Mechanistic insights into robust cardiac IKs potassium channel activation by aromatic polyunsaturated fatty acid analogues
Abstract
Voltage-gated potassium (KV) channels are important regulators of cellular excitability and control action potential repolarization in the heart and brain. KV channel mutations lead to disordered cellular excitability. Loss-of-function mutations, for example, result in membrane hyperexcitability, a characteristic of epilepsy and cardiac arrhythmias. Interventions intended to restore KV channel function have strong therapeutic potential in such disorders. Polyunsaturated fatty acids (PUFAs) and PUFA analogues comprise a class of KV channel activators with potential applications in the treatment of arrhythmogenic disorders such as long QT syndrome (LQTS). LQTS is caused by a loss-of-function of the cardiac IKs channel – a tetrameric potassium channel complex formed by KV7.1 and associated KCNE1 protein subunits. We have discovered a set of aromatic PUFA analogues that produce robust activation of the cardiac IKs channel, and a unique feature of these PUFA analogues is an aromatic, tyrosine head group. We determine the mechanisms through which tyrosine PUFA analogues exert strong activating effects on the IKs channel by generating modified aromatic head groups designed to probe cation–pi interactions, hydrogen bonding, and ionic interactions. We found that tyrosine PUFA analogues do not activate the IKs channel through cation–pi interactions, but instead do so through a combination of hydrogen bonding and ionic interactions.
Editor's evaluation
This work reports important findings regarding the regulation of ion channels by polyunsaturated fatty acids (PUFAs) through the identification of novel aromatic PUFA analogs with potent effects on the IKs channels, which allow for mechanistic insights into their mode of action. The experiments are solid, combining site-directed mutagenesis, electrophysiological and pharmacological approaches to dissect the different molecular mechanisms and sites involved in the functional interactions. This work will be of broad interest to ion channel biophysicists, physiologists, and medical chemists interested in drug development for LQT syndrome.
https://doi.org/10.7554/eLife.85773.sa0Introduction
The delayed rectifier potassium channel (IKs) underlies a critical repolarizing current that determines the timing of the ventricular action potential (Nerbonne and Kass, 2005). The cardiac IKs current is mediated by the association of the voltage-gated K+ channel KV7.1 α-subunit with the KCNE1 β-subunit (Abramochkin et al., 2018; Wang et al., 1998; Barhanin et al., 1996). The KV7.1 α-subunit consists of six transmembrane spanning segments, denoted S1–S6 where S1–S4 form the voltage-sensing domain (VSD) and S5–S6 form the pore domain (PD) (Sun and MacKinnon, 2017). The S4 segment contains several positively charged arginine residues that allow S4 to move outward, toward the extracellular side of the membrane, when the membrane becomes depolarized (Broomand et al., 2003). This outward movement of the S4 is transformed into pore opening as a result of conformational changes in the S4–S5 linker of KV7.1 (Kalstrup and Blunck, 2018). Co-expression of KCNE1 with KV7.1 imparts a more depolarized voltage dependence of activation, slower activation kinetics, and increased single-channel conductance compared to KV7.1 alone (Sun et al., 2012; Yang and Sigworth, 1998). Loss-of-function mutations in the cardiac IKs channel can lead to an arrhythmogenic disorder known as long QT syndrome (LQTS), which predisposes individuals to ventricular fibrillation and sudden cardiac death (Fernández-Falgueras et al., 2017; Roden, 2008; Watanabe et al., 2005). Current treatments for LQTS include pharmacological intervention with β-blockers or surgical implantation of a cardioverter defibrillator (Waddell-Smith and Skinner, 2016). However, limitations of these treatments generate a need for novel therapeutic interventions to treat LQTS.
Polyunsaturated fatty acids (PUFAs) are amphipathic molecules composed of a charged hydrophilic head group and a long, polyunsaturated hydrophobic tail group (Jump, 2002). It is well documented that PUFAs form a group of IKs channel activators that interact with the channel VSD, thus influencing the voltage dependence of IKs channel activation (Moreno et al., 2015; Moreno et al., 2012; Elinder and Liin, 2017). The ability of PUFAs to activate the IKs channel makes them great candidates for potential LQTS therapeutics. PUFAs promote IKs channel activation through an electrostatic interaction between the negative charge of the hydrophilic PUFA head and positively charged arginine residues in the S4 segment of the IKs channel (Elinder and Liin, 2017; Börjesson and Elinder, 2011; Larsson et al., 2020; Börjesson et al., 2008). This electrostatic activation of the IKs channel is seen as a leftward shift in the voltage dependence of IKs channel activation that leads to increases in IKs current. Recently, it has been reported that PUFAs increase IKs current through two independent effects: one on S4 (as described above) and one on the PD through an electrostatic interaction with a positively charged lysine residue located in S6 (K326) (Yazdi et al., 2021; Liin et al., 2018). This electrostatic interaction with the K326 mediates an increase in the maximal conductance (Gmax) of the IKs channel (Yazdi et al., 2021; Liin et al., 2018). The mechanism through which the negatively charged PUFA head group interacts with positive charges of S4 and S6 is called the lipoelectric hypothesis where the polyunsaturated tail of PUFAs and PUFA analogues incorporates into the cell membrane via hydrophobic interactions and electrostatically attracts the outermost gating charges of S4 as well as positively charged K326 in the S6 segment (Börjesson et al., 2008; Yazdi et al., 2021; Bohannon et al., 2019; Bohannon et al., 2020).
PUFA analogues that have the most robust effects on increasing IKs current are those that have a low pKa value and thus possess a negatively charged head group at physiological pH (Bohannon et al., 2020). Examples include PUFAs with glycine or taurine head groups that possess either a carboxyl or sulfonyl head group, respectively (Bohannon et al., 2020; Liin et al., 2016). We have observed that another PUFA analogue, N-(α-linolenoyl) tyrosine (NALT), has robust effects on IKs current. NALT is unique in that it possesses a large aromatic tyrosine head group rather than a carboxyl or sulfonyl group present in most of the PUFAs and PUFA analogues that we have characterized. NALT induces a potent leftward shift in the voltage dependence of IKs channel activation and an increase in the maximal channel conductance, thus increasing overall IKs current. Here, we aim to determine the mechanism behind IKs activation by NALT using PUFA analogues with aromatic and modified aromatic head groups. Better understanding of the mechanism of how these aromatic PUFA analogues have more potent IKs channel activation effects could help to aid future drug development for LQTS.
Results
Diverse PUFA analogues with a tyrosine head group activate the IKs channel
To measure the effects of the aromatic PUFA analogues on the cardiac IKs channel, we expressed the IKs channel complex in Xenopus laevis oocytes (Figure 1A). We co-injected mRNA for the KV7.1 α-subunit and the KCNE1 β-subunit to achieve expression of tetrameric IKs channels. Using two-electrode voltage-clamp recordings, we applied depolarizing voltage steps to activate the IKs channel (Figure 1B) before and after applying four different tyrosine PUFA analogues: N(α-linolenoyl)-tyrosine (NALT), linoleoyl tyrosine (Lin-tyrosine), docosahexanoyl tyrosine (DHA-tyrosine), and pinoleoyl tyrosine (Pin-tyrosine) (Figure 1C). From these voltage-clamp experiments, we are also able to acquire dose–response curves for different aspects of IKs channel activation, including changes in overall IKs current (I/I0, Figure 1D), changes in the voltage dependence of activation (ΔV0.5, Figure 1E), and changes in the maximal channel conductance (Gmax/Gmax0, Figure 1F). NALT, Lin-tyr, DHA-tyr, and Pin-tyr all activate the cardiac IKs channel by shifting the voltage dependence of IKs channel activation to more negative voltages (NALT: ΔV0.5 = –56.2 ± 3.6 mV; Lin-tyr: –74.4 ± 4.1 mV; DHA-tyr: –72.0 ± 4.9 mV; and Pin-tyr: –60.5 ± 5.8 mV at 20 μM; Figure 1E) and increasing the maximal conductance (NALT: 1.43 ± 0.3; Lin-tyr: 2.0 ± 0.6; DHA-tyr: 1.5 ± 0.2; and Pin-tyr: 1.8 ± 0.4 at 20 μM; Figure 1F). Together, the left shift in V0.5 and the increase in Gmax increase the overall IKs current measured in response to a voltage step close to 0 mV (NALT: 5.14 ± 1.2; Lin-tyr: 12.8 ± 2.1; DHA-tyr: 5.0 ± 0.9; and Pin-tyr: 5.8 ± 0.9 at 20 μM; Figure 1D: see ‘Materials and methods’ for calculation of I/I0). In comparison, Lin-glycine (a known IKs channel activator; Figure 1C) causes only modest leftward voltage shifts (ΔV0.5 = –30.8 ± 5.4 mV at 20 μM; Figure 1E), but similar increases in maximal conductance (2.6 ± 0.5 at 20 μM; Figure 1F) and I/I0 current (6.7 ± 1.1 at 20 μM; Figure 1D) as for most tyrosine PUFAs.
