Impaired bone strength and bone microstructure in a novel early-onset osteoporotic rat model with a clinically relevant PLS3 mutation
Abstract
Plastin 3 (PLS3), a protein involved in formation of filamentous actin (F-actin) bundles, is important in human bone health. Recent studies identify PLS3 as a novel bone regulator and PLS3 mutations can lead to a rare monogenic early-onset osteoporosis. However, the mechanism of PLS3 mutation leading to osteoporosis is unknown, and its effective treatment strategies have not been established. Here, we have constructed a novel rat model with clinically relevant hemizygous E10-16del mutation in PLS3 (PLS3E10-16del/0) that recapitulates the osteoporotic phenotypes with obviously thinner cortical thickness, significant decreases in yield load, maximum load, and breaking load of femora at 3, 6, 9 months old compared to wild-type rats. Histomorphometric analysis indicates a significantly lower mineral apposition rate in PLS3E10-16del/0 rats. Treatment with alendronate (1.0 µg/kg/day) or teriparatide (40 µg/kg five times weekly) for 8 weeks significantly improves bone mass and bone microarchitecture, and bone strength is significantly increased after teriparatide treatment (p<0.05). Thus, our results indicate that PLS3 plays an important role in the regulation of bone microstructure and bone strength, and we provide a novel animal model for the study of X-linked early-onset osteoporosis. Alendronate and teriparatide treatment could be a potential treatment for early-onset osteoporosis induced by PLS3 mutation.
Editor's evaluation
The findings are significant with regard to the clinical treatment of early onset osteoporosis due to PLS3 mutations. The evidence which supports the conclusions of the manuscript is strong. This manuscript will be of notable relevance to the field of metabolic bone diseases.
https://doi.org/10.7554/eLife.80365.sa0Introduction
Osteoporosis is the most common metabolic bone disorder and is characterized by low bone mineral density (BMD), bone microarchitecture deterioration, and increased predisposition for bone fractures. Osteoporosis is usually regarded as an aging-related disease, however, idiopathic osteoporosis has also emerged in children and adolescents, which refers to significantly lower-than-expected bone mass manifesting in childhood with no identifiable etiology (Mäkitie and Zillikens, 2022). Recently, genetic variations have been found to be closely associated with diversities of BMD, fracture risk, and effects of anti-osteoporotic treatment (Ralston and Uitterlinden, 2010). A series of studies identify multiple heritable genetic variants that lead to early-onset osteoporosis (EOOP).
In 2013, a new kind of severe EOOP was first described, which was caused by mutations in PLS3 (van Dijk et al., 2013). PLS3 (OMIM 300131) has 16 exons and spans approximately 90 kb, which is located on Xq23 and encodes a highly conserved protein plastin 3 (PLS3). PLS3 belongs to a family of actin-binding proteins, which is ubiquitously expressed in solid tissues and involved in the binding and bundling of actin filaments in the cytoskeleton, thus partaking in various cellular functions, such as cell migration and adhesion (Wolff et al., 2021). In bone, PLS3 is proposed to regulate cytoskeletal actin bundling, osteocyte function and their mechanosensory apparatus, osteoclast function, and bone matrix mineralization (Pathak et al., 2020; Wolff et al., 2021). A series of pedigree studies demonstrated that variants in PLS3 led to significantly reduced BMD and early-onset recurrent fragility fractures (Costantini et al., 2018; Fahiminiya et al., 2014; Hu et al., 2020; Kämpe et al., 2017; Kannu et al., 2017; Laine et al., 2015; Lv et al., 2017; van Dijk et al., 2013; Wang et al., 2020). Till now, 29 different mutations in PLS3 have been reported to induce EOOP (Brlek et al., 2021; Cohen et al., 2022; Wolff et al., 2021). Due to its X-chromosomal inheritance, PLS3-induced osteoporosis has more severe effects on males than females, although heterozygous carrier females also suffer from EOOP. However, the exact function of PLS3 in bone is still unknown.
So far, the animal models carrying patient-derived PLS3 mutations have not been generated, which are valuable to unveil the pathogenesis of this ultra-rare X-linked osteoporosis induced by PLS3 mutations. A previously reported PLS3 knock-out (KO) murine model displayed a significant decrease in cortical thickness (Ct.Th) with normal or decreased trabecular number. This model harbored a complete deletion of PLS3 gene that was different from mutations identified in patients (Neugebauer et al., 2018; Yorgan et al., 2020). Moreover, effective treatment strategies have not been established in EOOP related to PLS3 mutations. Few patients with PLS3-related EOOP received bisphosphonates or teriparatide (TPTD) treatment, while the efficacy was variable (Fratzl-Zelman et al., 2021; Hu et al., 2020; Lv et al., 2017; Välimäki et al., 2017; van Dijk et al., 2013). To explore the potential pathogenesis and treatment strategies of PLS3-related EOOP, we independently constructed a novel rat model with ubiquitous deletion of the exon 10–16 of PLS3 (PLS3E10-16del/0), which recapitulated a patient-specific mutation of exon 10–16 deletion in PLS3 (Lv et al., 2017). We observed that PLS3E10-16del/0 rats, similar to the respective patient, displayed impaired bone strength due to thinner cortical bone, which could be improved by treatment with alendronate (ALN) and TPTD. Compared to the previously reported mice model, the novel rat model had a milder bone phenotype possibly due to the presence of truncated PLS3 variants.
Results
The rats with hemizygous E10-16del mutation were successfully generated
A large fragment deletion of exon 10–16 in PLS3 was introduced into the genome of rats, and a 9626 bp deletion from 84,172 to 93,797 bp (NC_051356.1) was confirmed by genotyping and Sanger sequencing (Figure 1A and B). PLS3 antibody used in this study targeted the KO region of PLS3 protein (deleted amino acid region: 331–630). Therefore, using western blotting of equivalent amounts of total proteins from wild-type (WT) and PLS3E10-16del/0 rats, we observed a band of appropriate size for PLS3 (~70 kDa) in membranes of WT rats, which was lacking in membranes of PLS3E10-16del/0 rats (Figure 1C). Immunohistochemical staining of femoral sections also unraveled that PLS3 was present in osteocytes, osteoblasts, and osteoclasts in cortical and trabecular bone of WT rats, while PLS3 was not seen in bone cells of the PLS3E10-16del/0 rats (Figure 1E). Moreover, quantitative polymerase chain reaction (qPCR) results also indicated that the expression level of PLS3 E10-16 was extremely low in PLS3E10-16del/0 rats. However, we found a similar expression level of PLS3 E1-9 between PLS3E10-16del/0 and WT rats, which indicated a possible presence of truncated PLS3 variants (Figure 1D). Together, these results indicated that rats with hemizygous E10-16 deletion in PLS3 were successfully built.
