* | 0.73 ± 2.13 | 86.06* | 0.88 ± 0.48 | 1.20 | Fujita et al., 2009# | |
C2C12 myoblasts (mouse) | Day 2–17 | 0.2 mm* | 0.031* | 33.2 | 1.06 | __ | __ | __ | Yamamoto et al., 2011# | |
Rat myoblasts (neonatal) | Day 14 | 2.7 ± 0.18 mm (Bundle) | 5.72 (Bundle)* | 1680 ± 320 | 0.29* 5.5 ± 0.6 (effective) | 2840 ± 500 | 0.50* 9.4 ± 0.7 (effective) | 1.72 | Hinds et al., 2011 | |
C2C12 myoblasts (mouse) | Day 7 | 0.40 mm | 0.13* | 18.3 ± 2.4 | 0.15* | 34.5 ± 2.8 | 0.276* | 1.84 | Sato et al., 2013# | |
Rat myoblasts | 2 weeks | 1.38 mm (Bundle)* 0.9 mm (F-actin+) * | 1.50 (Bundle)g 0.63 ± 0.05 (F-actin+) | 17830 ± 1000 | 11.89 (Bundle)* 28.30 (F-actin+)* | 28800 ± 930 | 19.2 (Bundle)* 43.39 ± 3.82 (F-actin+) | 1.61 | Juhas and Bursac, 2014# | |
Primary Human myoblast | 4 weeks | 2.5 mmi | 4.91* | 701g | 0.14* | 1460g | 0.30* | 2.14 | Madden et al., 2015 | |
C2C12 myoblasts (mouse) | Day 14 | 0.5 ± 0.08 mm | 0.19* | 81.26g | 0.42* | 151.37g | 0.79* | 1.88 | Ikeda et al., 2016# | |
C2C12 myoblasts (mouse) | 3 weeks | 0.6 mmi | 0.28* | 166.3 ± 59.4 | 0.59* | __ | __ | __ | Nakamura et al., 2017 | |
hPSC derived human myoblasts | 2 weeks | 0.42 mmi | 0.14* | 140g | 1.04 | 402g | 3.00* | 2.88 | Rao et al., 2018 | |
C2C12 myoblasts (mouse) | Day 14 | 0.98 mmi | 0.756* | 48.39 ± 3.49 | 0.06 | 47.74 ± 0.31 | 0.06 | 1 | Capel et al., 2019 | |
Primary Human Myoblast | 2 weeks | 0.62 mmi | 0.30* | 1700 ± 130 | 5.70* | 3400 ± 180 | 11.40* | 2 | Khodabukus et al., 2019 | |
hPSC derived human myoblasts | 4 weeks | 0.28 mmi | 0.06* | 1393 ± 342 | 23.21* | 2924 ± 517 | 48.73* | 2.09 | Xu et al., 2019 | |
C2C12 myoblasts (mouse) | 10 Days | 0.99 mm | 0.77* | 1360 ± 210 | 1.77* | 1930 ± 120 | 2.50* | 1.41 | Akiyama et al., 2021 | |
Primary Human Myoblast | Day 17–19 | 1.39 mmi | 1.51* | __ | __ | 175* | 0.13* | __ | Alave Reyes-Furrer et al., 2021 |
-
*Recalculated data; gData extract from a graph; iData extract from an image; #Studies with where maximal instantaneous CF data was used.
-
Table 1—source data 1
Additional information specific to the experimental setup and stimulation parameters.
- https://cdn.elifesciences.org/articles/77204/elife-77204-table1-data1-v1.pdf
CF parameters
CF is affected by its relationship with different parameters, such as the frequency of the contractile stimulus (force-frequency), sarcomere length (force-length), and contraction velocity (force-velocity). Thus, an increase in stimulation frequency causes an increase in CF up to a maximal value (Fitts et al., 1991; Widmaier and Raff, 2008), although prolonged stimulation can lead to tissue fatigue and cause a decrease in CF over time (Grassi et al., 2015). In addition, contraction velocity is inversely related to cross-bridge formations and thus to CF production (Widmaier and Raff, 2008; Lindstedt et al., 2016). Power, defined by the product of CF and contraction velocity, is a measurable outcome of muscle contraction that reflects its efficiency and can be used to assess muscle fatigue (Alcazar et al., 2019). Finally, CF also depends on muscle length. An optimal length (L0) is where actin-myosin interactions are maximal and the muscle generates the highest tension (Fitts et al., 1991; Cheng et al., 2014a).
CF assessment should not be limited to determining the peak force value reached during contraction. It is rather preferred to analyze the complete contraction-relaxation profile. In essence, upon stimulation, skeletal muscle may present two types of mechanical profiles, as shown in Figure 1. An isolated contraction, or twitch response, is caused by a one-pulse stimulus that generates a single action potential (Widmaier and Raff, 2008). A tetanic contraction, in turn, is caused by a series of pulses whose frequency determines the contraction profile. Tetanic contraction may be unfused when the stimulation frequency allows the muscle to relax between pulses or fused when the interval between pulses is shorter than the time needed to reach relaxation. In the latter, the muscle exerts maximal CF (Widmaier and Raff, 2008; Wang et al., 2019).
Several parameters can be calculated from twitch and tetanus contraction profiles: The Tetanic-to-Twitch ratio, which compares maximal CF of these profiles, is independent from the size of the construct, and is affected by fatigue and maturation of muscle fibers, among others (Hill et al., 2021; Santoso et al., 2021). Time to peak (TTP) and half-relaxation time (RT50) can also be calculated from contraction profiles (Figure 1, lower graphs). These parameters contribute to evaluating contraction kinetics, and have revealed pathological phenotypes, such as impaired contractility in myotubes of ALS patients (Badu-Mensah et al., 2020).
Techniques for contractile force assessment
Hereafter, the most relevant techniques used to determine and measure CF in engineered skeletal muscle tissues, either for 2D or 3D configurations, are described: (1) cantilever deflection, (2) post deflection, or (3) force transducers. We also discuss alternative or emerging technologies for CF assessment, and provide a detailed comparison of the three main platforms currently in use.
Cantilever deflection
This technique is based on the deflection of a cantilever beam, which is fixed to one end and free for vertical movement on the other. The cantilever bends proportionally to the CF, and the variation of the height of the free end, or deflection, is measured by a laser and photodetector or image analysis (Wilson et al., 2007; Wilson et al., 2010; Fujita et al., 2010; Sun et al., 2013). Schematics in Figure 3 depict the cantilever setup with its three main states: resting, right after attachment of myoblasts, which are at a pre-differentiation state; initial deflection, due to the passive force exerted by the differentiated myotubes; and increased bending, caused by the CF that myotubes exert under stimuli. This force results in contraction of the cantilever’s upper side and a decrease in its length, while the lower surface of the beam remains relaxed with no change in its length. The differential strain distribution along cantilever thickness causes an upwards bending. Since one end of the cantilever is fixed, the entire bending effect is transformed into an uplift of its free end. Thus, myoblast’s horizontal contraction is transformed into a vertical displacement of the cantilever end.
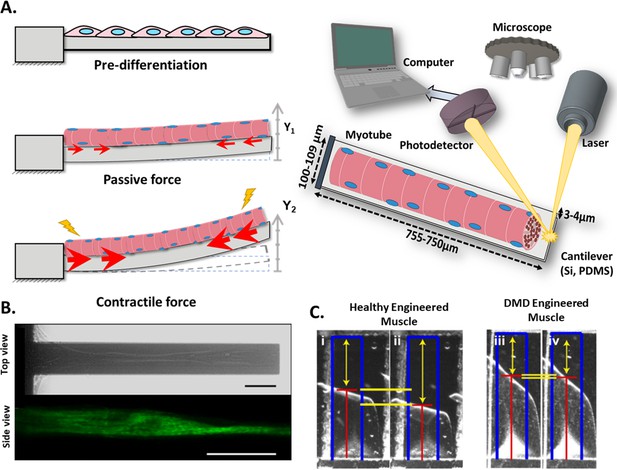
Cantilever deflection setup.
(A) The beam deflects due to myotube contraction (Left). In this case, cantilever deflection is interrogated by a laser beam and detected using a photodetector (Right). Commonly, cantilever arrays are made of Silicon (Si) or PDMS. Different coatings (FN, laminin, collagen I) have been tested to improve cell attachment and longer culture times. (B) Human myotubes on silicon cantilevers in bright field, top view (top) and immunostained for Myosin Heavy chain, side view (above). Scale bar: 50 µm. (C) Representative images from healthy and DMD myotubes at baseline (i and iii) and peak stress (ii and iv). Blue rectangles represent film length. Red lines represent the tracking of the film edge. Yellow arrows represent the distance between the projected film length and the unstressed film length. The yellow horizontal lines represent the change in projected film length from baseline stress (top bar) to peak stress (bottom bar).