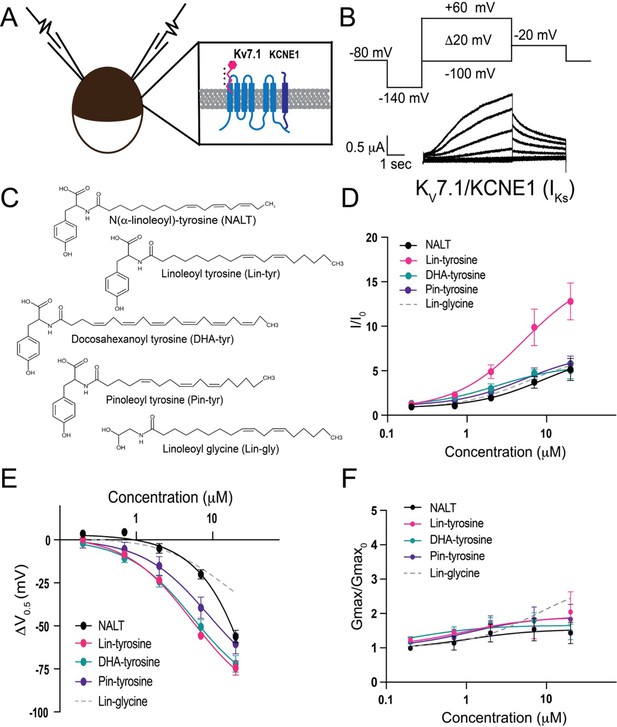
Polyunsaturated fatty acids (PUFA) analogues with a tyrosine head group are strong IKs channel activators.
(A) Schematic of two electrode voltage-clamp setup (inset: IKs channel cartoon + PUFA [pink]). (B) Voltage protocol (top) with representative KV7.1/KCNE1 (IKs) current (bottom). (C) Structures of NALT, Lin-tyrosine, DHA-tyrosine, and Pin-tyrosine (with Lin-glycine for comparison). (D–F) I/I0, (E) ΔV0.5, and (F) Gmax dose–response curves for NALT (black circles) (n = 4), Lin-tyrosine (pink circles) (n = 4), DHA-tyrosine (teal circles) (n = 3), Pin-tyrosine (purple circles) (n = 5), and Lin-glycine (gray dotted line) (n = 3). Values for all compounds and concentrations available in Figure 1—source data 1.
-
Figure 1—source data 1
Source Data for Panels D-F.
- https://cdn.elifesciences.org/articles/85773/elife-85773-fig1-data1-v2.docx
Distal –OH group is necessary for robust activation of the IKs channel
Amino acids with aromatic groups (like tryptophan, tyrosine, and phenylalanine) can participate in cation–pi interactions (Dougherty, 2007). Cation–pi interactions take place between the pi-electrons of an aromatic ring and positively charged (cationic) groups (such as arginine and lysine) (Infield et al., 2021). If tyrosine PUFAs activate the IKs channel via cation–pi interactions, we would expect that other aromatic groups (such as phenylalanine) would similarly affect IKs activation. We tested two different PUFA analogues that both contain a phenylalanine head group – linoleoyl phenylalanine (Lin-phe) and N-(α-linolenoyl) phenylalanine (NAL-phe) (Figure 2A). Lin-phe and NAL-phe both increase I/I0 (Lin phe: 2.6 ± 0.3; and NAL-phe: 2.4 ± 0.5 at 20 μM; Figure 2B–D), causing a modest leftward shift in the V0.5 (Lin-phe: ΔV0.5 = –13.1 ± 2.9 mV; and NAL-phe: –12.5 ± 3.8 mV at 20 μM; Figure 2E and F). However, Lin-phe and NAL-phe have minimal effects on the Gmax (Lin phe: 1.2 ± 0.1; and NAL-phe: 1.2 ± 0.2 at 20 μM; Figure 2G and H). All of these effects (I/I0, ΔV0.5, and Gmax) are reduced in comparison with tyrosine PUFAs, with Lin-phe and NAL-phe having an attenuated effect compared to NALT (p=0.51 and p=0.44, respectively; Figure 2D) and resulting in a significantly smaller increases in I/I0 compared to Lin-tyrosine (***p=0.0004 and ***p=0.0004, respectively; Figure 2D). In addition, both NALT and Lin-tyrosine cause a significantly greater ΔV0.5 compared to NAL-phe and Lin-phe (****p<0.0001; Figure 2F). Together, these differences suggest that cation–pi interactions are not the primary mechanism through which tyrosine PUFAs activate the IKs channel. Rather, our data suggest that it is actually the presence of the distal –OH group on the aromatic head group that is critical for the potent activation of the IKs channel because the loss of this –OH group (Lin-phe and NAL-phe) results in pronounced reductions in PUFA efficacy.
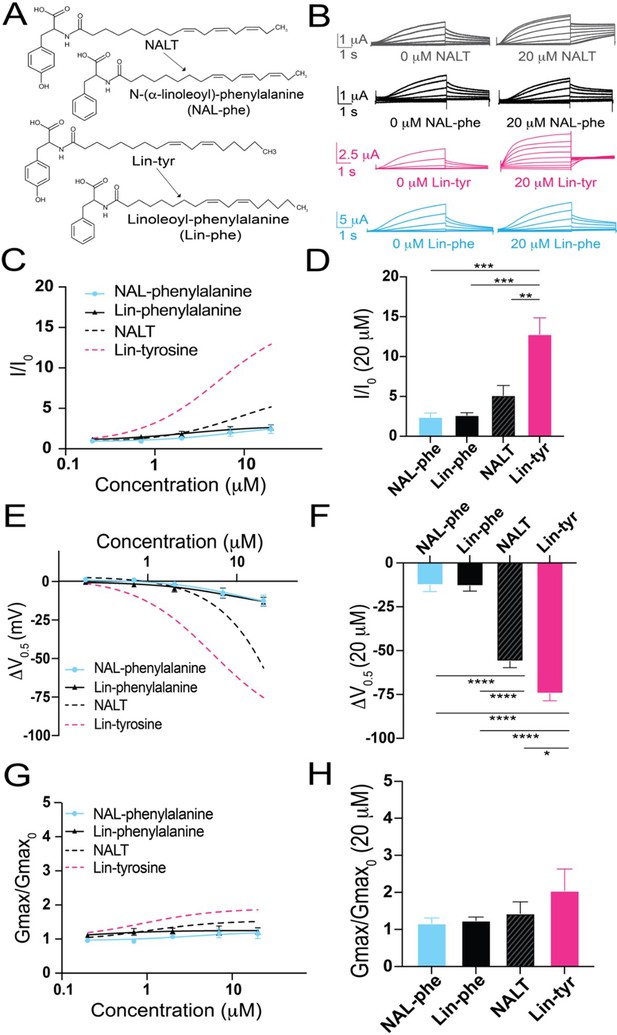
The distal hydroxyl (–OH) group of tyrosine polyunsaturated fatty acid (PUFA) analogues is necessary for robust IKs channel activation.