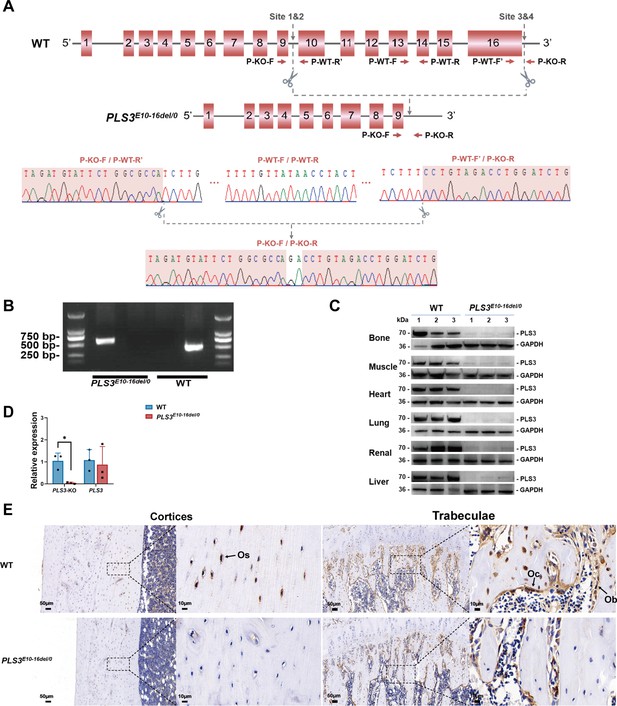
Rats with PLS3 E10-16del mutation built by CRISPR/Cas9 and confirmation.
(A) Schematic diagram of targeted PLS3 gene deletion using CRISPR/Cas9 and Sanger sequencing analysis. Four specific CRISPR target sites near the genomic region of exon 10–16 were designed. CRISPR/Cas9 systems specifically cleaved the target sites. Sanger sequence confirmed the genomic deletion of exon 10–16 (No. 84172–93797 bp) and two base pair insertions at the target site due to non-homologous end joining after the DNA cleavage. (B) Polymerase chain reaction (PCR) genotyping of PLS3E10-16del/0 rats. DNA from tail snips was subjected to PCR using P-KO-F/P-KO-R and P-WT-F/P-WT-R primers for mutant and WT allele, respectively. Amplification of mutant samples resulted in one copy of the upper 554 bp fragment, while WT samples yield one copy of the lower 450 bp fragment. (C) PLS3 gene deletion confirmed by western blot. PLS3 protein expression was absent in various tissues of the PLS3E10-16del/0 rats compared to age-matched WT rats, indicating the whole-body deletion of the PLS3 gene. (D) Quantitative PCR (qPCR) confirmation of PLS3 E10-16 deletion. The results of PLS3-KO indicated the expression level of PLS3 E10-16 (knockout region). The results of PLS3 indicated the expression level of PLS3 E1-9 (uneditable region). The expression level of PLS3 E10-16 was extremely low in PLS3E10-16del/0 rats while the expression level of PLS3 exon 1–9 was similar to WT rats. Data were pooled from three independent experiments and were presented as mean ± SEM. Data were analyzed using unpaired two-tailed Student t test. *p <0.05 vs WT groups. (E) Representative image of PLS3 immunohistochemistry in the femoral sections. Os, osteocytes; Ob, osteoblasts; Oc, osteoclasts. Immunohistochemical staining images revealed that PLS3 was present in osteocytes, osteoblasts, and osteoclasts in the cortical (left) and trabecular (right) bone of WT rats. Loss of staining in osteocytes, osteoblasts, and osteoclasts in the PLS3E10-16del/0 rats confirmed the successful deletion of the PLS3 gene in bone. WT: wild-type.
-
Figure 1—source data 1
Sanger sequencing confirmation of PLS3 E10-16 knockout.
- https://cdn.elifesciences.org/articles/80365/elife-80365-fig1-data1-v2.zip
-
Figure 1—source data 2
Western blotting analysis of PLS3 protein expression.
- https://cdn.elifesciences.org/articles/80365/elife-80365-fig1-data2-v2.zip
-
Figure 1—source data 3
Original data of quantitative polymerase chain reaction (qPCR) results.
- https://cdn.elifesciences.org/articles/80365/elife-80365-fig1-data3-v2.xlsx
Rats carrying mutant allele were born close to the expected Mendelian ratio (PLS3E10-16del/+: 28.6%, PLS3E10-16del/0: 28.5%, WT: 42.4%). The PLS3E10-16del/0 rats showed similar movement, locomotor activities, and longitudinal growth to WT rats (Figure 1—figure supplement 1). Moreover, the life span of WT and PLS3E10-16del/0 rats was similar and no rats died during the experimental period. There were also no statistical differences in body weight change between WT and PLS3E10-16del/0 rats during the whole experimental period (Figure 1—figure supplement 1).
The biomechanical properties of PLS3E10-16del/0 rats were significantly impaired. Compared to age- and gender-matched WT group, PLS3E10-16del/0 rats exhibited a decrease by 24.8% in yield load (p<0.001), 19.4% in maximum load (p<0.01), and 25.3% in breaking load (p<0.05) of femur of 3-month-old PLS3E10-16del/0 rats, which continued to 6 and 9 months old of mutant rats (Figure 2A and B). Notably, the stiffness (108.18±11.28 vs 501.20±84.97 N/mm, p<0.001) and breaking load (155.99±39.92 vs 227.75±27.06 N, p<0.01) of femur of 9-month-old PLS3E10-16del/0 rats were pronouncedly lower than those of WT rats. Significantly reduced maximum load of vertebrae was also found in PLS3E10-16del/0 rats (Figure 2C). Compared with WT rats, the average maximum load of the fifth lumbar vertebra decreased by 28.5% (p<0.01) and 43.6% (p<0.001) at 3 and 6 months old of PLS3E10-16del/0 rats. No significant difference was found in the stiffness, work-to-failure, and post-yield displacement between 3-month-old PLS3E10-16del/0 rats and WT rats (Figure 2A, Figure 2—figure supplement 1A).
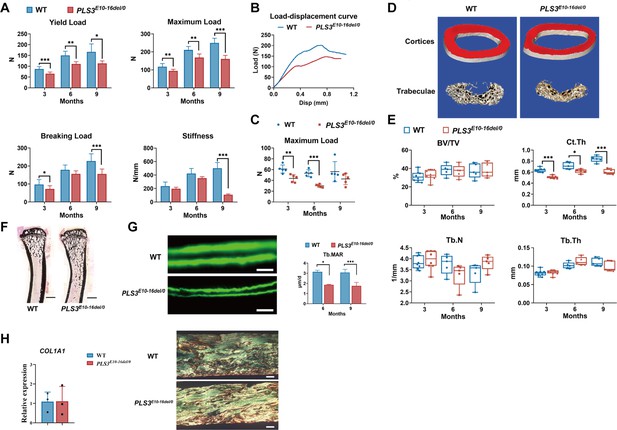
Bone strength, bone microstructure, and bone formation activity of PLS3E10-16del/0 rats.