© 2014, Elsevier. Figure 3B is reprinted with permission from Figure 1 from Smith et al., 2014. It is not covered by the CC-BY 4.0 license and further reproduction of this panel would need permission from the copyright holder.
© 2016, Nesmith et al. Figure 3C is reprinted with permission from Figure 5B from Nesmith et al., 2016 (published under a CC BY-NC-SA 3.0 license). It is not covered by the CC-BY 4.0 license and further reproduction of this panel would need permission from the copyright holder.
A backward analysis relating the measured displacement and the compression state of the cantilever allows for CF calculation. This computation can be resolved using Stoney’s equation (Wilson et al., 2007; Smith et al., 2014; Santoso et al., 2021), Finite Elements Analysis (FEA) (Pirozzi et al., 2013), or analytical solutions for the curvature radius (Wilson et al., 2007; Nesmith et al., 2016; Al Tanoury et al., 2021). The first two methods are best suited for a silicon cantilever setup, and according to Pirozzi and colleagues, Stoney’s equation provides enough accuracy for most applications. The FEA analysis is more mechanically rigorous but also computationally more complex. In this case, a laser-photodetector system is used to measure the vertical displacements, which are relatively small. The third approach is most appropriate for a polymeric film-like substrate in which image analysis will provide a change in the radius of curvature of the film. These materials allow larger deformations of the substrates (almost rolling) than the stiffer cantilevers. In either case, a thorough mechanical characterization of the system (e.g. thickness, flexibility) is required in order to feed the previous models.
These calculations assume that myotubes and substrates behave as one continuous solid element rather than two independent ones. Thus, myotubes need to be perfectly attached to the substrate. Special care needs to be taken to identify potential inhomogeneities within the culture or misalignments from the deflection axis since any slight deviation could render significant differences in CF assessment (Wilson et al., 2010). Techniques such as microcontact printing have been proposed to address these challenges, providing alignment and direct cell adhesion to the cantilever by using Collagen I (Guo et al., 2020), DETA (Wilson et al., 2010), or fibronectin (Nesmith et al., 2016; Sun et al., 2013). The use of coatings like DETA (Wilson et al., 2010) promotes long-term cell attachment (up to 21 days), which allows higher maturation of muscle constructs. Furthermore, the use of laminin and cell-tak, a tissue adhesive, enables evaluation of tetanic contractions in C2C12 myotubes with a reported tetanic-to-twitch ratio >1.5 (Fujita et al., 2010).
As a representative example, Figure 3B shows a top view (bright field) and a side view (fluorescence) image of a silicon cantilever with one single human myotube atop, and Figure 3C shows a representative image of a polymeric film-like structure with healthy and dystrophic myotubes under stimulation.
One important advantage of this technique is that there is no manipulation of the tissue at any time; hence, we consider this technique to be non-invasive and with high throughput potential. This 2D platform has been used to test different cell sources such as C2C12 (Fujita et al., 2010; Shimizu et al., 2010; Yamamoto et al., 2011; Pirozzi et al., 2013; Sun et al., 2013), myocytes from rats (McAleer et al., 2014; Wilson et al., 2010) or chicken (Santoso et al., 2021), human-induced pluripotent stem cells (hiPSCs) (Badu-Mensah et al., 2020; Guo et al., 2020), or primary human myoblast (Smith et al., 2014; Nesmith et al., 2016). Moreover, muscle disease models such as DMD (Nesmith et al., 2016; Al Tanoury et al., 2021) and amyotrophic lateral sclerosis (ALS) Badu-Mensah et al., 2020 have been evaluated on this platform, providing relevant information about the pathological mechanisms, and even testing novel treatments.
Post deflection
This technique is based on the quantification of the deflection of a pair of vertical microposts (µposts), due to contraction of the myobundle (Vandenburgh et al., 2008). The tissue construct, which is attached to the top part of the µposts, pulls them together during contraction, causing a horizontal displacement. This effect enables the calculation of the CF from equation (2), where R is the µpost pillar radius, L the length (height), E the elastic modulus, δ the µpost top displacement, and F is the CF of the muscle (Vandenburgh et al., 2008; Shimizu et al., 2017; Nagashima et al., 2020).
This equation corresponds to the deflection of a beam with a circular section. According to the classical principles of material science, the application of this expression assumes slender µpost profiles, exertion of myobundle force at the top of µposts (instead of a larger region as depicted in Figure 3), and a linear cantilever deflection in response to the exerted force. This is true if the stiffness of the µpost is at certain ranges, according to Sakar et al., 2012. They reported that an overly flexible cantilever might result in non-linear responses. Also, the effect of the top cap on the bending behavior of the µpost is negligible. However, if one would require higher fidelity on these calculations, finite element analysis could be used to include the thickness of the myobundle at the attachment site as well as any other structural feature. For example, the spring constant of the µposts was calculated with this methodology by including its exact geometry and the material properties (Hofemeier et al., 2021). In any case, a thorough characterization of the µposts is required prior to establishing the cell culture (i.e. µposts stiffness and shape [Vandenburgh et al., 2008; Christensen et al., 2020]).
Commonly, 3D skeletal muscle constructs are generated by embedding myoblasts in hydrogels (i.e. collagen, matrigel, fibrinogen) that simulate the native extracellular matrix (ECM) structure (Pollot et al., 2018) and provide the appropriate environment to develop the muscle structure. A casting process of the hydrogel and myoblasts allow the formation of the myobundle around the integrated µposts. Figure 4A (right) shows a graphical representation of this system, where the post deflection is assessed by a video camera. Figure 4A (Left) provide detailed representation of the three main states of the µposts: (1) resting, before the gelation or polymerization of the hydrogel, (2) initial deflection, due to the passive force exerted by the newly formed construct, and (3) increased deflection, caused by a contraction of the construct. The uniaxial passive force within the hydrogel (started at step 2) will promote myoblast alignment (Vandenburgh et al., 1996; Ruedinger et al., 2015; Thorrez et al., 2018) and myotube formation. Figure 4B shows a representative image of this displacement recorded from a top view before and after stimulation, and Figure 4C shows a typical sequence of the myobundle formation and an immunostaining image below, to show the alignment of the fibers and the formation of myotubes.
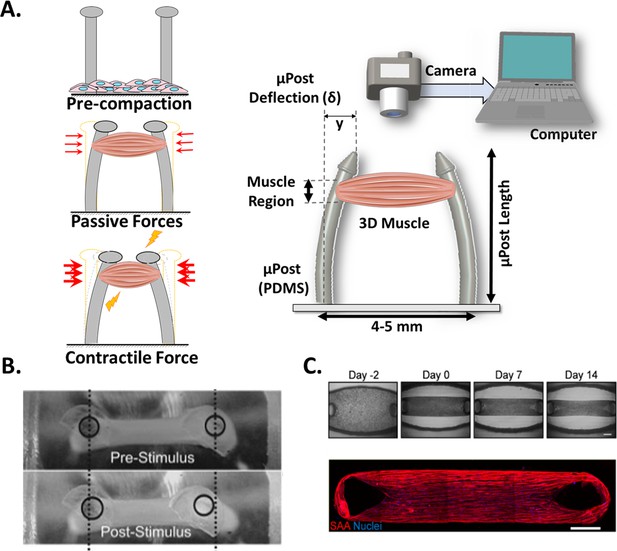
Post Deflection features.
(A) In vitro skeletal muscle is grown between two micropost which serve as anchors (tendons). As muscle contracts in response to a stimulus, posts bend proportionally. By tracking these displacements and knowing the mechanical characteristics of the platform, the force exerted by the muscle can be quantified. (B) Micropost displacement due to miniature bioartificial muscle (mBAM) contraction in response to a maximum tetanic electrical stimulus. Scale bar: 100 µm. (C) Formation of human skeletal muscle micro-tissue (hMMTs). Phase-contrast images depicting the remodeling of the ECM by human myoblast over time. Muscle construct immunostained (2 weeks) for sarcomeric α-actinin (SAA, red) and counterstained with DRAQ5 (1, 5-bis{[2-(di-methylamino)ethyl]amino}–4, 8-dihydroxyanthracene-9, 10-dione) nuclear stain in blue. Scale bar: 500 µm. Reprinted from Figure 2A and C from Afshar et al., 2020.
© 2008, John Wiley and Sons. Figure 4B is reprinted with permission from Figure 4A from Vandenburgh et al., 2008 with permission from John Wiley and Sons. It is not covered by the CC-BY 4.0 license and further reproduction of this panel would need permission from the copyright holder.
Vandenburgh and colleagues pioneered the development of this type of experimental setup in 2008 (Vandenburgh et al., 2008). Since then, many studies have been published using this platform, ranging from analysis of µpost design (Christensen et al., 2020), cellular sources (Yamamoto et al., 2009; Shimizu et al., 2020; Nagashima et al., 2020; Yoshioka et al., 2021a), drug screening assays (Afshar et al., 2020; Nagashima et al., 2020; Yoshioka et al., 2020) and passive forces (Agrawal et al., 2017), to the evaluation of contractility recovery following atrophy (Shimizu et al., 2017) or in a ALS-motor unit (Osaki et al., 2018b, Osaki et al., 2018a).