(A) Structures of NAL-phe and Lin-phe. (B) Representative current traces for NALT (gray), NAL-phe (black), Lin-tyr (pink), and Lin-phe with 0 μM PUFA (left) and 20 μM PUFA (right). (C, E, G) I/I0, (E) ΔV0.5, and (G) Gmax dose–response curves for NAL-phe (n = 4) and Lin-phe (n = 4) with dotted lines representing dose response of NALT (n = 4) and Lin-tyr (n = 4). (D, F, H) Maximum effects on (D) I/I0, (F) ΔV0.5, and (H) Gmax (at 20 μM) for NAL-phe (n = 4), Lin-phe (n = 4), NALT (n = 4), and Lin-tyr (n = 4). Asterisks indicate statistically significant differences determined by one-way ANOVA with Tukey’s test for multiple comparisons. Values for all compounds and concentrations available in Figure 2—source data 1.
-
Figure 2—source data 1
Source Data for Panels C-H.
- https://cdn.elifesciences.org/articles/85773/elife-85773-fig2-data1-v2.docx
Electronegative groups on aromatic ring are important for increases in maximal conductance
Our data thus far indicates that it is the presence of the –OH group, not cation–pi interactions, that is critical for pronounced IKs channel activation by tyrosine PUFAs. The –OH group found in tyrosine PUFAs is highly electronegative. To test how electronegativity influences IKs channel activation, we compared three modified phenylalanine PUFAs, which all include a highly electronegative group(s) attached to the aromatic ring. We compared N-(α-linolenoyl)–4-bromo-L-phenylalanine (4Br-NAL-phe), N-(α-linolenoyl)–4-fluoro-L-phenylalanine (4F-NAL-phe), and N-(α-linolenoyl) 3,4,5-trifluorophenylalanine (3,4,5F-NAL-phe) (Figure 3A). 4Br-NAL-phe, 4F-NAL-phe, and 3,4,5F-NAL-phe application increases I/I0 (4Br-NAL-phe: 4.6 ± 0.1 at 20 μM; 4F-NAL-phe: 4.6 ± 1.1 at 20 μM; 3,4,5F-NAL-phe: 7.1 ± 1.0 at 20 μM; Figure 3B–D), causes a leftward shift in the V0.5 (4Br-NAL-phe: ΔV0.5 = –22.8 ± 2.0 mV; 4F-NAL-phe: –23.9 ± 0.8 mV; 3,4,5F-NAL-phe: –32.4 ± 4.9 mV at 20 μM; Figure 3E and F) and increases the Gmax (4Br-NAL-phe: 1.9 ± 0.1 at 20 μM; 4F-NAL-phe: 2.0 ± 0.5; 3,4,5F-NAL-phe: 2.4 ± 0.4 at 20 μM; Figure 3G and H). Increasing the number of highly electronegative groups significantly improves the effects of phenylalanine PUFAs on increasing I/I0 and shifting the V0.5, evidenced by significant increases in I/I0 (*p=0.0186; Figure 3D) and a significantly greater leftward shift in the V0.5 (**p=0.0096; Figure 3F) from 3,4,5F-NAL-phe compared to NAL-phe alone. Interestingly, though, NALT still causes the most prominent left-shift in the V0.5 compared to 4Br-, 4F-, and 3,4,5F-NAL-phe (***p=0.0003; ***P=0.00028; and **p=0.0021, respectively). These data suggest that the presence of highly electronegative groups improve the activating effects of phenylalanine PUFAs on the IKs channel. However, they do not completely recapitulate the effects of tyrosine PUFAs on the shift in V0.5 of the IKs channel.
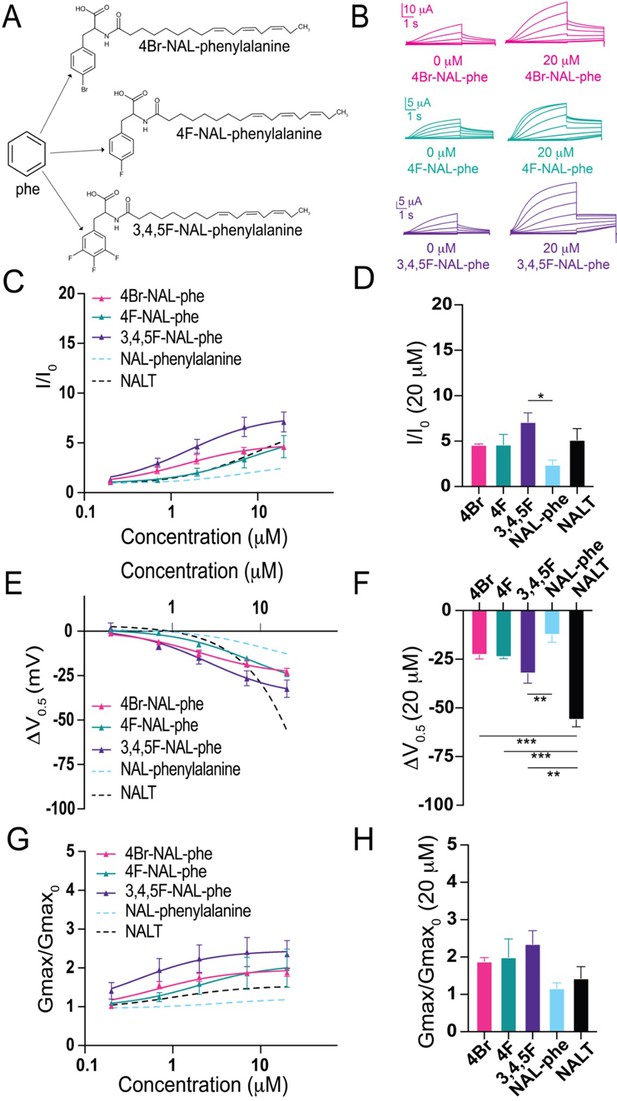
The addition of electronegative atoms to phenylalanine polyunsaturated fatty acid (PUFA) analogues strengthens IKs channel activation through improved effects on Gmax.
(A) Structures of 4Br-NAL-phe, 4F-NAL-phe, and 3,4,5F-NAL-phe. (B) Representative traces for 4Br-NAL-phe (pink), 4F-NAL-phe (teal), and 3,4,5F-NAL-phe (purple) with 0 μM PUFA (left) and 20 μM PUFA (right). (C, E, G) I/I0, (E) ΔV0.5, and (G) Gmax dose–response curves for NAL-phe (n = 4), 4Br-NAL-phe (n = 3), 4F-NAL-phe (n = 4), and 3,4,5F-NAL-phe (n = 5) with dotted line representing dose response of NALT (n = 4). (D, F, H) Maximum effects on (D) I/I0, (F) ΔV0.5, and (H) Gmax (at 20 μM) for 4Br-NAL-phe (n = 3), 4F-NAL-phe (n = 4), and 3,4,5F-NAL-phe (n = 5). Asterisks indicate statistically significant differences determined by one-way ANOVA with Tukey’s test for multiple comparisons. Values for all compounds and concentrations available in Figure 3—source data 1.
-
Figure 3—source data 1
Source Data for Panels C-H.
