Splicing factor SRSF1 is essential for homing of precursor spermatogonial stem cells in mice
eLife assessment
In this valuable study, the authors characterize the role of splicing factor SRSF1 during spermatogenesis with a conditional knockout of Srsf1 in male germ cells. The phenotype and molecular role of SRSF1 in regulating alternative splicing in precursor spermatogonial stem cells in juvenile testes are convincingly supported. The paper also provides convincing evidence that the mRNA encoding Tial, a factor relevant to spermatogonial maintenance and male fertility, is alternatively spliced in testis and that this splicing is regulated by SRSF1. The work will be of interest to the fields of reproductive biology, stem cell biology, and alternative splicing.
https://doi.org/10.7554/eLife.89316.4.sa0Valuable: Findings that have theoretical or practical implications for a subfield
- Landmark
- Fundamental
- Important
- Valuable
- Useful
Convincing: Appropriate and validated methodology in line with current state-of-the-art
- Exceptional
- Compelling
- Convincing
- Solid
- Incomplete
- Inadequate
During the peer-review process the editor and reviewers write an eLife Assessment that summarises the significance of the findings reported in the article (on a scale ranging from landmark to useful) and the strength of the evidence (on a scale ranging from exceptional to inadequate). Learn more about eLife Assessments
Abstract
Spermatogonial stem cells (SSCs) are essential for continuous spermatogenesis and male fertility. The underlying mechanisms of alternative splicing (AS) in mouse SSCs are still largely unclear. We demonstrated that SRSF1 is essential for gene expression and splicing in mouse SSCs. Crosslinking immunoprecipitation and sequencing data revealed that spermatogonia-related genes (e.g. Plzf, Id4, Setdb1, Stra8, Tial1/Tiar, Bcas2, Ddx5, Srsf10, Uhrf1, and Bud31) were bound by SRSF1 in the mouse testes. Specific deletion of Srsf1 in mouse germ cells impairs homing of precursor SSCs leading to male infertility. Whole-mount staining data showed the absence of germ cells in the testes of adult conditional knockout (cKO) mice, which indicates Sertoli cell-only syndrome in cKO mice. The expression of spermatogonia-related genes (e.g. Gfra1, Pou5f1, Plzf, Dnd1, Stra8, and Taf4b) was significantly reduced in the testes of cKO mice. Moreover, multiomics analysis suggests that SRSF1 may affect survival of spermatogonia by directly binding and regulating Tial1/Tiar expression through AS. In addition, immunoprecipitation mass spectrometry and co-immunoprecipitation data showed that SRSF1 interacts with RNA splicing-related proteins (e.g. SART1, RBM15, and SRSF10). Collectively, our data reveal the critical role of SRSF1 in spermatogonia survival, which may provide a framework to elucidate the molecular mechanisms of the posttranscriptional network underlying homing of precursor SSCs.
Introduction
Sertoli cell-only syndrome (SCOS), also known as del Castillo syndrome or germ cell aplasia, is one of the most common causes of severe non-obstructive azoospermia (NOA) (Wang et al., 2023). SCOS is the presence of only Sertoli cells in the testicular tubules of the testes, with no germ cells present (Juul et al., 2014; Wang et al., 2023). It is well known that abnormal self-renewal and differentiation of spermatogonial stem cells (SSCs) lead to SCOS (Kanatsu-Shinohara and Shinohara, 2013; La and Hobbs, 2019). In mice, gonocytes begin homing at 0–3 days postpartum (dpp) and then develop into SSCs at 4–6 dpp for continuous self-renewal and differentiation (Lee and Shinohara, 2011; McLean et al., 2003; Tan and Wilkinson, 2020). The mechanisms regulating homing of precursor SSCs are hence crucial for forming SSC pools and establishing niches (Oatley and Brinster, 2012). Spermatogonia migrate to form two distinct subtypes in mice. The first subtype develops into precursor SSCs that provide an SSC population for adult spermatogenesis, whereas the second subtype transitions directly to differentiated spermatogonia that contribute to the first round of spermatogenesis but do not self-renew (Kluin and de Rooij, 1981; Law et al., 2019). Therefore, homing of precursor SSCs to establish niches is essential for SSC self-renewal and differentiation.
Many transcription factors (e.g. FOXO1, PLZF, POU5F1, TAF4B, CHD4, BCL6B, BRACHYURY, ETV5, ID4, LHX1, POU3F1, DMRT1, NGN3, SOHLH1, SOHLH2, SOX3, and STAT3) promote SSC self-renewal and differentiation (Cafe et al., 2021; Song and Wilkinson, 2014). However, the molecular mechanisms of the posttranscriptional network underlying homing of precursor SSCs are not sufficiently clear. Previous studies have identified the key RNA-binding proteins DND1 and DDX5 in SSCs with a unique and dominant role in posttranscriptional regulation (Legrand et al., 2019; Yamaji et al., 2017). Surprisingly, recent studies have found that the RNA-binding proteins SRSF10, UHRF1, BUD31, and BCAS2 regulate alternative splicing (AS) in mouse spermatogonia (Liu et al., 2022; Liu et al., 2017; Qin et al., 2023; Zhou et al., 2022). It is well known that testes are rich in AS events (Mazin et al., 2021; Venables, 2002). Thus, understanding the mechanisms of AS in human reproduction can provide new insights into clinical diagnosis. However, the underlying mechanisms of how AS functions in homing of precursor SSCs are still largely unclear.