One advantage of this setup is that CF can be evaluated throughout the development of the muscle construct without causing disturbance, since information may be collected from the same sample as many times as required. Interestingly and perhaps due to its simplicity, this technique has encouraged the development of commercial platforms currently used to develop bioengineering cardiac tissues (Hansen et al., 2010). The major challenge is to track the deflection of µPosts accurately. In this line, fluorescent microbeads have been proposed as a means to easily follow µPost displacements (Sakar et al., 2012). For the above-mentioned reasons, we consider the post deflection method to be non-invasive, with high-throughput potential.
Many authors have adopted this experimental setup in combination with different stimulation protocols in order to enhance tissue maturation and induce contraction. For example, by chronical optical stimulation with ChR2, an increase of twitch and tetanic CF was observed in C2C12 (Sakar et al., 2012) and in human skeletal micro muscles (hµMs) (Mills et al., 2019). Alternatively, Kim and colleagues have used co-stimulation (mechanical and electrical) in a fascicle-like muscle model (eSMT), aiming ECM remodeling and thus CF improvement (Kim et al., 2019).
Force transducers
This technique is based on the use of a high-resolution force transducer coupled to the end of an engineered muscle (the other end is fixed to the substrate, as illustrated in Figure 5A). As the tissue contracts, it pulls from the transducer, allowing CF to be measured. The transducer converts the CF into a digital signal that can be recorded by a computer with the appropriate instrumentation.
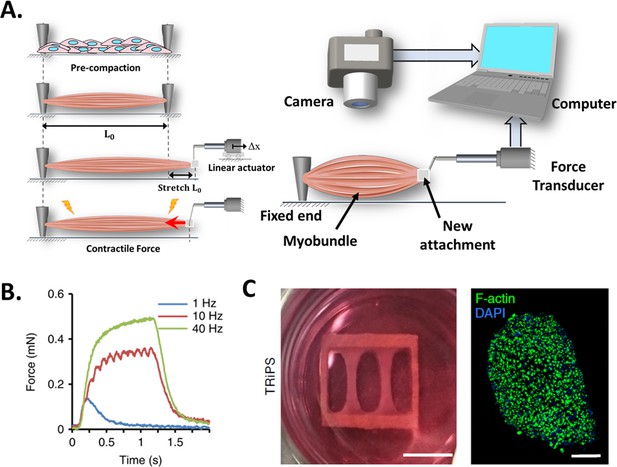
Force transducers.
(A) In vitro 3D tissue is grown between two anchors. To assess contraction force, one of its sides is connected to a force transducer which will evaluate the force exerted by the muscle due to stimuli. (B) Representative contractile properties of hPSC-derived iSKM bundles. TRiPS-derived bundle (4 weeks) shows increases in contractile force with an increase of stimulation frequency up to the formation of tetanic contraction. Specific force and tetanic-to-twitch ratio of H9 and TRiPS-derived bundle (2 weeks) and (C) (Left) two-week differentiated iSKM bundles pair anchored within a nylon frame. (Right) Representative immunostaining of dense, uniformly distributed myotubes in bundle-CSA. Panel B reprinted from Figures 3A, B and 4A from Rao et al., 2018.
Unlike the previous techniques, this setup only allows to perform endpoint measurements and requires manipulation of the 3D construct (invasive and low throughput), which may affect tissue structure and CF performance. Figure 5A (left) represents a step-by-step process of this setup. In short, the engineered muscle is grown in a specific bioreactor and then transferred to another setup where it is coupled to the force transducer (Alave Reyes-Furrer et al., 2021). This procedure is quite delicate as the muscle is prone to breakage. A linear actuator is then used to locate and set the construct to its original length and stretch the tissue above its baseline length (L0). Before taking any measurement, the construct may require time to stabilize and reacquire its resting state. Otherwise, tissue performance would be affected by the stress generated by the exchange of platforms.
Figure 5B shows a representative graph where the muscular construct has been subjected to a series of stimuli in order to determine its response to pulse frequency from twitch (1 Hz) to fused tetanic response (40 Hz). And Figure 5C presents a descriptive photograph of 2-week differentiated induced skeletal muscle bundles (iSKM) anchored within a nylon frame and a typical representative immunostaining of dense, uniformly distributed myotubes in bundle-CSA.
Researchers have used this platform to evaluate several conditions on 3D muscle constructs, such as cell sources (Dennis et al., 2001; Madden et al., 2015; Maffioletti et al., 2018; Rao et al., 2018), co-cultures (Larkin et al., 2006; Juhas et al., 2018) and media supplements (Fujita et al., 2009; Xu et al., 2019), as well as to perform drug screening assays (Ikeda et al., 2017; Zhang et al., 2018; Khodabukus et al., 2020). For example, Dennis and Kosnik generated three-dimensional skeletal muscle constructs from adult rats (myooids), to assess different CF parameters such as rheobase and chronaxie (Dennis and Kosnik, Ii, 2000), while Larkin et al., and Dhawan et al. evaluated the effect of muscle innervation on CF. Here, as expected the nerve-muscle constructs generated greated tetanic CF in vitro (212 µm vs 90 µN) and in vivo (649 ± 228µN vs 124 ± 31µN), respectively (Larkin et al., 2006; Dhawan et al., 2007).
Alternative and emerging technologies
Although force transducers, cantilevers, and post deflection are the most commonly used technologies to assess the production of CF in vitro, in this section we discuss several techniques that are being explored as an alternative way to quantify contraction kinetics: Traction force microscopy, contractility analysis, FRET, and microelectrode arrays (MEA).
Traction Force Microscopy (TFM) enables the measurement of local forces together with their directionality through the displacement tracking of micropillars or fluorescent microbeads embedded in elastic substrates (Ribeiro et al., 2016; Lemke and Schnorrer, 2017). This technique also allows the determination of traction forces along the entire length of the myotube, and throughout its differentiation process (Li et al., 2008). It is, thus, a non-invasive approach, which is also compatible with high-resolution microscopy. However, it presents some limitations inherent to the setup, such as the requirement of relatively low cell densities to track forces correctly, which may impact the size of de novo generated myotubes (Bruegmann et al., 2010). Thus, some authors use TFM with isolated native muscle fibers to overcome this limitation (Rausch et al., 2020). Inspired by this method other authors have determined the tensional status of hydrogels-containing human or C2C12 myotubes, by tracking deformable polyacrylamide fluorescent microbeads throughout the culture compaction and development (Hofemeier et al., 2021), or by detecting deformation of the substrate through Particle Imaging Velocity (Zhang et al., 2018; Cheesbrough et al., 2022).
Contractility-Pixel analysis relies on detecting changes in the position of the tissue by measuring the change of pixel intensity over time for a given region (Fujita et al., 2007; Kaji et al., 2010; Langhammer et al., 2010; Furuhashi et al., 2021). Overall, this technique does not provide a direct measure of CF, but instead, it resolves contraction and relaxation velocities and enables generation of curves, where contractility is usually presented as % of movement or as arbitrary units (Sala et al., 2018). Similar to TFM, this technique allows monitoring throughout culture development and it is also non-invasive. In contrast, image analysis is compatible with high-density cultures and even co-cultures. This method has been applied for drug-screening assays, and for the evaluation of contractility of individual cells and myotubes, to monolayers and 3D constructs (Kaji et al., 2010; Shimizu et al., 2015; Osaki et al., 2018a; Takahashi et al., 2018; Afshar Bakooshli et al., 2019; Vila et al., 2021).
Forster Resonance Energy Transfer (FRET) enables the measurement of contractile forces at the molecular level (pico Newtons, pN) based on changes of fluorescent emission upon the interaction of a pair of fluorescent probes (Grashoff et al., 2010; Shrestha et al., 2015; Wu et al., 2020). Changes in distance, stretching ( < 10–12 nm) tension, or orientation between probes modulates energy transfer, and thus the contraction measurements (Cost et al., 2015; Algar et al., 2019; Kaur and Dhakal, 2020). Interestingly, this method allows intracellular CF monitoring during culture development as it is non-invasive. However, it presents some specific challenges, such as an appropriate calibration to relate the light intensity with contraction force (Gates et al., 2019), the spatial-temporal resolution needed during contraction, and the lifespan of the fluorescence probes regarding the time required for differentiation processes. This procedure has been already applied to the evaluation of internal forces on F-actin and actinin using HEK-293 cells (Guo et al., 2014), in evaluating structural changes in the force-generating lever arm in myosin V (Gunther et al., 2020). It has also been applied in vivo to study the relationship between calcium handling and contraction in zebrafish beating hearts using the ratiometric biosensor Twitch-4 (Salgado-Almario et al., 2022). While further studies are needed to implement FRET for CF assessment of engineered skeletal muscles, we expect that it will become a valuable technique, complementary to the main techniques currently applied (Komatsubara et al., 2015).