- https://cdn.elifesciences.org/articles/85773/elife-85773-fig3-data1-v2.docx
Hydrogen bonding is important for pronounced leftward shifts in IKs channel voltage dependence
The presence of the –OH group on tyrosine PUFA analogues or the addition of electronegative groups to the phenylalanine head group improves IKs activation. However, a persistent and striking difference between tyrosine PUFAs and modified phenylalanine PUFAs in the magnitude of their voltage-shifting effects with the tyrosine PUFAs having an almost twice as big voltage shift effect than the modified phenylalanine PUFAs (Figure 3E and F). One explanation for this discrepancy is that the –OH group can also behave as a hydrogen bond donor. To determine whether hydrogen bonding contributes to the activating effects of tyrosine PUFA analogues, we applied the modified aromatic PUFA analogue N-(α-linolenoyl)–3-fluoro-L-tyrosine (3F-NALT), which has a fluorine atom adjacent to the tyrosine hydroxyl group (Figure 4A). The addition of the fluorine atom reduces the pKa of the distal hydroxyl group and increases the hydrogen bonding ability of said group in 3F-NALT as compared to NALT. Overall, the maximum effects on I/I0 are similar for 3F-NALT and NALT (3F-NALT: 5.0 ± 1.0; NALT: 5.14 ± 1.2 at 20 μM; p=0.7257, ns; Figure 4B–D). Notably, 3F-NALT induces a significantly greater maximum shift in the V0.5 (ΔV0.5 = –69.3 ± 1.4 at 20 μM) compared to NALT (–56.1 ± 3.6 AT 20 μM) (p=0.0298*; Figure 4E and F), while the effects on Gmax are not significantly different between 3F-NALT and NALT (3F-NALT: 1.3 ± 0.3; NALT: 1.4 ± 0.3 at 20 μM; p=0.7324, ns; Figure 4G and H). These data demonstrate that increasing the hydrogen bonding capacity of the –OH group increases the maximum shift in IKs channel voltage dependence. This implicates hydrogen bonding as an important mechanism for IKs activation and preferentially influences the effects on the voltage dependence of IKs activation.
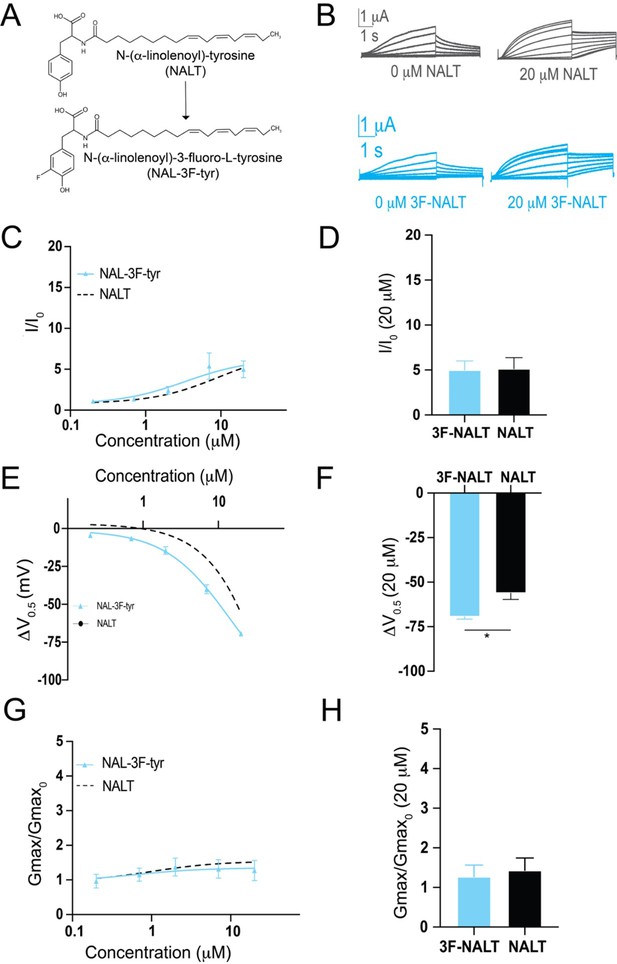
Hydrogen bonding through the distal –OH group of tyrosine polyunsaturated fatty acids (PUFAs) is important for effects on IKs channel voltage dependence.
(A) Structures of NALT and 3F-NALT. (B) Representative traces of NALT (gray) and 3F-NALT (cyan) with 0 μM PUFA (left) and 20 μM PUFA (right). (C, E, G) I/I0, (E) ΔV0.5, and (G) Gmax dose–response curves for NALT (black dashed line) (n = 4) and 3F-NALT (cyan) (n = 3). (D, F, H) Maximum effects on (D) I/I0, (F) ΔV0.5, and (H) Gmax (at 20 μM) for 3F-NALT (n = 3) and NALT (n = 4). Values for all compounds and concentrations available in Figure 4—source data 1.
-
Figure 4—source data 1
Source Data for Panels C-H.
- https://cdn.elifesciences.org/articles/85773/elife-85773-fig4-data1-v2.docx
Aromatic PUFAs appear to activate the IKs channel in similar mechanisms as non-aromatic PUFAs
To better understand the mechanism of these more effective aromatic PUFAs, we mutated residues previously shown to be important for non-aromatic PUFA activating effects on IKs channels. The residue R231, located in the voltage sensor (S4) (Figure 5A), has been previously shown to be important for the V0.5 shifting effect of non-aromatic PUFAs (Liin et al., 2018). We tested Lin-tyr, the largest V0.5 shifting aromatic PUFA, on the IKs channel with the mutation R231Q+Q234R to assess whether R231 is also important for the aromatic PUFA V0.5 shifting mechanism. The additional mutation Q234R is necessary to preserve the voltage dependence of activation in IKs channels with the R231Q mutation (Liin et al., 2018; Panaghie and Abbott, 2007; Wu et al., 2010). The V0.5 shifting effect of Lin-tyr was significantly decreased from ΔV0.5 = –74.4 mV ± 4.1 at 20 μM in the wild-type (WT) IKs channel toΔV0.5 = –36.5 mV ± 7.3 at 20 μM with the R231Q+Q234R mutation (****p < 0.0001; Figure 5B and C). This reduction indicates that R231 contributes to more than half of the voltage dependence shifting effect of Lin-tyr. The remaining shift is most likely due to PUFA head group interactions with other nearby S4 charges such as R228 and Q234R.
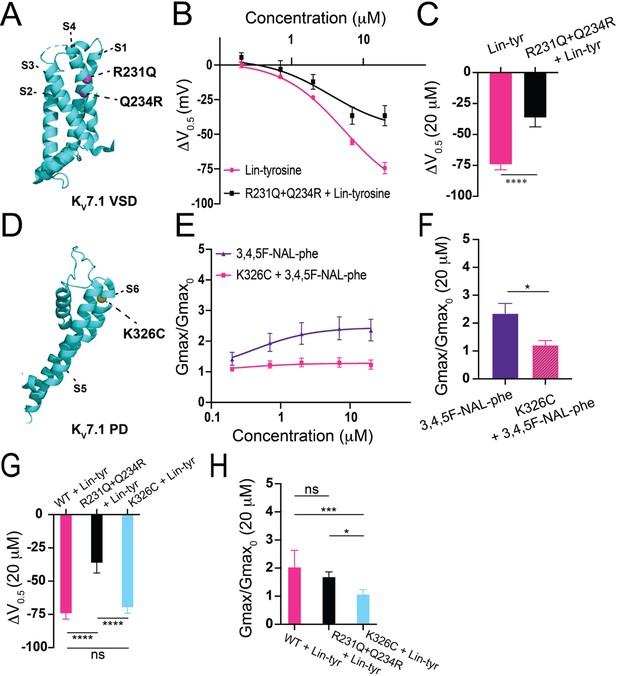
Proposed mechanisms of aromatic polyunsaturated fatty acids (PUFAs).