Serine/arginine-rich splicing factor 1 (SRSF1, previously SF2/ASF) is a widely studied and important splicing factor involved in cancer progression, heart development, and thymus development (Du et al., 2021; Katsuyama et al., 2019; Katsuyama and Moulton, 2021; Liu et al., 2021; Lv et al., 2021; Qi et al., 2021; Xu et al., 2005). Our previous work has shown that SRSF1 deficiency impairs primordial follicle formation during meiotic prophase I and leads to primary ovarian insufficiency (Sun et al., 2023b). However, the underlying mechanisms by which SRSF1 regulates pre-mRNA splicing in mouse SSCs remain unknown. A mouse model with Srsf1 conditional deletion can effectively address this uncertainty. This study showed that specific deletion of Srsf1 in mouse germ cells leads to NOA by impairing homing of mouse precursor SSCs. We further verified that SRSF1 directly binds and regulates Tial1/Tiar expression via AS, which may be critical for homing of mouse precursor SSCs.
Results
SRSF1 may have a vital role in posttranscriptional regulation in the testes
To investigate the role of SRSF1 in spermatogenesis, the dynamic localisation of SRSF1 in the testis was evaluated. Fascinatingly, the results of SRSF1 and γH2AX co-staining revealed that SRSF1 was expressed during spermatogenesis (Figure 1A and Figure 1—figure supplement 1). RT-qPCR and western blotting results showed that the expression of SRSF1 fluctuated during the developmental stages of the testes (Figure 1B and C). Concurrently, the results of SRSF1 and PLZF co-staining revealed that SRSF1 was highly expressed in the nuclei of spermatogonia (Figure 1D). To further explore the function of SRSF1 in regulating SSC self-renewal and differentiation, crosslinking immunoprecipitation and sequencing (CLIP-seq) was performed in adult mouse testes (Sun et al., 2023a). Gene Ontology (GO) enrichment analyses of the SRSF1 peak-containing genes revealed that spermatogenesis-related genes were regulated by SRSF1 (Figure 2A and Supplementary file 1). In combination with previous studies, we found that spermatogonia-related genes (e.g. Plzf, Id4, Setdb1, Stra8, Tial1/Tiar, Bcas2, Ddx5, Srsf10, Uhrf1, and Bud31) were bound by SRSF1. To provide in-depth insight into the binding of spermatogonia-associated genes, the SRSF1-binding peaks of the gene transcripts were shown by using Integrative Genomics Viewer (IGV) (Figure 2B). The co-staining results showed localisation and expression of the spermatogonia-related proteins in mouse testes (Figure 2C). Together, these results suggested that SRSF1 may have a vital role in posttranscriptional regulation in the testes, particularly during spermatogonial development.
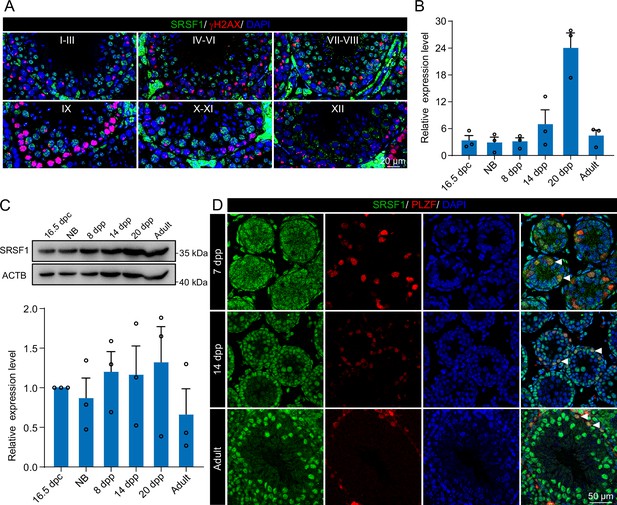
Expression and localisation of SRSF1 in the testis of mice at different developmental stages.