(A) Mechanical three-point bending tests of femora from PLS3E10-16del/0 and WT rats (n=5-8 per group). (B) Typical load-displacement curves of PLS3E10-16del/0 and WT rats. (C) Indentation tests of L5 from PLS3E10-16del/0 and WT rats (n=5 per group). Data were analyzed using unpaired two-tailed Student t test. *p<0.05, **p <0.01, ***p <0.001 vs WT groups. (D) Three-dimensional reconstruction images of femurs from PLS3E10-16del/0 and WT rats. (E) Micro-CT assessment of the distal femurs from PLS3E10-16del/0 and WT rats (n=5-8 per group). BV/TV: bone volume/tissue volume, Ct.Th: cortical thickness, Tb.Th: trabecular thickness, Tb.N: trabecular number. (F) Representative von Kossa-stained sections of tibia diaphysis of PLS3E10-16del/0 and WT rats. Scale bar = 2000 μm. WT: wild-type. (G) Typical images of unstained and uncalcified vertebra of PLS3E10-16del/0 rats and comparison of mineral apposition rate (n=4 per group). Scale bar = 10 μm. Data were analyzed using unpaired two-tailed Student t test. *p<0.05 vs WT groups. Tb.MAR: mineral apposition rate of lumbar trabeculae. (H) Expression level of COL1A1 and photomicrographs of picrosirius red-stained sections of cortical bone regions of femur visualized through polarized light microscopy. Scale bar = 100 μm. Data were pooled from three independent experiments and were presented as mean ± SEM.
-
Figure 2—source data 1
Original data of the results of three-point binding tests.
- https://cdn.elifesciences.org/articles/80365/elife-80365-fig2-data1-v2.xlsx
-
Figure 2—source data 2
Original data of the results of indentation testing.
- https://cdn.elifesciences.org/articles/80365/elife-80365-fig2-data2-v2.xlsx
-
Figure 2—source data 3
Micro-computed tomography (μCT) analysis of the distal femur and histomorphometric evaluation of L4.
- https://cdn.elifesciences.org/articles/80365/elife-80365-fig2-data3-v2.xlsx
-
Figure 2—source data 4
Original data of histomorphometry.
- https://cdn.elifesciences.org/articles/80365/elife-80365-fig2-data4-v2.xlsx
The microstructure of cortical bone was deteriorated in PLS3E10-16del/0 rats. Femoral Ct.Th of 3-, 6-, 9-month-old PLS3E10-16del/0 rats were 79.9% (p<0.001), 86.3% (p<0.05), 72.6% (p<0.001) of age-matched WT rats, respectively. However, bone volume/tissue volume (BV/TV), bone surface area/bone volume (BS/BV), trabecular thickness (Tb.Th), trabecular number (Tb.N), and trabecular separation (Tb.Sp) in femur of PLS3E10-16del/0 rats were similar to WT rats at all ages (Figure 2D and E, Figure 2—figure supplement 1B). No significant differences were found in %Tb.Ar, Tb.Th, Tb.N, and Tb.Sp of lumbar vertebrae between the PLS3E10-16del/0 rats and WT rats (Figure 2—figure supplement 1C).
The mineral apposition rate (MAR) of trabecular bone (Tb.MAR) in lumbar vertebrae was significantly decreased in 6- and 9-month-old PLS3E10-16del/0 rats than WT rats (Figure 2G), while no statistical changes were detected in MAR of endocortical (Ec.MAR) and periosteal surface (Ps.MAR) of tibial cortex (Figure 2—figure supplement 1D). The number of osteocytes, osteoclasts, and osteoblasts in PLS3E10-16del/0 rats was similar to WT rats at all ages (Figure 2—figure supplement 1E). Also, PLS3E10-16del/0 and WT rats had similar Ocn-positive areas at the trabecular and cortical bone of femur (Figure 2—figure supplement 2A). Serum levels of total alkaline phosphatase (ALP), β-CTX, and calcium were similar in PLS3E10-16del/0 and WT rats (Figure 2—figure supplement 2C).
Since no difference was found in bone mass between WT and PLS3E10-16del/0 rats, we further investigated the quantity and structure of bone collagen. The expression of COL1A1 in tibia was also similar between two groups. However, compared to WT rats, the cortical bone was more porous and the collagen fibers of PLS3E10-16del/0 rats were relatively disorganized (Figure 2H).
No difference was found in the area of adipocytes in the distal marrow per tissue area. However, 3-month-old PLS3E10-16del/0 rats had more adipocytes than WT rats of the same age (Figure 2—figure supplement 2B). We also investigated the parameters of glucose and lipid metabolism and found similar levels of serum glucose (Glu), triglycerides (TG), cholesterol (TC), low-density lipoprotein (LDL) between PLS3E10-16del/0 rats and WT at all ages (Figure 2—figure supplement 2D). Besides, PLS3 mutations were reported to have patent ductus arteriosus (Qiu et al., 2022). However, no obvious abnormalities were found in ultrasonic cardiogram and hematoxylin and eosin (H&E) staining about the cardiac structure and function of PLS3E10-16del/0 rats.
Effects of anti-osteoporotic treatment on PLS3E10-16del/0 rats
ALN or TPTD treatment for 8 weeks significantly improved bone microstructure of PLS3E10-16del/0 rats. Compared to vehicle (VEH) group, Tb.N and BV/TV values were increased by 38.4% and 38.7% in ALN group and by 35.9% and 29.3% in TPTD group, while Tb.Sp was decreased by 49.3% and 40.1% in ALN and TPTD group, respectively (all p<0.05), and cancellous BMD of femur increased by 43.0% in ALN group (p<0.01) and 33.3% in TPTD group (p<0.01). ALN or TPTD treatment significantly increase 7.4% and 4.8% of Ct.Th of PLS3E10-16del/0 rats (all p<0.05 vs VEH group) (Figure 3A and B). A significant histomorphometric increase by 31.7% and 60.3% in %Tb.Ar and Tb.Th of L4 was observed in TPTD group (all p<0.05 vs VEH group), but not in ALN group (Figure 4A and B). Tibial Ec.MAR of TPTD group was higher than VEH group (p<0.01), and the lowest Ec.MAR (2.75 μm/day) in the tibia was found in rats of ALN group (Figure 4E). Ps.MAR of the tibia was similar among ALN, TPTD, and VEH group (Figure 4—figure supplement 1).