(A) Structure of KV7.1 voltage-sensing domain (VSD) based on PDB: 6V00A projected using PyMOL Software (Schrödinger L and DeLano 2020). Pink spheres indicate mutated residues in the S4 segment, R321Q-Q234R, which are implicated in PUFA-mediated effects on voltage dependent activation. (B) ΔV0.5 dose–response curve for WT KV7.1/KCNE1 + Lin-tyr (pink) (n = 4) and KV7.1-R231Q-Q234R/KCNE1 + Lin-tyr (black) (n = 5). (C) Maximum effects on ΔV0.5 (at 20 μM) for WT KV7.1/KCNE1 + Lin-tyr (n = 4) and KV7.1-R231Q-Q234R/KCNE1 + Lin-tyr (n = 5). (D) Structure of KV7.1 pore domain (PD). Yellow spheres indicate mutated residue in the S6 segment, K326C, which is implicated in PUFA-mediated effects on maximal conductance. (E) Gmax dose–response curve for WT KV7.1/KCNE1 + 3,4,5F-NAL-phe (purple) (n = 5) and KV7.1-K326C/KCNE1 + 3,4,5F-NAL-phe (pink) (n = 3). (F) Maximum effects on Gmax (at 20 μM) for WT KV7.1/KCNE1 + 3,4,5F-NAL-phe (n = 5) and KV7.1-K326C/KCNE1 + 3,4,5F-NAL-phe (n = 3). (G) Maximum effects on ΔV0.5 (at 20 μM) of Lin-tyr on WT KV7.1/KCNE1 (n = 4), KV7.1-R231Q-Q234R/KCNE1 (n = 5), and KV7.1-K326C/KCNE1 (n = 6). (H) Maximum effects on Gmax (at 20 μM) of Lin-tyr on WT KV7.1/KCNE1 (n = 6), KV7.1-R231Q-Q234R/KCNE1 (n = 5), and KV7.1-K326C/KCNE1 (n = 6). Values for all compounds and concentrations available in Figure 5—source data 1.
-
Figure 5—source data 1
Source Data for Panels B, C, E, F, G, and H.
- https://cdn.elifesciences.org/articles/85773/elife-85773-fig5-data1-v2.docx
The residue K326, located near the pore, has been previously shown to be important for the Gmax increasing effect of non-aromatic PUFAs (Liin et al., 2018). We tested 3,4,5F NAL-phe, the largest Gmax increasing aromatic PUFA, on the IKs channel with the mutation K326C to assess whether K326 is also important for the aromatic PUFA Gmax increasing mechanism (Figure 5D). The Gmax increasing effect of 3,4,5F NAL-phe was significantly decreased from 2.4 ± 0.4 at 20 μM in the WT IKs channel to 1.22 ± 0.2 at 20 μM (*p=0.0287; Figure 5E and F). This reduction indicates that K326 is necessary for 3,4,5F NAL-phe’s Gmax increasing effect.
To test whether the V0.5 shift and Gmax increasing effects of aromatic PUFAs are independent of each other, we compare the Lin-tyr effects on both of the above mutations. The mutation R231Q+Q234R significantly decreases the V0.5 shift effect from ΔV0.5 = –74.4 mV ± 4.1 at 20 μM in the WT IKs channel to ΔV0.5 = –36.5 mV ± 7.3 at 20 μM in the mutation (****p < 0.0001), as mentioned above, but does not change Gmax increasing effect of Lin-tyr (Figure 5G and H). Similarly, the mutation K326C significantly decreases the Gmax effect of Lin-tyr from Gmax = 2.04 ± 0.59 at 20 μM in the WT IKs channel to Gmax = 1.08 ± 0.15 at 20 μM in the mutation (***p=0.0015) but does not change the V0.5 shift effect of Lin-tyr (Figure 5G and H). Overall, Figure 5 demonstrates that aromatic PUFAs are shifting the voltage dependence and increasing the current maximum by two independent mechanisms: one via interactions with S4 charges (R231) and one via interactions with K326.
Residue T224 in the S3–S4 loop is a novel locus for hydrogen bond formation between the IKS channel and tyrosine PUFAs
Our experiments using fluorinated NALT (NAL-3F-tyr) to improve the hydrogen bonding capacity of the tyrosine head group demonstrated that hydrogen bonding by the tyrosine’s para-hydroxyl group is the reason for the large effect of PUFAs with tyrosine head groups on the IKs channel voltage-dependent activation. To identify the residue with which the tyrosine head group hydrogen bonds, we mutated residues in the S3–S4 loop capable of hydrogen bond formation. We chose residues in the S3–S4 loop because it is located near the top of the PUFA head group in the proposed binding site for PUFAs to have the voltage-dependence effect. We individually mutated serine 217 (S217A), glutamine 220 (Q220L), threonine 224 (T224V), and serine 225 (S225A) and compared the effects of NALT on mutated channels compared to the WT IKs channel (Figure 6A and B). We found that S217A, Q220L, and S225A showed similar maximum shifts in voltage-dependent activation compared to the wild-type channel (WT + NALT: ΔV0.5 = –56.1 ± 3.6 mV; S217A + NALT: –65.9 ± 3.7 mV; Q220L + NALT: –59.5 ± 11.1 mV; S225A + NALT: –52.4 ± 3.7 mV at 20 μM, ns; Figure 6C and D). However, the T224V mutation significantly attenuated the leftward shift in the voltage dependence of activation in response to NALT application from ΔV0.5 = –56.1 ± 3.6 mV in WT channels to ΔV0.5 = –32.1 ± 7.0 at 20 μM (*p=0.03; Figure 6D). To determine whether this effect was specific to compounds with the ability to form hydrogen bonds, we compared the effects of hydrogen-bonding NALT and non-hydrogen-bonding NAL-phe on T224V mutant channels (Figure 6E). In contrast to the attenuation of the overall voltage shift observed when NALT was applied to the T224V, there was no difference in the voltage-shifting effects of NAL-phe between the T224V mutant and WT channels (WT + NAL-phe:ΔV0.5 = –12.5 ± 3.8 mV; T224V + NAL-phe: –13.2 ± 2.6 mV at 20 μM, ns; Figure 6F and G). These data demonstrate that the T224V mutation only reduces the efficacy of aromatic PUFAs that contain a hydrogen-bonding group like tyrosine. As a result, we have identified a novel interaction between the S3–S4 loop residue T224 and hydrogen bonding moieties of aromatic PUFA head groups. Figure 7A shows the distance between the residues R231 and T224V in KCNQ1 is 9.1 Å and the length of a tyrosine head group is 8.5 Å. The tyrosine head group fits nicely in between these two residues in silico, demonstrating the possibility of this interaction to occur (Figure 7A).

Loop is the locus for hydrogen bonding interactions with tyrosine polyunsaturated fatty acids (PUFAs).