Finally, Microelectrodes arrays (MEAs) systems have been used to characterize the electrical activity of neuron, cardiac or skeletal muscle cellular networks with a high spatial resolution. (Massobrio et al., 2015; Rabieh et al., 2016; Pasquarelli, 2021). These microelectrode arrays are able to record the bioelectric signals generated by the culture and/or stimulate them. Indeed, electrical stimulation has been already proven to be effective to promote myotube formation (Langhammer et al., 2013). But more importantly, this MEAs system can provide impedance monitoring to assess contractility, through the analysis of cell-microelectrode interactions. With each contraction, the changes of the shape of myotubes and the microelectrodes coverage, produces a perturbation in the AC current between pairs of electrodes proportional to the myotube movement allowing contraction analysis (Axion Biosystems, 2021). Even though this method does not provide quantitative CF measurements, it would provide relevant functional information of contraction kinetics.
CF platform comparison
There are a number of significant differences among the three main CF assessment methodologies, which are summarized in Figure 6. Generally, cantilevers are best suited for 2D models, myotubes, or muscular films, while µposts and force transducers are designed only for 3D tissue constructs, also known as myobundles. Besides the size, 2D cultures are usually kept in culture for a shorter time and present a risk of delamination, which increases with contractile activity. This partially explains the lower percentage of studies that have performed tetanic stimulation in 2D muscle models. In contrast, 3D models are cultured over longer periods, and present a lower risk of detachment from the µposts or anchoring structures. These 3D constructs are more resilient to chronic stimulation and better withstand tetanic stimulation (up to 90% of studies present this analysis). All three setups have been combined with electrical stimulation, typically by introducing platinum, gold, or carbon electrodes. However, optical and biochemical stimulation was only reported in cantilever and post deflection.
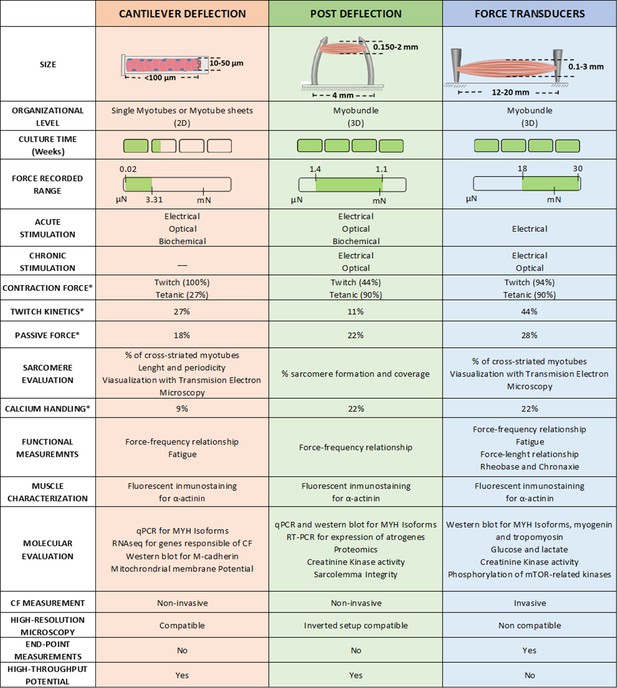
Overview of the different techniques used to measure contractile force in vitro.
* Represents de % of studies that have performed this measurement.
-
Figure 6—source data 1
Editable version of Figure 6.
- https://cdn.elifesciences.org/articles/77204/elife-77204-fig6-data1-v1.xlsx
The measured force range in Newtons for each platform is also significantly different, being 0.02–3.31 µN for the cantilever, 1.4 µN – 1.1 mN for µposts, and 18 µN – 30 mN for the transducer. These values are consistent with the sizes of the constructs each platform can accommodate. Figure 6 also presents the ratio of studies that presented the values of passive force (the contractile force at a resting state without stimulation) and twitch kinetics (PA, TTP, RT50). In addition, there are a number of contractile functional measurements that have been implemented in each platform: that is calcium handling, force-frequency relationship, fatigue, or force-length relationship. However, insufficient data is available to perform a fair comparison between studies (less than 20% of the studies evaluated these characteristics).
Regarding other functional outcomes, Figure 6 includes information about sarcomere evaluation, immunofluorescent imaging of α-actinin, and molecular expression (including genomic and proteomic). Most of these analyses are covered in all three setups, but the availability of biological material greatly differs depending on the setup. 3D muscle constructs provide larger amounts of tissue for analysis, enabling more extensive evaluations and assessments of scarcely expressed molecules.
Other characteristics unique to each setup need to be considered: Cantilever and posts deflection are potentially considered high throughput techniques, while force transducer platforms are not yet ready to handle many samples in parallel. Similarly, the first two are also non-invasive since there is no manipulation of the tissues to obtain the CF and allow for continuous monitoring of the evolution of the culture. The force transducer setup requires getting the myobundle or muscle construct attached to the sensor, and therefore, it becomes an end-point measurement. However, handling the myobundle allows control of the initial length of the myobundle prior to stimulation, enabling the analysis of additional variables. This feature is of most relevance as it can directly impact the force generated by the construct can generate. Another important feature is the compatibility of these techniques with high-resolution microscopy. While a 2D setup is easily integrated, 3D constructs have major limitations and require modification of the culturing setup. One should finally consider the complexity of the culture setup and the measuring platform, its cost, the access to appropriate facilities, as well as the required training/experience of the personnel operating these measurements. For instance, cantilever or µposts fabrication is quite complex since it requires microfabrication facilities or specific fabrication equipment, while no specific culturing protocols are required. On the contrary, the force transducer platform requires straightforward instrumentation, but it requires expert skills to manipulate the tissue construct correctly.
Contractile force data analysis
The previous section gathers a summary of the most relevant techniques used to quantify CF in vitro. There is a distinctive approach for 2D and 3D tissue models and the different methodologies used to assess CF in each of them. This section provides a uniform analysis of published data using the three methods described, enabling comparison of contractility across different models. It also provides insights into the development of disease models and evaluation of the effect of several pharmacological treatments.
Analysis criteria
It is relatively common to find CF values with different metrics or without proper clarification of morphological parameters such as sample width/thickness or CSA. This indetermination hinders a straightforward comparison between data collected using the different methodologies. Table 1 compares the morphological information (diameter and CSA) and the CF and sF of different healthy muscle constructs. CF data was directly extracted from the studies, time-average measurements were selected for both twitch and tetanus contraction unless these data were not provided in the study (noted with #). Maximum or instantaneous CF values were used as a necessary replacement in those cases. Additionally, it was often necessary to recalculate other parameters from the information provided by the authors (graphs or images), since it was either missing or provided in different units. Values that were recalculated are noted with an asterisk (*) in the table. To calculate the CF values, we have assumed the following premises:
Determination of the CSA (mm2): For 2D models we used the width (diameter) and thickness of the tissue, assuming an elliptical shape (). Calculations were based on diameter measurement assuming that myotube thickness is 1.5 times lower than diameter. For the 3D models, we worked with the circular cross-section of the sample ( = , where is the radius of the construct). Note that for the comparison among studies, all data have been normalized to the whole CSA (Figure 2).
If the authors present the sF directly rather than CF from twitch or tetanus, a backward calculation was made to ensure the comparison for either the CSA or CF.
Tetanic-to-Twitch Ratio was calculated from sF data within the same study (sFTe / sFTw), except for the post deflection experiments with C2C12 myotubes, where data unavailability forced the use of sFTe and sFTw data from different studies.
Muscle construct size and composition
The size of myotubes in vitro is smaller than the size of muscle cells in the native muscle tissue, commonly known as muscle fibers (Figure 1). While in the muscle fibers size ranges from 10 to 100 µm (King et al., 2004; Powell et al., 2002), the size of the myotubes in the cantilever deflection was 33.15 ± 23.831 µm and 13.29 ± 4.810 µm for C2C12 and human, respectively. This in vitro data is clearly within the lower range of the scale and could be related to the lack of maturation of the tissue.
In 3D muscle constructs in vitro, the size is limited by gas diffusion and, thus, it is difficult to scale up. Diameters of the engineered tissues range between 0.14–2 mm for post deflection and 0.17–3 mm for force transducers. While as shown in Figure 7A, the average-CSA ranges between 0.001–1 mm2 for post deflection, and 0.0001–5 mm2 for force transducers. Of note, the length of muscle constructs, which is independent of the diameter, is substantially higher in the force transducer platform (12–20 mm) compared to the one in µposts (around 4 mm) (Vandenburgh et al., 2008; Gholobova et al., 2020b).