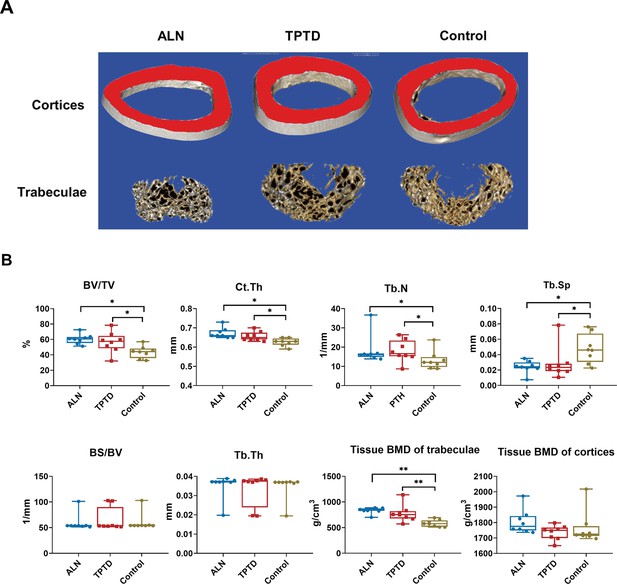
The efficacy of anti-osteoporotic treatment in PLS3E10-16del/0 rats.
(A) Three-dimensional reconstruction images of femurs after treatment. (B) Microstructural parameters of femurs by micro-CT after treatment (n=8 per group). ALN: alendronate, TPTD: teriparatide, BV/TV: bone volume/tissue volume, BS/BV: bone surface area/bone volume, Tb.Th: trabecular thickness, Tb.N: trabecular number, Tb.Sp: trabecular separation, Ct.Th: cortical thickness, BMD: tissue bone mineral density. Data were shown as the mean ± SD, evaluated by one-way ANOVA followed by Tukey’s post hoc test. *p<0.05; **p<0.01; ***p<0.001.
-
Figure 3—source data 1
Microstructural parameters of femurs measured by micro-CT after treatment.
- https://cdn.elifesciences.org/articles/80365/elife-80365-fig3-data1-v2.xlsx
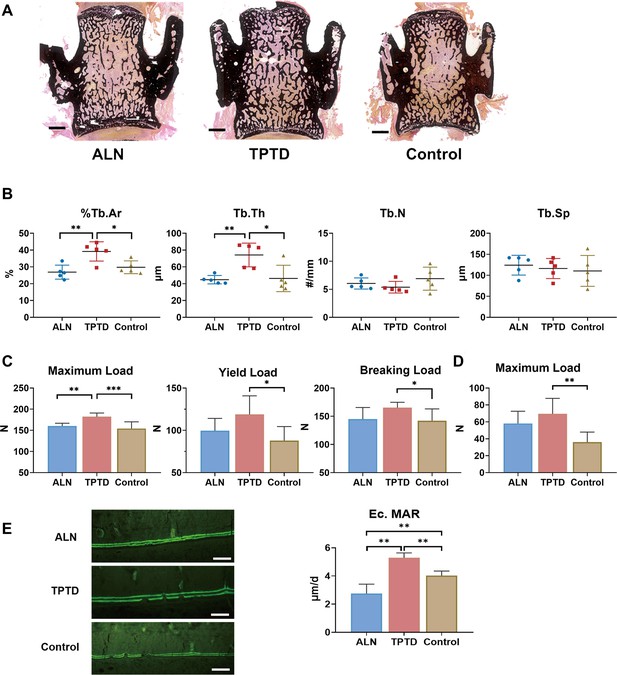
Changes in microarchitecture and strength after anti-osteoporotic treatment.
(A) Typical images of unstained and uncalcified vertebra of PLS3E10-16del/0 rats after treatment. Scale bar = 1000 μm. (B) Histomorphometric analysis of L4 after treatment (n=5 per group). %Tb.Ar: trabecular area, Tb.Th: trabecular thickness, Tb.N: trabecular number, Tb.Sp: trabecular separation. (C) Effects of treatment on the mechanical strength of femoral diaphysis (n=8 per group). The diaphysis was subjected to three-point bending test to failure, which provided data on yield load, maximum load, breaking load. (D) Effects of treatment on the mechanical strength of L5 (n=7-8 per group). The vertebral body was subjected to indentation test to acquire maximum load. (E) Comparison of Ec.MAR in the tibial cortex among three treatment groups (n=5 per group). Scale bar = 100 μm. ALN: alendronate, TPTD: teriparatide, control: saline. Ec.MAR: mineral apposition rate of endocortical surface of tibia cortex. Data were shown as the mean ± SD, evaluated by one-way ANOVA followed by Tukey’s post hoc test. *p<0.05; **p<0.01; ***p<0.001.
-
Figure 4—source data 1
Changes in strength after anti-osteoporotic treatment.
- https://cdn.elifesciences.org/articles/80365/elife-80365-fig4-data1-v2.xlsx
Moreover, TPTD treatment significantly increased maximum load (182.2±8.7 N vs 154.2±15.9 N, p<0.001), yield load (117.5±22.8 N vs 87.8±16.6 N, p<0.05), and breaking load (165.3±9.5 N vs 142.2±20.8 N, p<0.01) of femur and maximum load of the fifth lumbar vertebrae (69.5±18.3 N vs 32.1±5.1 N, p<0.01) than VEH group (Figure 4C and D). The bone strength of femur and lumbar vertebrae was not significantly improved in ALN group.
Discussion
Recent advancements in genetic research have uncovered that loss-of-function variants in PLS3 can cause a monogenic X-linked EOOP and osteoporotic fractures, but the exact mechanism is unknown (Wolff et al., 2021), and its optimal treatment regimen has not been established. In the present study, we have generated a novel rat model with large fragment deletion in PLS3, and we systematically assessed bone microarchitecture, bone biomechanical property, BMD, and bone remodeling for the first time in this novel rat model with patient-derived PLS3 mutation. Interestingly, the newly generated PLS3E10-16del/0 rat model displayed significantly impaired bone strength, decreased Ct.Th, and decreased MAR of trabecular bone. We demonstrated that ALN or TPTD could significantly improve bone microstructure and increase BMD, and TPTD could obviously improve the bone strength of PLS3E10-16del/0 rat.
In this study, PLS3E10-16del/0 rats displayed a bone-specific phenotype, including significantly impaired bone strength, decreased cortical bone thickness, and decreased MAR, despite the ubiquitous presence of the mutation. Similar results were found in patients with various PLS3 mutations and the PLS3-deficient mice model (Costantini et al., 2018; Fahiminiya et al., 2014; Hu et al., 2020; Kämpe et al., 2017; Kannu et al., 2017; Laine et al., 2015; Lv et al., 2017; Neugebauer et al., 2018; van Dijk et al., 2013; Wang et al., 2020; Yorgan et al., 2020), which demonstrated that PLS3 had indispensable roles in bone metabolism. Interestingly, although we observed no change in bone mass in PLS3E10-16del/0 rats, impaired bone strength was a prominent feature of this novel rat model, which could be attributed to the following factors. First, cortical wall thickness was significantly decreased in the PLS3E10-16del/0 rats, which was a fundamental structural determinant of bone strength. Second, higher porosity in cortical bone and disorganized collagen fibers in bone played important roles in impairment of bone strength (Gastaldi et al., 2020).