(A) Dynamic localisation of SRSF1 during spermatogenesis. Co-immunostaining was performed using SRSF1 and γH2AX antibodies in adult mouse testes. DNA was stained with DAPI. Scale bar, 20 μm. (B) Expression of Srsf1 in testes at different stages of development. The RT-qPCR data were normalised to Gapdh. N=3. (C) Western blotting of SRSF1 expression in testes at different stages of development. ACTB served as a loading control. The value in 16.5 days post-coitus (dpc) testes were set as 1.0, and the relative values of testes in other developmental periods are indicated. N=3. (D) Localisation and expression of SRSF1 in spermatogonia. Co-immunostaining was performed using PLZF and SRSF1 antibodies in 7 days postpartum (dpp), 14 dpp, and adult mouse testes. DNA was stained with DAPI. Arrowheads, spermatogonia. Scale bar, 50 μm.
-
Figure 1—source data 1
Western blotting of SRSF1 expression in testes at different stages of development.
ACTB served as a loading control.
- https://cdn.elifesciences.org/articles/89316/elife-89316-fig1-data1-v1.pdf
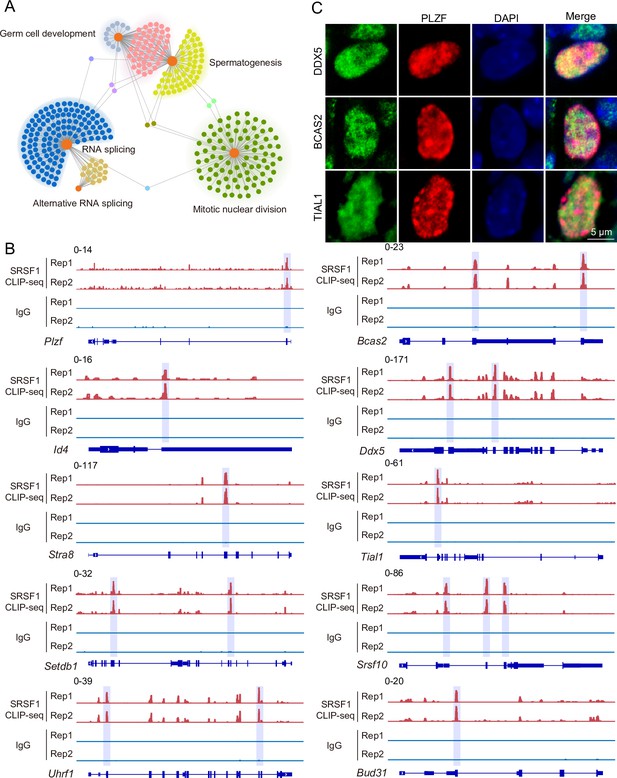
SRSF1-binding genes have an essential role in spermatogonia.
(A) Network showing Gene Ontology (GO) enrichment analyses of SRSF1-binding genes. (B) Representative genome browser views of spermatogonia-related gene transcripts bound by SRSF1. Higher peaks are marked by a lavender area. (C) Localisation of the spermatogonia-related proteins in mouse testes. Scale bar, 5 μm.
SRSF1 deficiency leads to SCOS
To define the specific involvement of SRSF1 in spermatogonia, we studied the physiological roles of SRSF1 in vivo using a mouse model. Considering that global Srsf1 knockout is lethal in mice (Xu et al., 2005), we used a conditional allele of Srsf1 (Srsf1Fl) in which exons 2, 3, and 4 of Srsf1 are flanked by two loxP sites (Figure 3A). By crossing Srsf1Fl and Vasa-Cre mice, we obtained Vasa-Cre Srsf1Fl/del mice with Srsf1 deletion in germ cells (Figure 3A and B). We verified the absence of the SRSF1 protein in germ cells by co-immunofluorescence analyses with SRSF1 and PLZF antibodies (Figure 3C). Subsequently, the breeding experiment indicated that conditional knockout (cKO) mice had a standard mating capacity but that the absence of Srsf1 led to complete infertility in males (Figure 3D). Histological examination of cKO epididymides revealed that sperm could not be found in the cauda epididymis (Figure 3E). Considering the limitations of sectioning, the cauda epididymal sperm count further validated this conclusion (Figure 3F). It was clear that spermatogenesis in the testes was severely impaired. Therefore, we focused our attention on the testes. The adult cKO mice were normal in size (Figure 3G). However, the sizes of cKO mouse testes were significantly reduced (Figure 3H). Histological examination of cKO testis sections showed that no germ cells could be visualised, and only a large number of Sertoli cells were observed in the testes of cKO mice (Figure 3I). Together, these results demonstrated that SRSF1 is critical for spermatogenesis and that its absence leads to SCOS.