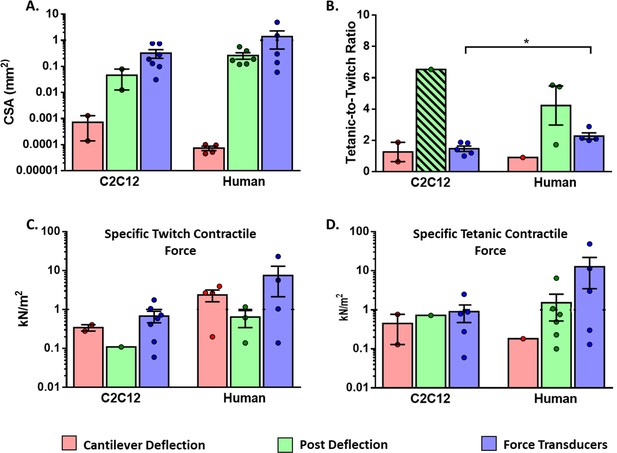
Functional characteristics of in vitro 2D and 3D skeletal muscle tissues from C2C12 and human sources (immortalized, iPSC and primary myoblast).
(A) Whole cross-sectional area (CSA) of muscle tissues. (B) Tetanic-to-Twitch ratio was calculated from data within the same study, except for bar with a diagonal pattern in post deflection, which was calculated from two different studies. (C) Twitch and Tetanic specific force measure in the three platforms for C2C12 constructs and (D) Human source. Data is presented as mean ± SEM. *p < 0.05, unpaired t-Test.
The dimensions of these constructs are affected by several variables such as the size of the culture platform (i.e. mold dimensions), hydrogel composition, and compaction (collagen vs. fibrinogen and matrigel) (Hinds et al., 2011; Pollot et al., 2018), culture protocols (i.e. stimulation during myogenesis), and cell culture density (Cvetkovic et al., 2014; Yoshioka et al., 2021a).
The scaffold composition, the type of proteins and their concentration, are also essential for tissue development and contraction performance. For example, time-to-tetanic peak was found almost two-times higher in 4 mg/ml fibrin-based hydrogels than in 2 mg/ml hydrogels. Additionally, when comparing fibrin with collagen I hydrogels, the latter exhibited lower twitch CF, tetanic CF, and tetanic-to-twitch ratio compared to fibrin hydrogels (Hinds et al., 2011). This can be partially explained by the elastic modulus of the hydrogel and the pore size. Collagen presents higher stiffness than fibrin, thus affecting the mechanical response of the tissue. The muscular cells need to overcome the elastic resistance provided by the hydrogel to exert proper contraction. On top of that collagen hydrogels present the smallest pore size and a higher degree of crosslinking fibers. Although this characteristic promotes differentiation, it also hinders cell migration (Pollot et al., 2018).
However, the core common issue in these 3D models is the number of myotubes and their localization within the scaffold. The cross-sectional area of native skeletal muscle presents a high and homogeneously distributed density of muscle fibers per area area (King et al., 2004). In contrast, histological studies of in vitro models have shown that myotubes are mainly in the outer ring of the constructs rather than homogeneously distributed along the cross-section (Nakamura et al., 2017). Thus, up to 90% of the CSA could be constituted by non-contractile tissue (Juhas and Bursac, 2014; Sakar et al., 2012; Akiyama et al., 2021; Ebrahimi et al., 2021), likely due to the lack of proper diffusion of nutrients and oxygen within the in vitro tissue (van der Schaft et al., 2011).
Several approaches such as tissue vascularization are being explored to overcome this hindrance (Gholobova et al., 2020a). For example, the use of porous biodegradable polymers promotes muscle differentiation and development of pre-vascular vessel-like structures (Levenberg et al., 2005). Other approaches include the use of sacrificial templates with thermo-responsive materials mimicking the vessel system (Wan et al., 2020), the development of aligned prevascularized muscles (van der Schaft et al., 2011), and the stimulation of angiogenesis and myogenesis through the exposure of myobundles to close-by engineered capillary networks (Osaki et al., 2018a).
Specific contractile force analysis
The performance of tissue constructs was analyzed by using Twitch Specific Force (Tw-sF), Tetanic Specific Force (Te-sF), and tetanic-to-twitch ratio parameters, which can be extracted or calculated from most studies (Table 1, Figure 7). In order to perform a consistent comparison, we have used the whole CSA (Figure 7A) to calculate sF, and segregated the data into two groups: muscle tissues generated with human myotubes or C2C12 myotubes. Of note, in this analysis human myotubes were generated from immortalized and primary human myoblasts, as well as human induced pluripotent stem cells (hPSC).
CSA data of muscle constructs (Figure 7A) was used to calculate sF generated in the different platforms (Figure 7C and D). These graphs show a vast data dispersion that required logarithmic scales. High dispersion and low data numbers prevented reaching statistical power for proper comparison among the different platforms. In any case, sF values of muscle constructs were much smaller (0.06–50 kN/m2) compared to the ones reported for native muscle, with maximal sF in the range of 100–700 kN/m2 (Maganaris et al., 2001; Urbanchek et al., 2001; Miller et al., 2015; Jeon et al., 2019).
On the contrary, tetanic-to-twitch data in Figure 7B presented low variability. This parameter was calculated from Te-sF and Tw-sF data within the same study, except for the case of C2C12 in µposts that was calculated from two different studies, due to lack of data availability. Interestingly, for the force transducer platform, we found that the tetanic-to-twitch ratio was significantly higher in human muscle compared to C2C12 muscle constructs (unpaired t-test, p < 0.05). We are uncertain about the relevance of this finding, but it is likely an intrinsic feature related to the species since gender-specific differences have been found for this parameter in native mouse muscles (Lafoux et al., 2020). Of note, this parameter is independent of CSA and provides a functional measurement that could be used to compare different studies regardless of the construct size or platform used.
Contractile force evaluation in disease models
Evaluation of CF in these platforms has also been successfully used to characterize the phenotype of muscle disease models. Table 2 shows a collection of the most relevant studies where CF is assessed for different drug-induced models or patient-dystrophic models. Remarkably, many of the studies have used human cell lines or primary cells from donor patients. The CF analysis confirmed that the disease engineered tissues exhibit an overall lower sF and poorer morphological characteristics compared to their healthy controls.
Summary of several skeletal muscle disease models in post deflection and force transducer platforms.
Key parameters like drugs, change in CF (%∆CF) and other observed effects are detailed.
Disease model | Drug | Platform | Cell source | %∆CF(Dose) | Observed effect | Reference |
---|---|---|---|---|---|---|
Atrophy | Dexamethasone | Post deflection | C2C12 myoblast (mouse) | –53% (100 µM) | Increase in the expression of Atrogin-1 (2.6) and MuRF-1 (2.2) Decrease in number of fibers with striation patterns (20% vs 8%) | Shimizu et al., 2017 |
Immortalized human myogenic cells | –57% (100 µM) | Increase in the expression of Atrogin-1 and MuRF-1. Expression of FOXO3 and KLF15 | Nagashima et al., 2020 | |||
Primary Human myoblast | –85% (10 nM) | Dose-dependent decrease in myotube width | Afshar et al., 2020 | |||
C2C12 myoblast (mouse) | –48% (1 mM) | Yoshioka et al., 2020 | ||||
Force transducer | Primary Human myoblast | –67% (25 µM) | Decrease in myotube diameter (25 µM, 12%) Decrease in effective-CSA (60%) Decrease in injury biomarkers CK and LDH | Khodabukus et al., 2020 | ||
C2C12 myoblast (mouse) | –70% (100 µM) | Decrease in myotube-CSA (37%) | Aguilar-Agon et al., 2021 | |||
Hypertrophy | IGF-1 | Force transducer | Rat myoblast | + 31% (75 ng) | Increase in CF (75 ng, 31%) Slow Time to peak twitch force (25 ng, 26%) | Huang et al., 2005 |
Primary Human myoblast | + 28% (0.5 mg/ml) | Increase in myotube diameter (0.5 mg/ml, 21%) Increase in injury biomarkers CK and LDH | Khodabukus et al., 2020 | |||
Post deflection | Primary Mouse myoblast | + 66% (100 ng/ml) | Increase in fiber-CSA (41%) | Vandenburgh et al., 2008 | ||
Immortalized control C57 and mdx myoblast | + 93% (0.01 µM) | Vandenburgh et al., 2009 | ||||
C2C12 myoblast (mouse) | + 25% | Increase in CF in Dex-induced atrophic tissues (45%*) | Shimizu et al., 2017 | |||
Derived myoblast from human dermal fibroblast | + 72% (100 ng/ml) | Decrease in CF in non-cryopreserved cells (100 ng/ml, 79%*) | Shimizu et al., 2020 | |||
Statin-induced myopathy (Rhabdomyolysis) | Lovastatin | Force transducer | Primary Human myoblast | –75% (2 µM) | Dose-dependent lipid accumulation | Madden et al., 2015 |
Primary Human myoblast | –53% (5 µM) | Dose-dependent lipid accumulation | Ananthakumar et al., 2020 | |||
Post deflection | Immortalized human myogenic cells | –75% (2 µM) | Increase in the expression of Atrogin-1 and MuRF-1. | Nagashima et al., 2020 | ||
Cerivastatin | Force transducer | Primary Human myoblast | –50% (50 nM) | Decrease in CF (50 nM, 50%*) Dose-dependent lipid accumulation | Madden et al., 2015 | |
Primary Human myoblast | –85% | Reduction in myotube diameter Dose-dependent decrease in injury biomarkers CK and LDH | Vandenburgh et al., 1996 | |||
Human Skeletal myoblast | –40% (100 nM) | Decrease in CF Decrease in passive force Myofibers Sarcomere degradation | Zhang et al., 2018 | |||
Post deflection | Primary Human myoblast | –62% (10 nM) | Decrease in CF (10 nM, 62%*) Dose-dependent decrease in myotube width | Alave Reyes-Furrer et al., 2021 |
-
*Recalculated data.