PLS3 is expressed in all solid tissues except hematopoietic cells and is involved in all the processes dependent on filamentous actin (F-actin) dynamics. In bone, we also verified that PLS3 was widely expressed in osteoblasts, osteoclasts, and osteocytes (Fahiminiya et al., 2014; Kamioka et al., 2004; Neugebauer et al., 2018). Bone histomorphometric analysis indicated that the quantities of bone cells were normal in PLS3E10-16del/0 rats, which was consistent with previous reports (Neugebauer et al., 2018; Yorgan et al., 2020). However, dysfunction of bone cells in PLS3 mutant animal models and patients was found in the current and previous studies. Animal studies indicated PLS3 involvement in cytoskeletal actin bundling (Oprea et al., 2008), thus mediating mechanotransduction in osteocytes and further affecting cellular signal transduction between osteoblasts and osteoclasts, though the regulation was not confirmed (Pathak et al., 2020; van Dijk et al., 2013). Studies on patients’ bone biopsies collectively insinuated a role for PLS3 in bone matrix mineralization (Balasubramanian et al., 2018; Kämpe et al., 2017; Kannu et al., 2017; Laine et al., 2015). Impaired bone mineralization induced by PLS3 mutation was also observed in cell experiments and a murine model (Fahiminiya et al., 2014; Yorgan et al., 2020). F-actin-bundling ability or Ca2+ sensitivity would be disturbed after PLS3 mutations (Schwebach et al., 2020), which would affect intracellular calcium concentrations that was needed during osteoblasts differentiation and bone formation (Wang et al., 2018). In PLS3E10-16del/0 rats, though immunohistochemical staining showed no significant changes in the expressions of Ocn, which was a late osteoblastic differentiation marker but did not represent a degree of bone mineralization (Moriishi et al., 2020), we found an obvious reduction in MAR of trabecular bone, indicating PLS3 was more likely involved in regulating bone mineralization. More recently, experimental findings suggested the regulatory function of PLS3 in osteoclastogenesis and osteoclast function through influencing podosome organization (Neugebauer et al., 2018). Taken together, these observations indicated that dysfunctional F-actin dynamics caused by PLS3 mutation would lead to abnormal bone metabolism. However, the exact roles of PLS3 regulating bone cells still needed to be further elucidated. Compared to the previously reported mice model with an entire deletion of PLS3 (Neugebauer et al., 2018; Yorgan et al., 2020), PLS3E10-16del/0 rats had a milder bone phenotype possibly due to the presence of truncated PLS3. PLS3 is comprised of an N-terminal Ca2+-binding regulatory domain (RD) followed by a core consisting of two actin-binding domains. F-actin bundling by PLS3 is tightly regulated by Ca2+ binding to RD (Schwebach et al., 2020). Deletion of PLS3 E10-16 might result in a truncated protein with the RD retained as we detected the presence of PLS3 E1-9 cDNA. However, further studies are needed to verify the findings and explore the functional roles of different domains in bone regulation.
Recently, studies indicated that patients with PLS3 mutations had significantly elevated serum DKK1 concentrations than patients with WNT1 mutations, which indicated impaired WNT signaling may involve in the occurrence of PLS3-related osteoporosis (Mäkitie et al., 2020a). PLS3-deficient murine model exhibited decreased expression of WNT16 (Yorgan et al., 2020). WNT16-deficient mice displayed cortical bone defects with normal trabecular bone, with unchanged cortical MAR (Movérare-Skrtic et al., 2014). Both PLS3 and WNT16 could inhibit osteoclasts formation by inhibiting NF-κB activation and Nfatc1 expression (Movérare-Skrtic et al., 2014; Neugebauer et al., 2018). Therefore, PLS3 mutation could lead to increased osteoclasts differentiation through decreased PLS3 and WNT16 expression, which could lead to thin cortical bone. However, serum bone turnover biomarker levels of ALP and β-CTX were normal in this PLS3E10-16del/0 rats and patients with PLS3 mutations (Balasubramanian et al., 2018; Fahiminiya et al., 2014; Kämpe et al., 2017; Laine et al., 2015), so more in-depth research on pathogenesis of PLS3-related osteoporosis was needed in the future.
Since patients with PLS3 mutation presented with low BMD, multiple peripheral fractures, and vertebral compression fractures (Hu et al., 2020; Lv et al., 2017; Mäkitie et al., 2020b; van Dijk et al., 2013), it is of great clinical significance to establish an effective treatment regimen for PLS3-related osteoporosis. Bisphosphonates are still recommended as the first-line antiresorptive therapy for osteoporosis. TPTD, a recombinant fragment of human parathyroid hormone (1–34), is an extensively used bone anabolic drug for osteoporosis. It is noticed that the action mechanisms are completely different between antiresorptive and osteoanabolic agents. ALN inhibits osteoclast activity and bone resorption mainly via the mevalonate pathway (Cremers et al., 2019). Moreover, TPTD stimulates bone formation by affecting protein kinases, MAP-kinase, phospholipases, as well as the WNT signaling pathway (Canalis et al., 2007). In this study, ALN and TPTD were all effective in increasing BMD and improving bone microstructure of PLS3E10-16del/0 rats, which were consistent with their efficacy in patients with PLS3-related EOOP (Fratzl-Zelman et al., 2021; Hu et al., 2020; Lv et al., 2017; Välimäki et al., 2017; van Dijk et al., 2013). Interestingly, TPTD treatment obviously improved the bone mechanical strength of femora and vertebrae of PLS3E10-16del/0 rats, consistent with the improvement of bone microstructure of the above sites. However, we did not observe that ALN significantly improved bone biomechanical properties of PLS3E10-16del/0 rats, which may be related to the small therapeutic dose and the short treatment time in this study. In another study, treatment with ALN at a dose of 30 µg/kg/day for 12 weeks significantly improved stiffness of a murine model of osteogenesis imperfecta (McCarthy et al., 2002). It is necessary to carry out studies with a larger dose and longer time treatment of ALN to clarify its effects on bone strength of PLS3E10-16del/0 rats.
Although the precise molecular mechanisms of PLS3 mutation inducing EOOP needed to be clarified, we confirmed that PLS3 played critical roles in regulating bone metabolism and maintaining the integrity of bone structure and mechanical properties in this novel PLS3E10-16del/0 rat model. Our results demonstrated for the first time that TPTD and ALN were effective to PLS3E10-16del/0 rats, which provided valuable experimental evidence for the treatment of PLS3-related EOOP. However, there were a few limitations. Since PLS3 was widely expressed, we did not conduct enough morphometrical and functional analyses of other tissues and organs of PLS3E10-16del/0 rats. We did not conduct in-depth research on the signal pathways regulating bone metabolism, such as WNT/β-catenin, OPG-RANK-RANKL pathway, and so on in PLS3E10-16del/0 rats, which were helpful to clarify the pathogenesis of this EOOP.