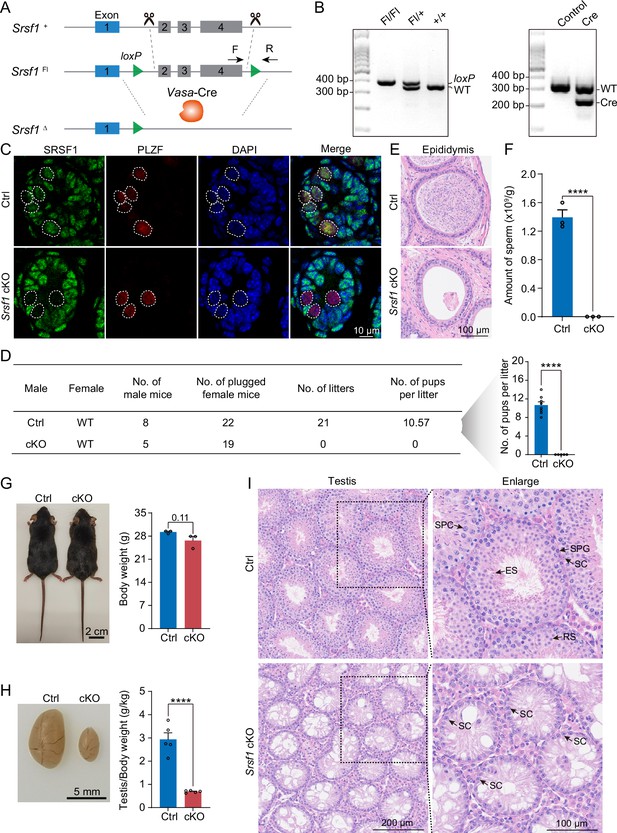
SRSF1 plays critical roles in spermatogenesis and male fertility.
(A) Vasa-Cre mice were crossed with Srsf1Fl/Fl mice to generate Srsf1 conditional knockout (cKO) mice. Cre-mediated deletion removed exons 2, 3, and 4 of Srsf1 and generated a null protein allele. (B) Genotyping PCR was performed using Vasa-Cre and Srsf1 primers. (C) Co-immunostaining of SRSF1 and PLZF in 7 days postpartum (dpp) control (Ctrl) and cKO testis. DNA was stained with DAPI. Scale bar, 10 μm. (D) Fertility test results showed a male infertility phenotype in the cKO mice (N=5) compared to the Ctrl mice (N=8). The number of pups per litter was determined in the cKO (N=5) and Ctrl (N=8) mice. (E) Haematoxylin-eosin-stained epididymis sections from adult Ctrl and cKO mice were obtained. Scale bar, 100 μm. (F) Cauda epididymal sperm counting was performed. N=3. (G) Normal body weight in cKO mice. The body sizes and weights of adult Ctrl and cKO mice are shown as the mean ± SEM. N=3. (H) Testis atrophy in adult cKO mice. Testis sizes and weights/body of adult Ctrl and cKO mice are shown as the mean ± SEM. N=5. (I) Haematoxylin-eosin-stained testis sections from adult Ctrl and cKO mice were obtained. Scale bar, left panel: 200 μm, right panel: 100 μm. SC, Sertoli cell; SPG, spermatogonium; SPC, spermatocyte; RS, round spermatid; ES, elongated spermatid. Unpaired Student’s t-test determined significance; exact p value p ≥ 0.05, ****p < 0.0001. The points and error bars represent the mean ± SEM.
-
Figure 3—source data 1
Genotyping PCR was performed using Vasa-Cre and Srsf1 primers.
- https://cdn.elifesciences.org/articles/89316/elife-89316-fig3-data1-v1.pdf
Loss of SRSF1 impairs spermatogonia survival
To further confirm the absence of germ cells in the testes of cKO mice, PLZF and γH2AX co-staining was performed in adult mouse testes. These data suggested that SRSF1 deficiency impaired germ cell survival (Figure 4A). The results of VASA and TRA98 co-staining further confirmed this phenotype (Figure 4B). Considering the limitations of sectioning, we used whole-mount immunostaining to perform a comprehensive analysis and found that germ cells were indeed absent in the testes of adult cKO mice (Figure 4C). To dynamically analyse the loss of germ cells, we collected testes from 5 dpp, 7 dpp, and 14 dpp mice. Morphological results showed that the testes of 7 dpp and 14 dpp cKO mice were much smaller than those of Ctrl mice (Figure 5A). To determine the presence of germ cells in cKO testes, VASA staining was performed in 5 dpp, 7 dpp, and 14 dpp Ctrl and cKO testes. The results showed that germ cells were still present in cKO mice but were significantly reduced in 7 dpp and 14 dpp cKO testes (Figure 5B). The quantifications of germ cells per tubule showed a significant reduction in the number of 7 dpp and 14dpp cKO testes, especially 14 dpp cKO testes (Figure 5B). In addition, TUNEL results showed that apoptosis significantly increased in cKO testes (Figure 5C). These data suggested that the absence of SRSF1 causes apoptosis in a large number of spermatogonia that are unable to survive.