As shown in Table 2, the most common drugs used in these models are: dexamethasone, a synthetic glucocorticoid commonly used to induce muscle atrophy (Aguilar-Agon et al., 2021), and insulin growth factor 1 (IGF-1), which activates muscle stem cells (satellite cells, SCs) and regulates anabolic and catabolic pathways in skeletal muscle (Yoshida and Delafontaine, 2020). All these studies have shown that CF decreases or increases in a dose-dependent manner when dexamethasone or IGF-1 are applied, respectively. Authors have also identified changes in myotubes diameter and in the percentage of fibers with striation patterns or the expression of specific biomarkers. Finally, other drugs such as lovastatin (Madden et al., 2015; Afshar et al., 2020; Ananthakumar et al., 2020; Nagashima et al., 2020) and cerivastatin Brady et al., 2008; Madden et al., 2015; Zhang et al., 2018 have been used to generate statin-induced myopathy models in these systems.
Discussion
Regardless of the experimental platform used or the size of the construct, in vitro muscle constructs present a significantly smaller CF compared to native tissue (0.2–2.9% of native muscle Tw-sF). Gene expression analyses performed on engineered constructs have shown that they are similar to fetal muscles and far removed from adult native muscle (Nagashima et al., 2020). The general conclusion is that engineered tissues are still at an immature stage of development. Indeed, some of the latest experimental approaches consider using co-culture with neural cells to stimulate NMJ formation, muscle innervation (Demestre et al., 2015; Zahavi et al., 2015; Rimington et al., 2021), and tissue maturation.
Among the main experimental platforms, the force transducer studies are the ones that have generated the highest amount of CF data. Moreover, this platform reports the highest CF mean values among the in vitro muscle constructs. Since constructs evaluated by the force transducer platforms are also the ones with the largest muscle mass, a proper normalization is necessary to compare the CF of the engineered muscles between the different platforms. When normalizing CF to total CSA, we found a very high data variability for all the parameters analyzed (twitch, tetanus), both in constructs generated by C2C12 cells and human cells. However, the tetanic-to-twitch ratio presented a low data variability, enabling comparison between different studies. Tetanic-to-Twitch ratio is a parameter independent of the CSA, which indicates that the total CSA used is not adequate for CF data normalization among studies, even those using the same platform.
There are several aspects influencing contractile function that largely differ across studies, that is, assessment time, tissue maturation, contractile area, and length and mass of the muscle construct. For instance, the percentage of myotubes with a cross-striated pattern, which accounts for the muscle maturation level, appears to be higher in muscle constructs assessed by force transducers ( > 95%) compared to µpost (73.52%) and cantilever platforms (12%) (Hinds et al., 2011; Ebrahimi et al., 2021; Al Tanoury et al., 2021). Importantly, none of these variables are normalized by total CSA, and many are rarely reported in the literature. One possibility for future analyses would be to use the effective CSA, considering the contractile area. This can be achieved with immunofluorescence analysis of sarcomeric proteins (Sakar et al., 2012; Juhas and Bursac, 2014). In addition, this analysis could also provide further valuable data such as muscle maturity level (cross-striation) and sarcomere length, which relates to optimal muscle length.
Our comparative analysis across the experimental platforms shows that sF values reported with the post deflection platform are substantially lower than those with force transducers. This may be due to several factors, including a lower contractile area, lower maturation level, and/or a suboptimal sarcomeric length. In the force transducer, the initial length, L0, in the resting state before contraction stimulation is adjustable, allowing pre-tension of the construct and maximization of CF. However, it is unattainable to control muscle length in the post deflection method or the cantilever system. In µposts, there is a shortening of L0 caused by the passive tension of the construct, that is, a progressive contraction of the cells and hydrogel during maturation. Muscle construct shortening in µposts may vary depending on the elastic modulus and would be more pronounced in hydrogels presenting lower elastic properties, such as fibrin, than collagen (Hinds et al., 2011; Cvetkovic et al., 2014). Due to limited available data, it is not possible to determine to what extent this factor affects the sF readout of µposts experiments. However, data extracted from few selected studies suggest that the sarcomere length does not differ substantially between cantilever deflection (1.58 µm, Santoso et al., 2021), post-deflection (2.76 µm, Ebrahimi et al., 2021) and force transducer platforms (1.5–2.7 µm, Hinds et al., 2011; Rao et al., 2018). Moreover, these data are in line with the optimal sarcomere length (about 2 µm) that produces the maximum tension in native muscles (Moo et al., 2020).
Another factor that may account for the lower sF values in µpost muscle constructs compared to force transducers is the muscle mass. Thus, in the force transducer platform, the muscle construct is undoubtedly longer, about 12–20 mm (Gholobova et al., 2020b) compared to 4 mm, in the case of µposts (Vandenburgh et al., 2008). The total CSA would not fully normalize for longer muscle constructs with similar CSA, where more muscle mass contributes to muscle contraction. Hence, an inappropriate CF normalization may partly account for higher sF values observed on average in force transducer constructs compared to post-deflection.
Finally, an unexpected finding derived from our analysis is the overall higher tetanic-to-twitch ratio values of muscle constructs in µpost (1.5–5.6) compared to constructs assessed by force transducers (1-3). Native rat and human muscles present tetanic-to-twitch ratios in the range of 4–10 (Cheng et al., 2014a), so constructs in µposts appear to recapitulate more closely this feature of native muscles. We hypothesize that constructs assessed using force transducers may suffer undue stress due to the additional manipulation required for FC measurement, which may cause malfunction or fatigue, limiting the tetanic response.
Multiple physiological conditions still need to be adjusted and accounted for in order to achieve higher and more reliable contractile performance data in engineered muscles. In light of the CF and tetanic-to-twitch ratio data, it appears that the use of the ratio would limit the variability of CF data, rendering it a useful parameter for CF data comparison across studies.
Conclusions and future challenges
CF is a key parameter used to assess the actual functionality of in vitro muscle models. In this review, we have discussed the three main methodologies commonly used to measure CF in muscle in vitro systems: cantilever deflection, post deflection, and force transducers. These platforms cover the evaluation of 2D and 3D in vitro skeletal muscle models, from myotubes to muscle engineered tissue in healthy and disease models.
Currently, there is no standard parameter to express CF for in vitro muscle systems. Thus, we have used specific contractile force (sF), by normalizing either twitch or tetanic contraction with the calculated whole cross-sectional area of the engineered tissue in order to compare all the relevant studies. We believe, however, that a more accurate way to calculate sF would be to use the effective-CSA to normalize CF. This would require analyzing the specific number of myotubes in the CSA, which is unfortunately not available in many studies up to date. Therefore, for future studies, we propose that contractility studies in skeletal muscle constructs should include information related to the construct size (length and diameter), contractile area (i.e. myotube coverage in CSA), maturity level (i.e. % of myotubes showing cross-striations), and sarcomere length to complement the information on contractile performance. In any case, we highly recommend including the tetanic-to-twitch ratio since this parameter provides unique information about muscle contractile performance. Furthermore, our analysis suggests that it may enable proper comparison among different studies. Future studies will help us understand the relative impact of these variables on the force performance of muscle constructs and, consequently, the best methodology for measuring and normalizing CF data.
To date, 3D models have evidenced the highest CF; however sF values present a high variability, with no significant differences among the platforms, and they are still far from the native sF range. There is a certain consensus that the cause of this reduced contractile capacity is a maturation deficit of the tissue constructs. In this line, different approaches are being developed to obtain more physiologically relevant muscle models by implementing vascularization, innervation, and mechanical or electromechanical stimulation. Nevertheless, how these new experimental developments would influence the CF of engineered muscle constructs remains unresolved.
Even though CF is a practical, distinctive parameter for assessing muscle function, it is highly influenced by intrinsic properties of the muscle constructs, so the assessment should be complemented alongside other methodologies, such as immunofluorescence, Ca2+ handling, and protein quantification. Future investigations should provide insights into integrating this information with contractile values reported in the literature. Moreover, we expect the emerging CF assessment technologies to provide unique insights into intracellular contractile mechanisms.