In conclusion, PLS3 plays major roles in bone metabolism and bone integrity. Impaired bone microstructure and bone strength were prominent characteristics of rats with hemizygous E10-16del mutation of PLS3, though the exact pathogenesis still needs further study. ALN and TPTD treatment can increase BMD and improve the bone microstructure of rats with PLS3-related EOOP.
Materials and methods
Generation of PLS3 KO rat model and genetic identification
Request a detailed protocolGeneral PLS3 KO rat model was generated using the CRISPR/Cas9 system at the Institute of Laboratory Animal Sciences, Chinese Academy of Medical Sciences & Peking Union Medical College. Five specific guide RNAs were used to target the exons 10–16 of PLS3 (Table 1), which were annealed and cloned into the PUC57-gRNA expression vector (Addgene 51132, Cambridge, MA, USA) with a T7 promoter. In vitro, transcription of gRNA template was accomplished using the MEGAshortscript Kit (AM1354, Ambion). The pST1374-NLS-flag-linker-Cas9 vector (Addgene 44758) was linearized using the Age I enzyme and transcribed with a T7 Ultra Kit (Ambion, AM1345). After purified with the MEGAclear Kit (AM1908, Ambion), a mixture of transcribed Cas9 mRNA and gRNA was microinjected into the cytoplasm of zygotes of Sprague Dawley (SD) rats which were obtained commercially from Beijing Vital River Laboratory Animal Technology Co., Ltd. A male founder was backcrossed with WT rats to generate female heterozygous KO rats (PLS3E10-16del/+), which were subsequently crossed with WT male rats. As being located on the X chromosome, PLS3-induced osteoporosis was more severe in males than females, male hemizygous KO rats (PLS3 E10-16del/0) of the offspring were selected to be further studied. Since PLS3-related osteoporosis had its disease onset early in childhood and adolescence, and rats reached the peak bone mass at 6–9 months of age, we chose rats at age of 3, 6, and 9 months for further investigation. All rats were registered and preserved on an SD genetic background in a specific pathogen-free environment without surpassing six animals in ventilated cages. Rats were provided with standard chow and water ad libitum.
Small guide RNA (gRNA) sequence for PLS3 gene knockout.
No. | Targeting site | Name | Sequence (5’–3’) |
---|---|---|---|
1 | ATGTATTCTGGCGCCATCT | PLS3-gRNA-UP1 | TAGGatgtattctggcgccatct |
PLS3-gRNA-DOWN1 | aaacAGATGGCGCCAGAATACAT | ||
2 | TACATACATACATAGATGA | PLS3-gRNA-UP2 | TAGGtacatacatacatagatga |
PLS3-gRNA-DOWN2 | aaacTCATCTATGTATGTATGTA | ||
3 | TCTCAGGTGAAGTGCACA | PLS3-gRNA-UP3 | TAGGtctcaggtgaagtgcaca |
PLS3-gRNA-down3 | aaacTGTGCACTTCACCTGAGA | ||
PLS3-gRNA-UP4 | TAGGATCCAGGTCTACAGGAAAG | ||
PLS3-gRNA-down4 | aaacctttcctgtagacctggat | ||
4 | CTTTCCTGTAGACCTGGAT | PLS3-gRNA-UP5 | TAGGATCCAGGTCTACAGGAAAG |
PLS3-gRNA-down5 | AAACCTTTCCTGTAGACCTGGAT |
-
The gRNAs were designed based on Rattus norvegicus (Norway rat) genome assembly Rnor_6.0 (rn6), using CRISPR Design Tool (http://tools.genome-engineering.org).
-
Gene ID: 81748; Location: Chromosome X - NC_005120.4.
-
The words in red represented the protospacer adjacent motif (PAM) sequences (5’–3’).
Genomic DNA was isolated from tail snips using E.Z.N.A. Tissue DNA Kit (Omega Bio-tek, Norcross, GA, USA). Genotyping was performed using PCR amplification. The allele-specific primers were listed in Table 2. Primers P-KO-F/P-KO-R were used to amplify PLS3 KO allele, and a 554 bp PCR production would be generated. Primers P-WT-F/P-WT-R were used to amplify WT allele, and a 450 bp PCR production would be generated. Thermal cycling conditions consisted of an initial denaturation at 95°C for 3 min, followed by 38 cycles at 95°C for 30 s, 52–58°C for 30 s, and 72°C for 1 min. Sanger sequencing of PCR products was further completed.
Primers used for Sanger sequencing, genotyping, and qPCR.
Primer name | Sequence (5’–3’) | GenBank accession number | Nucleotide position |
---|---|---|---|
P-WT-F | CCCATAAGTTGTTCCTTGATTTCC | NC_051356.1 | 88350–88373 |
P-WT-R | CACTGCCTGAATAAGACCCACTC | NC_051356.1 | 88777–88799 |
P-WT-F’ | CCTTGTGGAAGTAAAACCGAA | NC_051356.1 | 93529–93549 |
P-WT-R’ | TACAAAGGCCAAGTTCAG | NC_051356.1 | 84492–84510 |
P-KO-F | CTTCATTCCCTTTGCACGTT | NC_051356.1 | 84092–84111 |
P-KO-R | TACAAAGGCCAAGTTCAG | NC_051356.1 | 94242–94262 |
PLS3-KO-F | AAATTCTCCTTGGTTGGCATT | NM_031084.1 | 1507–1527 |
PLS3-KO-R | TCCAGCTTCACTCAATGTTCC | NM_031084.1 | 1672–1692 |
PLS3-F | GAAAATGATCCCGATTGCAG | NM_031084.1 | 496–515 |
PLS3-R | CTCTCATCAATTGTATCGGGAA | NM_031084.1 | 605–626 |
COL1A1-F | TCCTGACGCATGGCCAAGAA | NM_053304.1 | 164–183 |
COL1A1-R | CATAGCACGCCATCGCACAC | NM_053304.1 | 289–308 |
GAPDH-F | TTCAACGGCACAGTCAAGG | NM_017008.4 | 235–253 |
GAPDH-R | CTCAGCACCAGCATCACC | NM_017008.4 | 331–348 |
-
Primers PLS3-KO-F/PLS3-KO-R were selected to amplify a fragment spanning from exon 13 to exon 14 of the rat PLS3 cDNA. The qPCR results were used to indicate the expression level of PLS3 E10-16 (knockout region). Primers PLS3-F/PLS3-R were selected to amplify a fragment spanning from exon 5 to exon 6 of the rat PLS3 cDNA. The qPCR results were used to indicate the expression level of PLS3 E1-9 (uneditable region).
-
F: forward, R: reverse, WT: wild-type, KO: knockout.