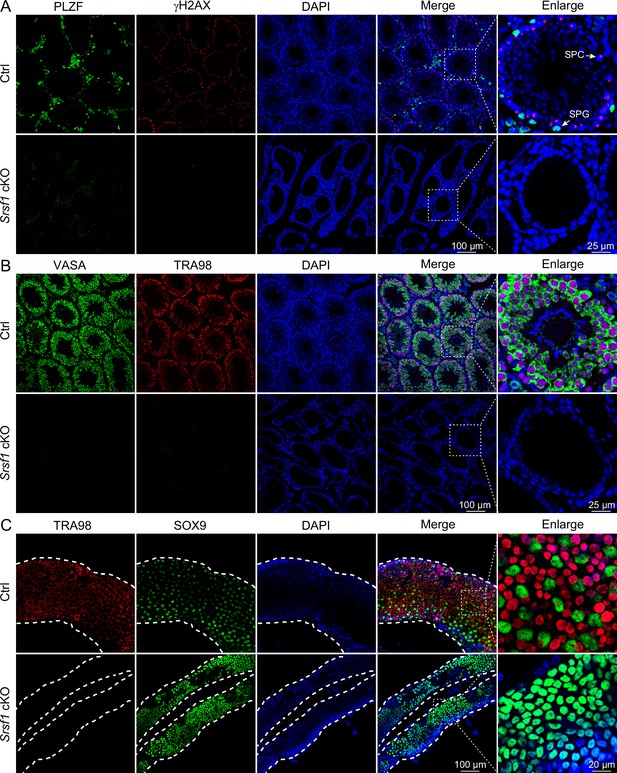
Loss of germ cells in adult conditional knockout (cKO) mouse testes.
(A) Co-immunostaining of PLZF and γH2AX in adult control (Ctrl) and cKO testis. DNA was stained with DAPI. Scale bar, right panel: 25 μm, other panels: 100 μm. (B) Co-immunostaining of VASA and TRA98 in adult Ctrl and cKO testis. DNA was stained with DAPI. Scale bar, right panel: 25 μm, other panels: 100 μm. (C) Whole-mount co-immunostaining of TRA98 and SOX9 in adult Ctrl and cKO testis. DNA was stained with DAPI. White dashed lines, boundary of the tubule. Scale bar, right panel: 20 μm, other panels: 100 μm.
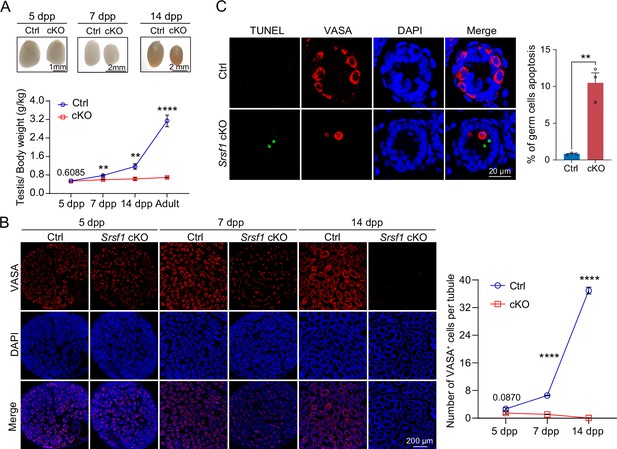
SRSF1 is required for spermatogonia survival.
(A) Testis sizes of 5 days postpartum (dpp), 7 dpp, and 14 dpp control (Ctrl) and conditional knockout (cKO) mice are shown. The testis/body weight ratios (g/kg) of 5 dpp, 7 dpp, 14 dpp, and adult Ctrl and cKO mice are shown as the mean ± SEM. N=4. (B) Immunostaining of VASA in 5 dpp, 7 dpp, and 14 dpp Ctrl and cKO testis. DNA was stained with DAPI. Scale bar, 200 μm. Number of VASA-positive cells per tubule is the mean ± SEM. N=3. (C) TUNEL apoptosis assay was performed on sections from 7 dpp Ctrl and cKO testis. DNA was stained with DAPI. Scale bar, 20 μm. Percentage of germ cells apoptosis is the mean ± SEM. N=3. Unpaired Student’s t-test determined significance; exact p value p ≥ 0.05, **p < 0.01, ****p < 0.0001. The points and error bars represent the mean ± SEM.
Loss of SRSF1 impairs homing of precursor SSCs
To further investigate the reason for the failure of spermatogonia to survive, we observed homing of precursor SSCs in the testes of mice at 5 dpp. Interestingly, the results of VASA and SOX9 co-staining showed that partial germ cells could not complete homing in 5 dpp cKO testes (Figure 6A and B). In mice, starting at 3 dpp, cytoplasmic FOXO1 in some gonocytes begins to enter the nucleus (Goertz et al., 2011). These cells further develop into prospermatogonia, which are expected to develop into SSCs (Goertz et al., 2011). Thus, immunohistochemical staining for FOXO1 was performed on 5 dpp mouse testis sections (Figure 6C). Further, germ cell statistics of FOXO1 expression in the nucleus showed a reduced number of prospermatogonia in cKO mice (Figure 6D). And germ cells in which FOXO1 is expressed in the nucleus similarly undergo abnormal homing (Figure 6E). Thus, all the above data indicated that SRSF1 has an essential role in the homing of precursor SSCs.