Given the growing interest in 3D models and the advances in this field, we anticipate a new generation of in vitro systems that will become the driving force behind subsequent research discoveries. Thus, there are still many opportunities for cooperation in these developments and a need for creative and technological researchers to make it possible.
References
-
Mechanical loading stimulates hypertrophy in tissue-engineered skeletal muscle: Molecular and phenotypic responsesJournal of Cellular Physiology 234:23547–23558.https://doi.org/10.1002/jcp.28923
-
Mechanical loading of tissue engineered skeletal muscle prevents dexamethasone induced myotube atrophyJournal of Muscle Research and Cell Motility 42:149–159.https://doi.org/10.1007/s10974-020-09589-0
-
Mechanical stretch is a down-regulatory signal for differentiation of C2C12 myogenic cellsMaterials Science and Engineering 17:75–78.https://doi.org/10.1016/S0928-4931(01)00340-X
-
An Electrical Stimulation Culture System for Daily Maintenance-Free Muscle Tissue ProductionCyborg and Bionic Systems 2021:1–12.https://doi.org/10.34133/2021/9820505
-
Optically controlled contraction of photosensitive skeletal muscle cellsBiotechnology and Bioengineering 109:199–204.https://doi.org/10.1002/bit.23285
-
Patterning the differentiation of C2C12 skeletal myoblastsIntegrative Biology 3:897.https://doi.org/10.1039/c1ib00058f
-
Regulating Myoblast Phenotype Through Controlled Gel Stiffness and DegradationTissue Engineering 13:1431–1442.https://doi.org/10.1089/ten.2006.0356
-
Human skeletal muscle fibres: molecular and functional diversityProgress in Biophysics and Molecular Biology 73:195–262.https://doi.org/10.1016/s0079-6107(00)00006-7
-
Synergy between myogenic and non-myogenic cells in a 3D tissue-engineered craniofacial skeletal muscle constructJournal of Tissue Engineering and Regenerative Medicine 2:408–417.https://doi.org/10.1002/term.112
-
Optogenetic control of heart muscle in vitro and in vivoNature Methods 7:897–900.https://doi.org/10.1038/nmeth.1512
-
Special Issue “Novel Research about Biomechanics and Biomaterials Used in Hip, Knee and Related Joints.”Journal of Applied Biomaterials & Biomechanics 8:4829.https://doi.org/10.5301/JABB.2010.4829
-
Scalable 3D Printed Molds for Human Tissue Engineered Skeletal MuscleFrontiers in Bioengineering and Biotechnology 7:20.https://doi.org/10.3389/fbioe.2019.00020
-
Biobased Elastomer Nanofibers Guide Light-Controlled Human-iPSC-Derived Skeletal MyofibersAdvanced Materials (Deerfield Beach, Fla.) 1:e2110441.https://doi.org/10.1002/adma.202110441
-
Physiology and metabolism of tissue-engineered skeletal muscleExperimental Biology and Medicine 239:1203–1214.https://doi.org/10.1177/1535370214538589
-
Conditions that promote primary human skeletal myoblast culture and muscle differentiation in vitroAmerican Journal of Physiology-Cell Physiology 306:C385–C395.https://doi.org/10.1152/ajpcell.00179.2013
-
How to Measure Molecular Forces in Cells: A Guide to Evaluating Genetically-Encoded FRET-Based Tension SensorsCellular and Molecular Bioengineering 8:96–105.https://doi.org/10.1007/s12195-014-0368-1
-
Biofabricating murine and human myo-substitutes for rapid volumetric muscle loss restorationEMBO Molecular Medicine 13:e12778.https://doi.org/10.15252/emmm.202012778
-
Excitability and isometric contractile properties of mammalian skeletal muscle constructs engineered in vitroexcitability and isometric contractile properties of mammalian skeletal muscle constructs engineered in vitroIn Vitro Cellular & Developmental Biology - Animal 36:327.https://doi.org/10.1290/1071-2690(2000)036<0327:EAICPO>2.0.CO;2
-
Excitability and contractility of skeletal muscle engineered from primary cultures and cell linesAmerican Journal of Physiology. Cell Physiology 280:C288–C295.https://doi.org/10.1152/ajpcell.2001.280.2.C288
-
Neurotization improves contractile forces of tissue-engineered skeletal muscleTissue Engineering 13:2813–2821.https://doi.org/10.1089/ten.2007.0003
-
A novel bioreactor for stimulating skeletal muscle in vitroTissue Engineering. Part C, Methods 16:711–718.https://doi.org/10.1089/ten.TEC.2009.0125
-
Bioengineered in vitro skeletal muscles as new tools for muscular dystrophies preclinical studiesJournal of Tissue Engineering 12:1–19.https://doi.org/10.1177/2041731420981339
-
The determinants of skeletal muscle force and power: their adaptability with changes in activity patternJournal of Biomechanics 24 Suppl 1:111–122.https://doi.org/10.1016/0021-9290(91)90382-w
-
Accelerated de novo sarcomere assembly by electric pulse stimulation in C2C12 myotubesExperimental Cell Research 313:1853–1865.https://doi.org/10.1016/j.yexcr.2007.03.002
-
Novel method for fabrication of skeletal muscle construct from the C2C12 myoblast cell line using serum-free medium AIM-VBiotechnology and Bioengineering 103:1034–1041.https://doi.org/10.1002/bit.22318
-
Designing of a Si-MEMS device with an integrated skeletal muscle cell-based bio-actuatorBiomedical Microdevices 13:123–129.https://doi.org/10.1007/s10544-010-9477-3
-
Skeletal muscle fatigue and decreased efficiency: two sides of the same coin?Exercise and Sport Sciences Reviews 43:75–83.https://doi.org/10.1249/JES.0000000000000043
-
FRET and optical trapping reveal mechanisms of actin activation of the power stroke and phosphate release in myosin VThe Journal of Biological Chemistry 295:17383–17397.https://doi.org/10.1074/jbc.RA120.015632
-
Development of a drug screening platform based on engineered heart tissueCirculation Research 107:35–44.https://doi.org/10.1161/CIRCRESAHA.109.211458
-
Screening out irrelevant cell-based models of diseaseNature Reviews. Drug Discovery 15:751–769.https://doi.org/10.1038/nrd.2016.175
-
Rapid formation of functional muscle in vitro using fibrin gelsJournal of Applied Physiology (Bethesda, Md 98:706–713.https://doi.org/10.1152/japplphysiol.00273.2004
-
Sex- and fiber-type-related contractile properties in human single muscle fiberJournal of Exercise Rehabilitation 15:537–545.https://doi.org/10.12965/jer.1938336.168
-
Incorporation of macrophages into engineered skeletal muscle enables enhanced muscle regenerationNature Biomedical Engineering 2:942–954.https://doi.org/10.1038/s41551-018-0290-2
-
Recent applications of FRET-based multiplexed techniquesTrAC Trends in Analytical Chemistry 123:115777.https://doi.org/10.1016/j.trac.2019.115777
-
Defined electrical stimulation emphasizing excitability for the development and testing of engineered skeletal muscleTissue Engineering. Part C, Methods 18:349–357.https://doi.org/10.1089/ten.TEC.2011.0364
-
Factors That Affect Tissue-Engineered Skeletal Muscle Function and PhysiologyCells Tissues Organs 202:159–168.https://doi.org/10.1159/000446067
-
Force Generation for Locomotion of Vertebrates: Skeletal Muscle OverviewIEEE Journal of Oceanic Engineering 29:684–691.https://doi.org/10.1109/JOE.2004.833205
-
Relationship between force and size in human single muscle fibresExperimental Physiology 96:539–547.https://doi.org/10.1113/expphysiol.2010.055269
-
Advanced maturation by electrical stimulation: Differences in response between C2C12 and primary muscle progenitor cellsJournal of Tissue Engineering and Regenerative Medicine 5:529–539.https://doi.org/10.1002/term.345
-
Identification and quantification of skeletal myotube contraction and association in vitro by video microscopyCytoskeleton (Hoboken, N.J.) 67:413–424.https://doi.org/10.1002/cm.20457
-
A topographically modified substrate-embedded MEA for directed myotube formation at electrode contact sitesAnnals of Biomedical Engineering 41:408–420.https://doi.org/10.1007/s10439-012-0647-8
-
Functional evaluation of nerve-skeletal muscle constructs engineered in vitroIn Vitro Cellular & Developmental Biology. Animal 42:75–82.https://doi.org/10.1290/0509064.1
-
Mechanical forces during muscle developmentMechanisms of Development 144:92–101.https://doi.org/10.1016/j.mod.2016.11.003
-
Engineering vascularized skeletal muscle tissueNature Biotechnology 23:879–884.https://doi.org/10.1038/nbt1109
-
A novel functional assessment of the differentiation of micropatterned muscle cellsJournal of Biomechanics 41:3349–3353.https://doi.org/10.1016/j.jbiomech.2008.09.025
-
Skeletal muscle tissue in movement and health: positives and negativesThe Journal of Experimental Biology 219:183–188.https://doi.org/10.1242/jeb.124297
-
In vivo specific tension of human skeletal muscleJournal of Applied Physiology (Bethesda, Md 90:865–872.https://doi.org/10.1152/jappl.2001.90.3.865
-
Optogenetics, Tools and Applications in NeurobiologyJournal of Medical Signals and Sensors 7:71–79.