Western blot analysis
Request a detailed protocolIn order to confirm the general deletion of PLS3 protein, proteins from bone, muscle, heart, lung, renal, and liver of PLS3E10-16del/0 and WT rats were extracted for western blot. Briefly, the tissues were separately homogenized in RIPA buffer containing 1 mM PMSF and Halt Protease and Phosphatase Inhibitor cocktail (Thermo Fisher Scientific, Waltham, MA, USA). The protein concentration was determined with BCA Protein Assay Kit (Thermo Fisher Scientific). Protein samples (30 µg each) were loaded onto a 4–12% gradient SDS-PAGE gel and then transferred to PVDF membranes. After being blocked with 5% BSA, the membranes were incubated with primary antibody against PLS3 (Cat# ab233104, Abcam, 1:1000 dilution) or GAPDH (Cat# ab8245, Abcam, 1:1000 dilution) and washed with TBST three times, which were then incubated with a horseradish peroxidase-conjugated anti-rabbit or anti-mouse secondary antibody. Finally, the immunoblots were visualized by enhanced chemiluminescence.
Real-time qPCR
Request a detailed protocolTotal RNA was extracted from the tibia of WT and PLS3E10-16del/0 rats with TRIzol reagent and was reversely transcribed to cDNA using the PrimeScript RT Reagent Kit (Takara, Kusatsu, Japan). The gene expression levels were quantified by qPCR using TB Green Premix Ex Taq II (Tli RNase H Plus, Takara) on a Viia 7 Real-Time PCR System (Life Technologies, USA). Primer sequences for RT-qPCR were listed in Table 2. Relative mRNA expression levels were calculated using the 2−ΔΔCT method and normalized to the internal control GAPDH.
Treatment
Request a detailed protocolA total of 24 PLS3E10-16del/0 male rats at 3 months of age were randomized either to VEH, ALN, or TPTD therapy (n=8 in each group) for 8 weeks. ALN (Merck and Co., Inc, Rahway, NJ, USA) 1.0 µg/kg body weight was subcutaneously injected daily into rats in ALN group (Diab et al., 2011; Iwata et al., 2006). In the TPTD group, the agent (recombinant human parathyroid hormone 1–34, provided by Salubris Biotherapeutics, Inc, Shenzhen, China) was subcutaneously injected at the dose of 40 µg/kg body weight, five times weekly (Komrakova et al., 2010; Lane et al., 1996). Rats in the VEH group received 0.9% saline and acted as control groups.
All animal experiments were approved by the Institutional Animal Care and Use Committee of the Peking Union Medical College Hospital (XHDW-2021-027).
Bone microstructure assessment
Request a detailed protocolThe left femur was fixed in 4% paraformaldehyde, of which microarchitecture was assessed by micro-computed tomography (μCT) (Inveon MM CT, Siemens, Erlangen, Germany) according to the recommended protocol (Bouxsein et al., 2010). In vitro scans were operated with an X-ray tube voltage of 60 kV, a current of 400 μA, an exposure time of 800 ms, and a voxel size of 20 μm. The region of interest for trabecular bone was drawn in the distal epiphysis, starting 1.5 mm below the growth plate and extending 100 slices to proximal end. Cortical bone was analyzed in a 1000-μm-long volume situated in the middle of the diaphysis. BMD, BV/TV, BS/BV, Ct.Th, Tb.Th, Tb.N, and Tb.Sp were measured. The Inveon Research Workplace software (Siemens) was used for reconstruction and analysis of two-dimensional (2D) and 3D image.
Assessment of biomechanical properties of bone
Request a detailed protocolThe right femur and the fifth lumbar vertebrae (L5) were wrapped in saline-soaked gauze and stored at –20°C, which were thawed at room temperature 2 hr before the mechanical test. Three-point binding tests and indentation testing were implemented on a fatigue-testing machine (BOSE ElectroForce 3200, TA Instruments, New Castle, DE, USA). From the load-displacement curves of femurs, stiffness, yield load, maximum load, breaking load, post-yield displacement, and work-to-fracture were generated. Force‐displacement measurement was also performed on the fifth lumbar vertebral bodies, and maximum load was measured.
Measurement of bone metabolic markers and metabolic parameters
Request a detailed protocolUnder abdominal anesthesia, blood samples were collected via cardiac puncture. Concentrations of serum calcium (Ca), phosphorus (P), and ALP (a bone formation marker) were measured by an automated chemistry analyzer (AU5800, Beckman Coulter Inc, Brea, CA, USA). Serum level of C-telopeptide of type Ⅰ collagen (β-CTX, bone resorption marker) was measured by ELISA (Cat# CSB-E12776r, Cusabio Biotech Co., Wuhan, China). To determine whether PLS3 KO affected other metabolism, we measured the levels of serum Glu, TC, LDL, and TG using the automated chemistry analyzer.
Analysis of histology and histomorphometry
Request a detailed protocolAll rats received intraperitoneal injection of calcein (10 mg/kg body mass, Sigma-Aldrich, Co., St. Louis, MO, USA) for histomorphometric analysis on the second and the sixth day before euthanization. The left femur was decalcified after μCT scanning and were embedded in paraffin and cut into 4 μm sections using a microtome (Leica RM2016, Leica Microsystems). H&E staining and tartrate-resistant acid phosphatase staining (Servicebio, Cat# G1050) were performed. The osteoclast number per bone perimeter (N.Oc/B.Pm), osteocyte number per bone area (N.Ot/B.Ar), and osteoblast number/bone perimeter (N.Ob/B.Pm), number and area of adipocytes in the distal marrow per tissue area were calculated. Bone slices were also subjected to the picrosirius red staining for the evaluation of the organization of collagen fibers through polarized light microscopy (Axio Imager D2, Zeiss, Germany).
The right tibia and the fourth vertebrae (L4) were fixed in 70% alcohol and embedded in modified methyl methacrylate without decalcification. The embedded samples were cut into 10 μm thick sections, which were de-plasticized and stained with von Kossa stain kit (Servicebio, Cat# G1043) to calculate trabecular area (%Tb.Ar), Tb.Th, Tb.N, and Tb.Sp. For unstained slices, the MAR was calculated by dividing the distance between the two calcein labels by the inter-labeling period. Analysis was performed with ImageJ software according to the recommendation of ASBMR (Dempster et al., 2013).
Immunohistochemical staining
Request a detailed protocolParaffin-embedded femur samples were subjected to immunostaining for PLS3 and osteocalcin (Ocn). Slides were subjected to 0.05% trypsin at 37°C for 30 min for antigen retrieval and blocked in 3% hydrogen peroxide and 3% bovine serum albumin. Rabbit anti-PLS3 antibody (Cat# ab23310, Abcam, 1:200 dilution) or anti-Ocn antibody (Cat# ab93876, Abcam, 1:200 dilution) were applied, followed by incubation with HRP-labeled secondary antibody (ZSGB-BIO). PLS3 and Ocn expression were visualized using 3,3'-diaminobenzidine staining. Counterstaining was performed using hematoxylin. Ocn-positive area was quantified using ImageJ software.