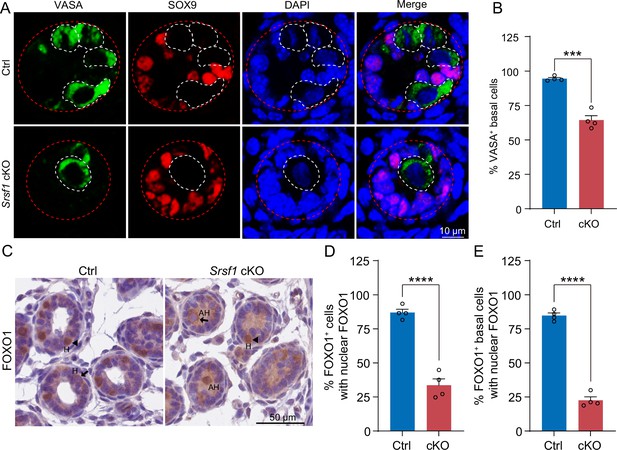
SRSF1 is required for homing of precursor spermatogonial stem cells (SSCs).
(A) Co-immunostaining of VASA and SOX9 in 5 days postpartum (dpp) control (Ctrl) and conditional knockout (cKO) testis. DNA was stained with DAPI. Scale bar, 10 μm. Red dashed circles, tubule. White dashed circles, germ cell. (B) The percentage of VASA-positive basal cells is shown as the mean ± SEM. N=4. (C) Immunohistochemical staining of FOXO1 in 5 dpp Ctrl and cKO testis. The nuclei were stained with haematoxylin. Scale bar, 10 μm. Arrowheads, FOXO1 in the nucleus. Arrows, FOXO1 in the cytoplasm. H, homing. AH, abnormal homing. (D) The percentage of FOXO1-positive cells with nuclear FOXO1 is shown as the mean ± SEM. 913 Ctrl germ cells and 592 cKO germ cells were obtained from four animals. (E) The percentage of FOXO1-positive basal cells with nuclear FOXO1 is shown as the mean ± SEM. 913 Ctrl germ cells and 592 cKO germ cells were obtained from four animals. Unpaired Student’s t-test determined significance; ***p < 0.001, ****p < 0.0001. The points and error bars represent the mean ± SEM.
SRSF1 is essential for gene expression in spermatogonia
To investigate the molecular mechanisms of SRSF1 in spermatogonia, we isolated mRNA from 5 dpp cKO and Ctrl mouse testes and performed RNA-seq (Figure 7—figure supplement 1). RNA-seq and RT-qPCR results showed a significant reduction in the expression of Srsf1 in 5 dpp cKO mouse testes (Figure 7A). Western blotting results showed that SRSF1 expression was significantly reduced in the testes of cKO mice at 5 dpp (Figure 7B). Hence, for Ctrl and cKO samples, the confidence level of the RNA-seq data was high. The volcano map and cluster heatmap showed 715 downregulated and 258 upregulated genes identified by RNA-seq data in 5 dpp cKO mouse testes (Figure 7C and D and Supplementary file 2). These gene GO enrichment analyses indicated abnormal germ cell development and cell cycle arrest in 5 dpp cKO mouse testes (Figure 7E). Surprisingly, the heatmap showed that spermatogonia-associated gene (e.g. Gfra1, Pou5f1, Plzf, Nanos3, Dnd1, Stra8, and Taf4b) expression was significantly reduced in the testes of cKO mice at 5 dpp (Figure 7F). Simultaneously, the tracks of differentially expressed genes are demonstrated by using IGV (Figure 7G). Next, we validated the abnormal expression of spermatogonia-associated genes (downregulated: Gfra1, Pou5f1, Plzf, Dnd1, Stra8, and Taf4b; unchanged: Nanos3) by RT-qPCR (Figure 7H). Together, these data indicated that germ cell-specific deletion of Srsf1 impairs the expression of spermatogonia-associated genes.