-
Leucine elicits myotube hypertrophy and enhances maximal contractile force in tissue engineered skeletal muscle in vitroJournal of Cellular Physiology 232:2788–2797.https://doi.org/10.1002/jcp.25960
-
Electrical stimulation of excitable tissue: design of efficacious and safe protocolsJournal of Neuroscience Methods 141:171–198.https://doi.org/10.1016/j.jneumeth.2004.10.020
-
Molecular determinants of force production in human skeletal muscle fibers: effects of myosin isoform expression and cross-sectional areaAmerican Journal of Physiology-Cell Physiology 308:C473–C484.https://doi.org/10.1152/ajpcell.00158.2014
-
The sarcomere force-length relationship in an intact muscle-tendon unitThe Journal of Experimental Biology 223:jeb215020.https://doi.org/10.1242/jeb.215020
-
Cyclic mechanical preconditioning improves engineered muscle contractionTissue Engineering. Part A 14:473–482.https://doi.org/10.1089/tea.2007.0104
-
Development and evaluation of a removable tissue-engineered muscle with artificial tendonsJournal of Bioscience and Bioengineering 123:265–271.https://doi.org/10.1016/j.jbiosc.2016.08.003
-
A human in vitro model of Duchenne muscular dystrophy muscle formation and contractilityThe Journal of Cell Biology 215:47–56.https://doi.org/10.1083/jcb.201603111
-
Electrical pulse stimulation of cultured skeletal muscle cells as a model for in vitro exercise - possibilities and limitationsActa Physiologica (Oxford, England) 220:310–331.https://doi.org/10.1111/apha.12830
-
Effects of electrical stimulation in C2C12 muscle constructsJournal of Tissue Engineering and Regenerative Medicine 2:279–287.https://doi.org/10.1002/term.93
-
ConferenceMicroelectrode Arrays, Implants, and Organs-on-a-chipIn Biosensors and Biochips. pp. 291–322.https://doi.org/10.1007/978-3-030-76469-2
-
Fabrication of hybrid scaffolds obtained from combinations of PCL with gelatin or collagen via electrospinning for skeletal muscle tissue engineeringJournal of Biomedical Materials Research. Part A 109:1600–1612.https://doi.org/10.1002/jbm.a.37156
-
Natural polymeric hydrogel evaluation for skeletal muscle tissue engineeringJournal of Biomedical Materials Research. Part B, Applied Biomaterials 106:672–679.https://doi.org/10.1002/jbm.b.33859
-
Mechanical stimulation improves tissue-engineered human skeletal muscleAmerican Journal of Physiology. Cell Physiology 283:C1557–C1565.https://doi.org/10.1152/ajpcell.00595.2001
-
Use of flow, electrical, and mechanical stimulation to promote engineering of striated musclesAnnals of Biomedical Engineering 42:1391–1405.https://doi.org/10.1007/s10439-013-0966-4
-
For whom the cells pull: Hydrogel and micropost devices for measuring traction forcesMethods (San Diego, Calif.) 94:51–64.https://doi.org/10.1016/j.ymeth.2015.08.005
-
Hydrogels for 3D mammalian cell culture: a starting guide for laboratory practiceApplied Microbiology and Biotechnology 99:623–636.https://doi.org/10.1007/s00253-014-6253-y
-
Striated muscle function, regeneration, and repairCellular and Molecular Life Sciences 73:4175–4202.https://doi.org/10.1007/s00018-016-2285-z
-
Assembly of skeletal muscle cells on a Si-MEMS device and their generative force measurementBiomedical Microdevices 12:247–252.https://doi.org/10.1007/s10544-009-9379-4
-
Microfluidic devices for construction of contractile skeletal muscle microtissuesJournal of Bioscience and Bioengineering 119:212–216.https://doi.org/10.1016/j.jbiosc.2014.07.003
-
Fabrication of contractile skeletal muscle tissues using directly converted myoblasts from human fibroblastsJournal of Bioscience and Bioengineering 129:632–637.https://doi.org/10.1016/j.jbiosc.2019.11.013
-
Understanding FRET as a research tool for cellular studiesInternational Journal of Molecular Sciences 16:6718–6756.https://doi.org/10.3390/ijms16046718
-
Effect of heat stress on contractility of tissue-engineered artificial skeletal muscleJournal of Artificial Organs 21:207–214.https://doi.org/10.1007/s10047-018-1020-y
-
Calpain 3 deficiency affects SERCA expression and function in the skeletal muscleExpert Reviews in Molecular Medicine 18:e7.https://doi.org/10.1017/erm.2016.9
-
ConferenceA Novel Functional In Vitro Model that Recapitulates Human Muscle DisordersIn Muscle Cell and Tissue - Current Status of Research Field. InTech.
-
Specific force deficit in skeletal muscles of old rats is partially explained by the existence of denervated muscle fibersThe Journals of Gerontology. Series A, Biological Sciences and Medical Sciences 56:B191–B197.https://doi.org/10.1093/gerona/56.5.b191
-
Dysregulation of calcium homeostasis in muscular dystrophiesExpert Reviews in Molecular Medicine 16:e16.https://doi.org/10.1017/erm.2014.17
-
Longitudinal growth of skeletal myotubes in vitro in a new horizontal mechanical cell stimulatorIn Vitro Cellular & Developmental Biology 25:607–616.https://doi.org/10.1007/BF02623630
-
Mechanically induced alterations in cultured skeletal muscle growthJournal of Biomechanics 24:91–99.https://doi.org/10.1016/0021-9290(91)90380-6
-
Tissue-engineered skeletal muscle organoids for reversible gene therapyHuman Gene Therapy 7:2195–2200.https://doi.org/10.1089/hum.1996.7.17-2195
-
High-content drug screening with engineered musculoskeletal tissuesTissue Engineering. Part B, Reviews 16:55–64.https://doi.org/10.1089/ten.TEB.2009.0445
-
Förster resonance energy transfer (FRET)-based small-molecule sensors and imaging agentsChemical Society Reviews 49:5110–5139.https://doi.org/10.1039/c9cs00318e
-
Preparation of artificial skeletal muscle tissues by a magnetic force-based tissue engineering techniqueJournal of Bioscience and Bioengineering 108:538–543.https://doi.org/10.1016/j.jbiosc.2009.05.019
-
Novel neuromuscular junction model in 2D and 3D myotubes co-cultured with induced pluripotent stem cell-derived motor neuronsJournal of Bioscience and Bioengineering 129:486–493.https://doi.org/10.1016/j.jbiosc.2019.10.004
-
Miniaturized skeletal muscle tissue fabrication for measuring contractile activityJournal of Bioscience and Bioengineering 131:434–441.https://doi.org/10.1016/j.jbiosc.2020.11.014
-
Myogenesis and muscle regenerationHistochemistry and Cell Biology 138:187–199.https://doi.org/10.1007/s00418-012-0972-x
-
A compartmentalized microfluidic neuromuscular co-culture system reveals spatial aspects of GDNF functionsJournal of Cell Science 128:1241–1252.https://doi.org/10.1242/jcs.167544
-
Channelrhodopsin-2 and optical control of excitable cellsNature Methods 3:785–792.https://doi.org/10.1038/nmeth936
-
Bioprinting of 3D in vitro skeletal muscle models: A reviewMaterials & Design 193:108794.https://doi.org/10.1016/j.matdes.2020.108794
Article and author information
Author details
Funding
Basque Government Department of Health (2021333020)
- Ainara Vallejo-Illarramendi
Diputación Foral de Gipuzkoa (Research and development)
- Jacobo Paredes Puente
Ministerio de Ciencia e Innovación (PID2020-119780RB-I00)
- Ainara Vallejo-Illarramendi
The funders had no role in study design, data collection and interpretation, or the decision to submit the work for publication.
Copyright
© 2022, Vesga-Castro et al.
This article is distributed under the terms of the Creative Commons Attribution License, which permits unrestricted use and redistribution provided that the original author and source are credited.
Metrics
-
- 6,795
- views
-
- 1,001
- downloads
-
- 32
- citations
Views, downloads and citations are aggregated across all versions of this paper published by eLife.
Citations by DOI
-
- 32
- citations for umbrella DOI https://doi.org/10.7554/eLife.77204