(A) Top view of KV7.1 voltage-sensing domain (VSD) highlighting mutated residues in the S3–S4 loop. (B) Representative traces of WT KV7.1/KCNE1 (black), KV7.1-S217A/KCNE1 (red), KV7.1-Q220L/KCNE1 (teal), KV7.1-T224V/KCNE1 (cyan), and KV7.1-S225A/KCNE1 (purple) with 0 μM (left) and 20 μM (right) NALT. (C) ΔV0.5 dose–response curve for WT KV7.1/KCNE1 (n = 4), KV7.1-S217A/KCNE1 (n = 5), KV7.1-Q220L/KCNE1 (n = 3), KV7.1-T224V/KCNE1 (n = 4), and KV7.1-S225A/KCNE1 (n = 7) with NALT. (D) Maximum effects on ΔV0.5 (at 20 μM) for WT and S3–S4 loop mutations. Asterisks indicate statistically significant differences determined by one-way ANOVA. (E) Representative traces of WT KV7.1/KCNE1 with NALT (black) and NAL-phe (gray) compared to KV7.1-T224V/KCNE1 with NALT (cyan) and NAL-phe (dark purple), KV7.1-S217A/KCNE1 (red), KV7.1-Q220L/KCNE1 (teal), KV7.1-T224V/KCNE1 (cyan), and Kv7.1-S225A/KCNE1 (purple) with 0 μM (left) and 20 μM (right) NALT. (F) ΔV0.5 dose–response curve for WT KV7.1/KCNE1 and KV7.1-T224V/KCNE1 with NALT and NAL-phe. (G) Maximum effects on ΔV0.5 (at 20 μM) for WT KV7.1/KCNE1 (n = 4) and KV7.1-T224V/KCNE1 with NALT (n = 4) and NAL-phe (n = 7). Asterisks indicate statistically significant differences determined by one-way ANOVA. Values for all compounds and concentrations available in Figure 6—source data 1.
-
Figure 6—source data 1
Source Data for Panel C, D, F, and G.
- https://cdn.elifesciences.org/articles/85773/elife-85773-fig6-data1-v2.docx
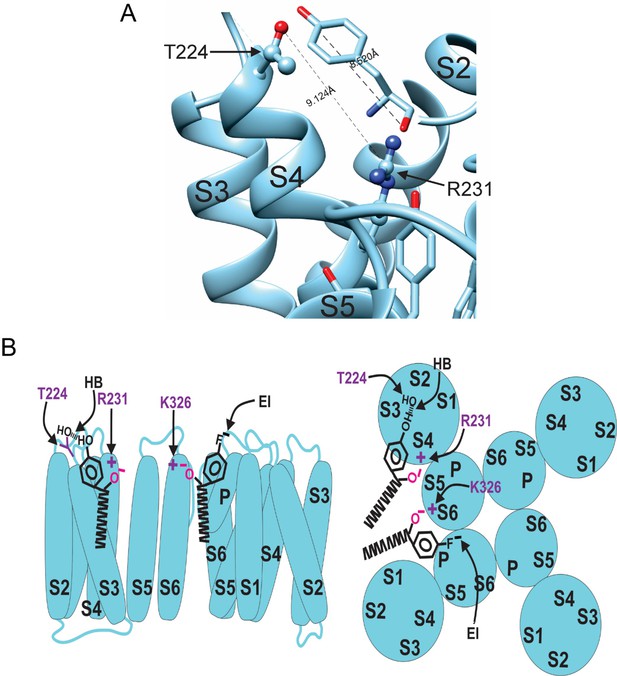
Model for aromatic polyunsaturated fatty acid (PUFA) interaction with Kv7.1/KCNE1 channels.
(A) Image of the KCNQ1 structure (PDB 6UZZ) marking the distance between R231 and T224. This distance is shown in comparison to the length of a tyrosine head group. (B) Model for aromatic PUFAs effect on KV7.1/KCNE1 channels, side view (left) and top view (right). One site is between S4 and S5: Aromatic PUFAs shift the voltage dependence of opening by stabilizing the upstate of S4 by an electrostatic interaction between R231(+) and the carboxyl group (O-) of the PUFA. A hydrogen bond (HB) by the hydroxyl group (OH) at the para site of the aromatic ring of the PUFA with T224 stabilizes the PUFA in this site. Another site is between S6 and S1: aromatic PUFAs increase the maximum conductance by an electrostatic interaction between K326(+) and the carboxyl group (O-). An electrostatic interaction (EI) by the para fluorine (F-) stabilize the PUFA in this site.
In our proposed mechanism, aromatic PUFA analogues modulate the IKs channel via two independent interactions (Figure 5). In one site, the carboxyl group is interacting with R231 (in S4) and the distal –OH is hydrogen bonding with T224 (in the S3–S4 loop) (Figure 7B). In the other site, the carboxyl group is interacting with K326 (in S6) and the distal electronegative atom stabilizes the PUFA via an electrostatic interaction (Figure 7B).
Discussion
We have found that PUFA analogues with tyrosine head groups are strong activators of the cardiac IKs channel. Tyrosine PUFAs shift the voltage dependence of activation to negative potentials and increase the maximal conductance, which together contribute to increases in overall IKs current. The tyrosine head group is an aromatic ring with a distal -OH group in the para-position. Tyrosine PUFA analogues have the potential to interact with the IKs channel through several candidate mechanisms involving either the aromatic ring or the -OH group (or both). The aromatic ring could modulate IKs channel function through cation–pi interactions with positively charged groups on the IKs channel. In addition, the -OH group could participate in electrostatic interactions and/or act as a hydrogen bond donor. In this work, we elucidate the mechanisms of this PUFA-induced activation of the IKs channel by applying PUFA analogues with modified aromatic head groups designed to test specific chemical interactions between the PUFA head group and the IKs channel.
If cation–pi interactions were the primary mechanism through which tyrosine PUFAs activate the IKs channel, we would expect similar activating effects of PUFA analogues with aromatic rings that lack the –OH group, such as phenylalanine. However, PUFA analogues with phenylalanine head groups (Lin-phe and NAL-phe) do not activate the IKs channel to the same degree as PUFA analogues with a tyrosine head group (Lin-tyr and NALT) and display significant reductions in efficacy for increases in I/I0 and shifts in the V0.5. Further evidence that cation–pi interactions are not a predominant mechanism for IKs channel activation by tyrosine PUFA analogues comes from experiments applying fluorinated phenylalanine PUFAs (4F-NAL-phe and 3,4,5F-NAL-phe), which can be used as a tool to probe cation–pi interactions in ion channel function (Pless et al., 2014). Pless et al., 2014 demonstrated that tri-fluorination of phenylalanine disperses the electrostatic surface potential that is necessary for cation–pi interactions (Pless et al., 2014). Disruption of the electrostatic surface potential through addition of fluorine atoms to the NAL-phe head group (3,4,5F-NAL-phe), therefore, is expected to reduce the efficacy of 3,4,5F-NAL-phe in comparison to NAL-phe alone. However, we find the opposite when we apply 3,4,5F-NAL-phe to the cardiac IKs channel, and see that 3,4,5F-NAL-phe is a more potent activator of the IKs channel compared to NAL-phe alone. Together, these data suggest that cation–pi interactions are not the primary mechanism through which these aromatic PUFA analogues activate the cardiac IKs channel.
When we look at several fluorinated and brominated phenylalanine PUFA analogues, we find specifically that 3,4,5F-NAL-phe has significantly greater effects on I/I0 and ΔV0.5 compared to NAL-phe alone. While not statistically significant, 4Br-, 4F-, and 3,4,5F-NAL-phe also lead to some of the most consistent increases in Gmax among the PUFA analogues tested in this work, with each of these compounds leading to a twofold increase in Gmax. These data suggest that aromatic PUFA analogues with highly electronegative atoms on the distal end of the aromatic head group have the most pronounced effects on the maximal conductance of the IKs channel. Although brominated and fluorinated phenylalanine analogues increase the maximal conductance of the IKs channel, these modified PUFAs still fail to recapitulate the leftward ΔV0.5 observed with tyrosine PUFA analogues. While the –OH group of tyrosine PUFA analogues is indeed strongly electronegative, it can also act as a hydrogen bond donor. When we applied a fluorinated tyrosine PUFA (3F-NALT) to increase hydrogen bonding abilities, we found that this leads to a stronger leftward shift in the voltage dependence of IKs activation. This suggests that hydrogen bonding via the –OH group contributes to the left-shifting effects of voltage-dependent activation through effects on the IKs channel voltage sensor. Most notably, these results suggest that specific modifications to the aromatic PUFA head group can preferentially improve either the voltage-shifting or maximal conductance effects of PUFA analogues. Our data suggests that adding highly electronegative groups to an aromatic ring, such as bromine and fluorine, most consistently improve the maximal conductance-increasing effects and reduce voltage dependence-shifting effects relative to PUFA analogues with a tyrosine or phenylalanine head group. On the other hand, we found that reducing the pKa of the –OH group (and increasing the potential for hydrogen bonding), while leaving the effect on Gmax intact, preferentially improves the voltage-shifting effects on the IKs channel.