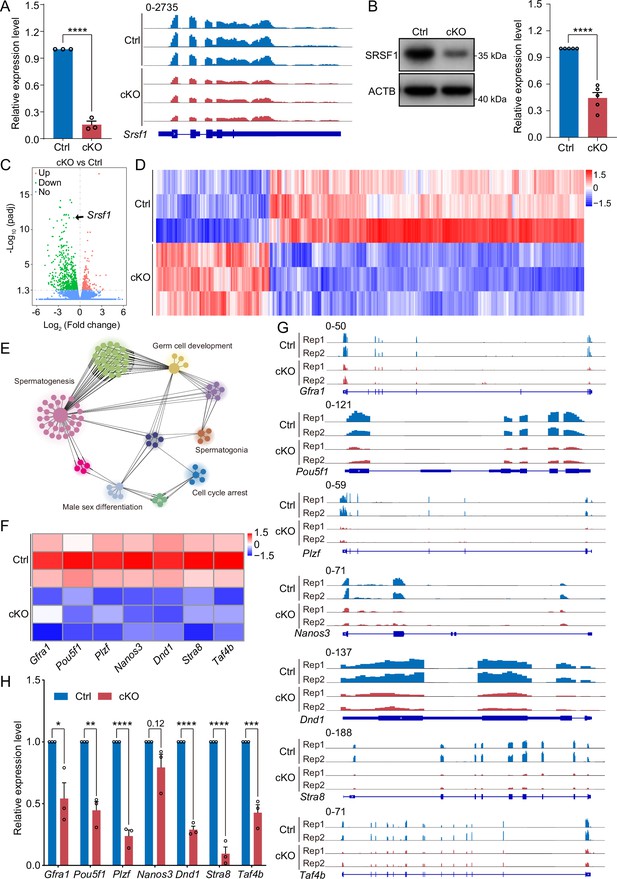
SRSF1 regulates the expression of spermatogonia-related genes.
(A) Expression of Srsf1 in 5 days postpartum (dpp) control (Ctrl) and conditional knockout (cKO) mouse testis. The RT-qPCR data were normalised to Gapdh. N=5. The expression of Srsf1 is shown as reading coverage in 5 dpp mouse testis. (B) Western blotting of SRSF1 expression in 5 dpp mouse testis. ACTB served as a loading control. The value in Ctrl testes was set as 1.0, and the relative values in cKO testis are indicated. N=5. (C) Volcano map displaying the distribution of differentially expressed genes from RNA-seq data. The abscissa in the figure represents the gene fold change in 5 dpp cKO and Ctrl mouse testis. |log2FoldChange|≥0. The ordinate indicates the significance of gene expression differences between 5 dpp cKO and Ctrl mouse testis. padj ≤0.05. Upregulated genes are shown as red dots, and downregulated genes are shown as green dots. (D) Cluster heatmap of differentially expressed genes. The ordinate is the genotype, and the abscissa is the normalised FPKM (fragments per kilobase million) value of the differentially expressed gene. Red indicates a higher expression level, while blue indicates a lower expression level. (E) Network showing Gene Ontology (GO) enrichment analyses of differentially expressed genes. (F) Heatmap of spermatogonia-related gene expression. (G) The expression of spermatogonia-related genes is shown as read coverage. (H) The expression of spermatogonia-related genes in 5 dpp cKO and Ctrl mouse testis. The RT-qPCR data were normalised to Gapdh. The value in the Ctrl group was set as 1.0, and the relative value in the cKO group is indicated. N=3. Unpaired Student’s t-test determined significance; exact p value p ≥ 0.05, *p < 0.05, **p < 0.01, ***p < 0.001, ****p < 0.0001. The points and error bars represent the mean ± SEM.
-
Figure 7—source data 1
Western blotting of SRSF1 expression in 5 days postpartum (dpp) mouse testes.
ACTB served as a loading control.
- https://cdn.elifesciences.org/articles/89316/elife-89316-fig7-data1-v1.pdf
SRSF1 directly binds and regulates the expression and AS of Tial1/Tiar
Multiomics analyses were carried out in a subsequent study to identify the molecular mechanisms by which SRSF1 regulates spermatogonia survival. Considering that the CLIP-seq data were obtained from adult mouse testis, the set of genes bound by CLIP-seq was restricted to those expressed only in the 5 dpp mouse testis RNA-seq data. we found that 3543 of the 4824 genes bound by SRSF1 were expressed in testes at 5 dpp. Venn diagram data revealed that 9 out of 715 downregulated genes were bound by SRSF1 and underwent abnormal AS (Figure 8A). And 1 out of 258 upregulated genes was bound by SRSF1 and underwent abnormal AS (Figure 8A). Interestingly, we found that 39 unchanged genes were bound by SRSF1 and underwent abnormal AS (Figure 8A). The AS genes were subsequently investigated in 5 dpp cKO mouse testes using transcriptomic analyses. RNA-seq analyses showed that 162 AS events were significantly affected (false discovery rate [FDR] <0.05) in cKO mouse testes (Figure 8B and C and Supplementary file 3). Most of the 133 affected AS events (162) were classified as skipped exons (SEs), with 10 AS events categorised as retained introns (RIs), 13 as mutually exclusive exons (MXEs), 4 as alternative 5' splice sites (A5SSs), and 2 as alternative 3' splice sites (A3SSs) (Figure 8C). Additionally, the overall analysis of aberrant AS events showed that SRSF1 effectively inhibits the occurrence of SE and MXE events and promotes the occurrence of RI events (Figure 8C). Then, GO enrichment analyses of AS genes revealed that four genes concerning germ cell development were altered in AS forms (Figure 8D). It has been shown that Tial1/Tiar affects the survival of primordial germ cells (Beck et al., 1998). Moreover, Tial1/Tiar is 1 of 39 unchanged genes that are bound by SRSF1 and undergo abnormal AS. Thus, multiomics analyses suggested that Tial1/Tiar were posttranscriptionally regulated by SRSF1. Next, we investigated the mechanism by which SRSF1 regulates the AS of Tial1/Tiar, RT-PCR results showed that the pre-mRNA of Tial1/Tiar in 5 dpp cKO mouse testes exhibited abnormal AS (Figure 8E). We then visualised the different types of AS based on RNA-seq data by using IGV (Figure 8F and Figure 8—figure supplement 1). The results of RNA immunoprecipitation (RIP)-qPCR showed that SRSF1 could bind to the pre-mRNA of Tial1/Tiar (Figure 8G). Interestingly, RNA-seq analyses showed that the fragments per kilobase million (FPKM) of Tial1/Tiar was unchanged in 5 dpp cKO mouse testes (Figure 8H). RT-qPCR results showed that Tial1/Tiar transcript levels were not inhibited (Figure 8I). However, western blotting showed that expression levels of TIAL1/TIAR isoform X2 were significantly suppressed (Figure 8J and Figure 8—figure supplement 1). In summary, the data indicate that SRSF1 is required for TIAL1/TIAR expression and splicing in spermatogonia survival.