Statistical analysis
Request a detailed protocolAll experiments were repeated at least thrice independently. Results were reported as means ± SD (standard difference). Comparisons of parameters between transgenic and WT rats were completed using Student’s t test. Parameters of rats at different ages or in different treatment groups were compared with one-way ANOVA followed by Tukey’s post hoc test. Statistical analysis was performed using SPSS Statistics 26.0 (IBM, Armonk, NY, USA), GraphPad Prism 8 (Statcon). Statistical significance was determined when p values equal to or less than 0.05.
Data availability
All data analyzed during this study are included in the manuscript and supporting file. Source Data files have been provided for Figures 1-4.
References
-
Novel PLS3 variants in X‐linked osteoporosis: exploring bone material propertiesAmerican Journal of Medical Genetics Part A 176:1578–1586.https://doi.org/10.1002/ajmg.a.38830
-
Guidelines for assessment of bone microstructure in rodents using micro-computed tomographyJournal of Bone and Mineral Research 25:1468–1486.https://doi.org/10.1002/jbmr.141
-
Mechanisms of anabolic therapies for osteoporosisThe New England Journal of Medicine 357:905–916.https://doi.org/10.1056/NEJMra067395
-
A novel frameshift deletion in PLS3 causing severe primary osteoporosisJournal of Human Genetics 63:923–926.https://doi.org/10.1038/s10038-018-0472-5
-
Pharmacology of bisphosphonatesBritish Journal of Clinical Pharmacology 85:1052–1062.https://doi.org/10.1111/bcp.13867
-
Effects of the combination treatment of raloxifene and alendronate on the biomechanical properties of vertebral boneJournal of Bone and Mineral Research 26:270–276.https://doi.org/10.1002/jbmr.197
-
Osteoporosis caused by mutations in PLS3: clinical and bone tissue characteristicsJournal of Bone and Mineral Research 29:1805–1814.https://doi.org/10.1002/jbmr.2208
-
An experimental procedure to perform mechanical characterization of small-sized bone specimens from thin femoral cortical wallJournal of the Mechanical Behavior of Biomedical Materials 112:104046.https://doi.org/10.1016/j.jmbbm.2020.104046
-
A novel mutation in PLS3 causes extremely rare X-linked osteogenesis imperfectaMolecular Genetics & Genomic Medicine 8:e1525.https://doi.org/10.1002/mgg3.1525
-
Terminal differentiation of osteoblasts to osteocytes is accompanied by dramatic changes in the distribution of actin-binding proteinsJournal of Bone and Mineral Research 19:471–478.https://doi.org/10.1359/JBMR.040128
-
PLS3 deletions lead to severe spinal osteoporosis and disturbed bone matrix mineralizationJournal of Bone and Mineral Research 32:2394–2404.https://doi.org/10.1002/jbmr.3233
-
PLS3 mutations in X-linked osteoporosis: clinical and bone characteristics of two novel mutationsHormone Research in Paediatrics 88:298–304.https://doi.org/10.1159/000477242
-
A novel splice mutation in pls3 causes X-linked early onset low-turnover osteoporosisJournal of Bone and Mineral Research 30:510–518.https://doi.org/10.1002/jbmr.2355
-
Bone-selective analogs of human PTH (1-34) increase bone formation in an ovariectomized rat modelJournal of Bone and Mineral Research 11:614–625.https://doi.org/10.1002/jbmr.5650110509
-
Biomarkers in Wnt1 and PLS3 osteoporosis: altered concentrations of DKK1 and FGF23Journal of Bone and Mineral Research 35:901–912.https://doi.org/10.1002/jbmr.3959
-
PLS3 mutations cause severe age and sex-related spinal pathologyFrontiers in Endocrinology 11:393.https://doi.org/10.3389/fendo.2020.00393
-
Early-Onset osteoporosisCalcified Tissue International 110:546–561.https://doi.org/10.1007/s00223-021-00885-6
-
Plastin 3 influences bone homeostasis through regulation of osteoclast activityHuman Molecular Genetics 27:4249–4262.https://doi.org/10.1093/hmg/ddy318
-
The osteocyte as the new discovery of therapeutic options in rare bone diseasesFrontiers in Endocrinology 11:405.https://doi.org/10.3389/fendo.2020.00405
-
Teriparatide treatment in patients with Wnt1 or PLS3 mutation-related early-onset osteoporosis: a pilot studyThe Journal of Clinical Endocrinology and Metabolism 102:535–544.https://doi.org/10.1210/jc.2016-2423
-
PLS3 mutations in X-linked osteoporosis with fracturesThe New England Journal of Medicine 369:1529–1536.https://doi.org/10.1056/NEJMoa1308223
-
A novel nonsense variant in pls3 causes X-linked osteoporosis in a Chinese familyAnnals of Human Genetics 84:92–96.https://doi.org/10.1111/ahg.12344
-
Plastin 3 in health and disease: a matter of balanceCellular and Molecular Life Sciences 78:5275–5301.https://doi.org/10.1007/s00018-021-03843-5
Article and author information
Author details
Funding
National Key Research and Development Program of China (2018YFA0800801)
- Mei Li
Chinese Academy of Medical Sciences Initiative for Innovative Medicine (2021-I2M-C&T-B-007)
- Mei Li
National Natural Science Foundation of China (No.81873668)
- Mei Li
Beijing Natural Science Foundation (7202153)
- Mei Li
Fundamental Research Funds for the Central Universities (3332022102)
- Jing Hu
National Key Research and Development Program of China (2021YFC2501704)
- Mei Li
Chinese Academy of Medical Sciences Initiative for Innovative Medicine (2021-I2M-1-051)
- Mei Li
National Natural Science Foundation of China (82070908)
- Mei Li
The funders had no role in study design, data collection and interpretation, or the decision to submit the work for publication.
Acknowledgements
This work is supported by National Key R&D Program of China (2018YFA0800801, 2021YFC2501704), CAMS Innovation Fund for Medical Sciences (CIFMS) (2021-I2M-C&T-B-007, 2021-I2M-1-051), National Natural Science Foundation of China (No. 81873668, 82070908), Beijing Natural Science Foundation (7202153), and the Fundamental Research Funds for the Central Universities (3332022102).
Ethics
This study was performed in strict accordance with the recommendations in the Guide for the Care and Use of Laboratory Animals of the National Institutes of Health. All animal experiments were approved by the Institutional Animal Care and Use Committee of the Peking Union Medical College Hospital (XHDW-2021-027). Every effort was made to minimize pain and suffering by providing support when necessary and choosing ethical endpoints.
Copyright
© 2023, Hu et al.
This article is distributed under the terms of the Creative Commons Attribution License, which permits unrestricted use and redistribution provided that the original author and source are credited.
Metrics
-
- 481
- views
-
- 94
- downloads
-
- 5
- citations
Views, downloads and citations are aggregated across all versions of this paper published by eLife.
Citations by DOI
-
- 5
- citations for umbrella DOI https://doi.org/10.7554/eLife.80365