Previous work has demonstrated that PUFA analogues have two independent effects on IKs channel activation. PUFA analogues are known to shift the voltage dependence of activation in the IKs channel through electrostatic effects on the channel voltage sensor (Börjesson et al., 2008; Liin et al., 2015). This is mediated by interactions of the negative PUFA head group with the outermost positively charged arginine residues located in the S4 segment (Liin et al., 2016; Liin et al., 2015). Recently, though, a second effect on the IKs channel pore has been reported to influence the maximal conductance of the IKs channel (Liin et al., 2018). This is mediated through electrostatic interactions between the PUFA head groups and a positively charged lysine residue in the S6 segment – K326 (Liin et al., 2018). In addition, molecular dynamics (MD) simulations with the Kv7.1 (KCNQ1) channel (the pore-forming domain for the IKs channel) (Yazdi et al., 2021) identified two separate high-occupancy sites for linoleic acid: site 1 at R231 in the S4 segment, and site 2 at K326 in the S6 segment (Yazdi et al., 2021). We here show that the more effective aromatic PUFAs also act on these sites in S4 and S6. To do this, we selected the best V0.5 shifting aromatic PUFA (Lin-tyr) to test on the IKs channel with the S4 mutation R231Q. Additionally, we selected the best Gmax increasing aromatic PUFA (3,4,5F-NAL-phe) to test on the IKs channel with S6 mutation K326C. The mutation R231Q decreases the V0.5 shifting effect of Lin-tyr by half, indicating that Lin-tyr is shifting the voltage dependence by creating an electrostatic interaction with the positive charges on the voltage sensor. Conversely, the mutation K326C almost completely removed the Gmax increasing effect of 3,4,5F-NAL-phe. To demonstrate that these effects are also independent of each other in aromatic PUFAs, we evaluate the V0.5 shifting effect and the Gmax increasing effect of Lin-tyr on both mutations. The R231Q mutation decreases V0.5 shifting effect but does not change the Gmax effect of Lin-tyr. While the K326 mutation decreases the Gmax effect but does not change the V0.5 shifting effect of Lin-tyr. We therefore propose that the increased effects of the aromatic PUFAs, compared to non-aromatic PUFAs, are due to the additional hydrogen bonding in site 1 and electrostatic interactions in site 2 to better anchor them in these binding sites to increase their effects (Figure 7B). As mentioned above, we also show that the aromatic rings have the potential to be modified to give preferential effects on either the IKs channel voltage sensor or channel pore.
Our experiments with NAL-Phe and 3F-NALT show that the hydrogen bonding capacity of the –OH on the tyrosine of NALT is necessary for it to have a more effective voltage dependence shift effect. We further discovered the specific details of the hydrogen bond interactions between this –OH group of NALT and the S3–S4 loop of the IKs channel. We mutated all residues capable of hydrogen bonding in the S3–S4 loop, removing their ability to hydrogen bond and tested whether this changed the NALT voltage dependence shifting effect. The voltage dependence of mutations S217A, Q220L, and S225A was shifted to the same degree by NALT as the wild-type IKs channel. However, the voltage dependence of mutation T224V was shifted significantly less than the WT IKs channels. This shows that the –OH group on the tyrosine of NALT hydrogen bonds with T224V, thereby improving the PUFA’s ability to shift the voltage dependence. This hydrogen bond interaction between PUFAs and the 3–4 loop of the IKs channel is a novel mechanism to increase the effect of PUFAs to activate the IKs channel. These data suggest that the drugs designed to target this interaction would be more effective at shifting IKs channel voltage dependence.
Overall, our findings suggest that different aromatic PUFA analogues not only increase PUFA efficacy on activating the IKs channel, but their specific effects on IKs function can be modulated independently, either increasing the maximal conductance or voltage-shifting effect. Patients with LQTS can have mutations in the IKs channel that causes either a decrease in Gmax or a rightward shift of voltage dependence. By independently modulating PUFAs to either increase Gmax or cause a leftward shift in voltage dependence, we may be able to design therapies that are more personalized to each patient’s specific LQTS mutation. This novel mechanistic understanding of how aromatic PUFAs have these effects on the IKs channel may help to aid drug development for LQTS.
Materials and methods
Molecular biology
Request a detailed protocolKV7.1 and KCNE1 channel cRNA were transcribed using the mMessage mMachine T7 kit (Ambion). 50 ng of cRNA was injected at a 3:1, weight:weight (KV7.1:KCNE1) ratio into defolliculated X. laevis oocytes (Ecocyte, Austin, TX) for IKs channel expression. Injected cells were incubated for 72–96 hr in standard ND96 solution (96 mM NaCl, 2 mM KCl, 1 mM MgCl2, 1.8 mM CaCl2, 5 mM HEPES; pH = 7.5) containing 1 mM pyruvate at 16°C prior to electrophysiological recordings.
Primers for KV7.1 mutations:
R231Q/Q234R – cggccatcaggggTatccAAttTctgAGAatcctgagAatg
K326C – ccagacgtgggtcgggTGCaccatcgcctcctgcttc
S225A – caggtgtttgccacgGCCgcTatcaggggTatccgcttcc
Q220L – gtgggctccaagggAcTTgtgtttgccacgtcgg
T224V – ggggcaggtgtttgcAGTgtcggcTatcaggggcatc
S217A – gtcctctgcgtgggcGccaaggggcaggtgtttg
PUFA analogues and fluorinated PUFAs
Request a detailed protocolCommercially available PUFAs N-(α-linolenoyl) tyrosine (NALT) item number 10032 and linoleoyl phenalanine (Lin-phe) item number 20063 were obtained from Cayman Chemical (Ann Arbor, MI) or Larodan (Solna, Sweden). Linoleoyl tyrosine (Lin-tyr), docosahexaenoyl tyrosine (DHA-tyr), and pinolenoyl tyrosine (Pin-tyr) were synthesized as described previously (Bohannon et al., 2020). NAL-phe, 4Br-NAL-phe, 4F-NAL-phe, 3,4,5F-NAL-phe, and 3F-NALT were synthesized similarly, with detailed descriptions of the synthesis procedures for each compound provided in the supplemental methods. PUFA analogues were kept at –20°C as 100 mM stock solutions in ethanol except 4Br-NAL-phe, 4F-NAL-phe, 3,4,5F-NAL-phe, and 3F-NALT where stock solutions were prepared as needed on the day of recording. Serial dilutions of the different PUFAs were prepared from stocks to make 0.2 μM, 0.7 μM, 2.0 μM, 7.0 μM, and 20 μM concentrations in ND96 solutions (pH = 7.5). NALT is a commercially available compound. The other compounds used in this study were synthesized in small amounts. Thus, NALT is used as the focus of this study.
Two-electrode voltage-clamp (TEVC)
Request a detailed protocolX. laevis oocytes, co-expressing wild-type KV7.1 and KCNE1, were recorded in the two-electrode voltage-clamp (TEVC) configuration. Recording pipettes were filled with 3 M KCl. The recording chamber was filled with ND96 (96 mM NaCl, 2 mM KCl, 1 mM MgCl2, 1.8 mM CaCl2, 5 mM Tricine; pH 7.5). Dilutions of PUFAs and PUFA analogues were perfused into the recording chamber using the Rainin Dynamax Peristaltic Pump (Model RP-1) (Rainin Instrument Co., Oakland, CA). Electrophysiological recordings were obtained using Clampex 10.3 software (Axon, pClamp, Molecular Devices). During the application of PUFAs, the membrane potential was stepped every 30 s from –80 mV to 0 mV for 5 s before stepping to –40 mV and back to –80 mV to ensure that the PUFA effects on the current at 0 mV reached steady state (Figure 1D). A voltage-step protocol was used to measure the current vs. voltage (I–V) relationship before PUFA application and after the PUFA effects had reached steady state for each concentration of PUFA. Cells were held at –80 mV followed by a hyperpolarizing prepulse to –140 mV to make sure all channels are fully closed. The voltage was then stepped from –100 to 60 mV (in 20 mV steps) followed by a subsequent voltage step to –20 mV to measure tail currents before returning to the –80 mV holding potential.
Data analysis
Request a detailed protocolTail currents were analyzed using Clampfit 10.3 software in order to obtain conductance vs. voltage (G–V) curves to determine the voltage dependence of channel activation. V0.5, the voltage at which half the maximal current occurs, was obtained by fitting the G–V curves from each concentration of PUFA with a Boltzmann equation:
where Gmax is the maximal conductance at positive voltages and s is the slope factor in mV. The current values for each concentration at 0 mV (I/I0) were used to plot the dose–response curves for each PUFA. These dose–response curves were fit using the Hill equation to obtain the Km value for each PUFA:
where A is the fold increase in current caused by the PUFA at saturating concentrations, Km is the apparent affinity of the PUFA, x is the concentration, and n is the Hill coefficient. Fitted maximum values derived from the dose–response curves are reported for each of the effects (I/I0, ΔV0.5, and Gmax) from the different PUFAs tested. In some cases, there is variability in the V0.5 between batches of oocytes. In order to correct for variability due to oocytes, when the V0.5 was greatly different than 20 mV in control solution, we applied a correction in order to more accurately measure PUFA-induced IKs current increases. We subtracted the V0.5 (given by fitting the G–V with a Boltzmann equation) by 20 mV and used the current measured at the resulting voltage. The maximum conductance (Gmax) was calculated by taking the difference between the maximum and minimum current values (using the G–V curve for each concentration) and then normalizing to the value Gmax0 in control solution (0 μM). Graphs plotting mean and standard error of the mean (SEM) for I/I0, ΔV0.5, Gmax, and Km were generated using GraphPad Prism (GraphPad Software, La Jolla, CA).
Statistics
Unpaired t-tests and one-way ANOVA with multiple-comparisons statistics were computed using GraphPad Prism (GraphPad Software). Results were considered significant if p<0.05.
Materials availability statement
Request a detailed protocolMutations and newly synthesized PUFAs are available from the corresponding author upon reasonable request.
Data availability
References
-
Transcripts of Kv7.1 and Mink channels and slow delayed rectifier K+ current (I Ks) are expressed in Zebrafish (Danio rerio) heartPflügers Archiv - European Journal of Physiology 470:1753–1764.https://doi.org/10.1007/s00424-018-2193-1
-
Polyunsaturated fatty acids produce a range of activators for heterogeneous IKs channel dysfunctionThe Journal of General Physiology 152:e201912396.https://doi.org/10.1085/jgp.201912396
-
An electrostatic potassium channel opener targeting the final voltage sensor transitionThe Journal of General Physiology 137:563–577.https://doi.org/10.1085/jgp.201110599
-
Molecular movement of the voltage sensor in a K channelThe Journal of General Physiology 122:741–748.https://doi.org/10.1085/jgp.200308927
-
Cation-pi interactions involving aromatic amino acidsThe Journal of Nutrition 137:1504S–1508S.https://doi.org/10.1093/jn/137.6.1504S
-
Cation-Π interactions and their functional roles in membrane proteinsJournal of Molecular Biology 433:167035.https://doi.org/10.1016/j.jmb.2021.167035
-
The Biochemistry of N-3 polyunsaturated fatty acidsThe Journal of Biological Chemistry 277:8755–8758.https://doi.org/10.1074/jbc.R100062200
-
Polyunsaturated fatty acids as Modulators of Kv7 channelsFrontiers in Physiology 11:1–8.https://doi.org/10.3389/fphys.2020.00641
-
Effects of N-3 polyunsaturated fatty acids on cardiac ion channelsFrontiers in Physiology 3:245.https://doi.org/10.3389/fphys.2012.00245
-
Marine N-3 Pufas modulate Iks gating, Channel expression, and location in membrane MicrodomainsCardiovascular Research 105:223–232.https://doi.org/10.1093/cvr/cvu250
-
Molecular physiology of cardiac RepolarizationPhysiological Reviews 85:1205–1253.https://doi.org/10.1152/physrev.00002.2005
-
The role of S4 charges in voltage-dependent and voltage-independent Kcnq1 potassium channel complexesThe Journal of General Physiology 129:121–133.https://doi.org/10.1085/jgp.200609612
-
Asymmetric functional contributions of acidic and aromatic side chains in sodium channel voltage-sensor domainsThe Journal of General Physiology 143:645–656.https://doi.org/10.1085/jgp.201311036
-
Regulation of voltage-activated K(+) channel gating by Transmembrane Β subunitsFrontiers in Pharmacology 3:63.https://doi.org/10.3389/fphar.2012.00063
-
Update on the diagnosis and management of familial long QT syndromeHeart, Lung and Circulation 25:769–776.https://doi.org/10.1016/j.hlc.2016.01.020
-
Mink-Kvlqt1 fusion proteins, evidence for multiple Stoichiometries of the assembled I(sK) channelThe Journal of Biological Chemistry 273:34069–34074.https://doi.org/10.1074/jbc.273.51.34069
-
Kcne1 Remodels the voltage sensor of Kv7.1 to modulate channel functionBiophysical Journal 99:3599–3608.https://doi.org/10.1016/j.bpj.2010.10.018
-
Single-channel properties of Iks potassium channelsJournal of General Physiology 112:665–678.https://doi.org/10.1085/jgp.112.6.665
-
Identification of Pufa interaction sites on the cardiac potassium channel Kcnq1The Journal of General Physiology 153:e202012850.https://doi.org/10.1085/jgp.202012850
Article and author information
Author details
Funding
HORIZON EUROPE European Research Council (850622)
- Sara I Liin
Swedish Research Council (2021-01885)
- Sara I Liin
National Institutes of Health (R01HL131461)
- H Peter Larsson
The funders had no role in study design, data collection and interpretation, or the decision to submit the work for publication.
Acknowledgements
This work was supported by the Swedish Research Council (2021-01885 to SIL), the European Research Council (ERC) under the European Union’s Horizon 2020 research and innovation program (grant agreement no. 850622 to SIL), and the National Institutes of Health (R01HL131461 to HPL). We thank Jason D Galpin and Christopher A Ahern (University of Iowa; R24 NS104617-04; the Facility for Atomic Mutagenesis) and Xiongyu Wu (Linkoping University) for synthesis of different aromatic PUFAs. DPT acknowledges support from the Canada Research Chairs program.
Copyright
© 2023, Bohannon, Jowais et al.
This article is distributed under the terms of the Creative Commons Attribution License, which permits unrestricted use and redistribution provided that the original author and source are credited.
Metrics
-
- 650
- views
-
- 106
- downloads
-
- 10
- citations
Views, downloads and citations are aggregated across all versions of this paper published by eLife.
Citations by DOI
-
- 10
- citations for umbrella DOI https://doi.org/10.7554/eLife.85773