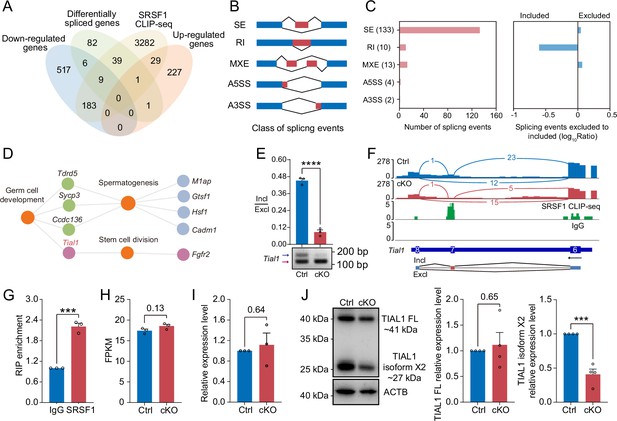
SRSF1 directly binds and regulates the expression and alternative splicing (AS) of Tial1/Tiar.
(A) Venn diagram showing the correlation among downregulated, upregulated, alternatively spliced, and SRSF1-binding genes. (B) Schematic diagram showing the classes of splicing events. (C) Splicing events were analysed by number, exclusion, and inclusion. (D) Network showing Gene Ontology (GO) enrichment analyses of AS genes. (E) The AS of Tial1/Tiar in 5 days postpartum (dpp) Ctrl and conditional knockout (cKO) mouse testes was analysed by RT-PCR. N=3. The ratio of inclusion (Incl) to exclusion (Excl) is shown accordingly. (F) Analyses of Tial1/Tiar expression and exon-exon junctions were performed. (G) SRSF1 directly binds the pre-mRNA of Tial1/Tiar by RNA immunoprecipitation (RIP)-qPCR in 5 dpp mouse testes. N=3. (H) The fragments per kilobase million (FPKM) of Tial1/Tiar in 5 dpp Ctrl and cKO mouse testes. (I) The expression of Tial1/Tiar in 5 dpp Ctrl and cKO mouse testes. The RT-qPCR data were normalised to Gapdh. The value in the Ctrl group was set as 1.0, and the relative value in the cKO group is indicated. N=3. (J) Western blotting of TIAL1/TIAR expression in 5 dpp mouse testes. ACTB served as a loading control. The FL/isoform X2 value of TIAL1/TIAR in the Ctrl group was set as 1.0, and the relative value in the cKO group is indicated. N=3. FL, full length. Unpaired Student’s t-test determined significance; exact p value p ≥ 0.05, ***p < 0.001, ****p < 0.0001. The points and error bars represent the mean ± SEM.
-
Figure 8—source data 1
The alternative splicing (AS) of Tial1/Tiar in 5 days postpartum (dpp) Ctrl and conditional knockout (cKO) mouse testes was analysed by RT-PCR.
- https://cdn.elifesciences.org/articles/89316/elife-89316-fig8-data1-v1.pdf
-
Figure 8—source data 2
Western blotting of TIAL1/TIAR expression in 5 days postpartum (dpp) mouse testes.
ACTB served as a loading control.
- https://cdn.elifesciences.org/articles/89316/elife-89316-fig8-data2-v1.pdf
SRSF1 recruits AS-related proteins to modulate AS in testes
To identify the interacting proteins for which SRSF1 exerts its AS role, we performed MS analyses of IP samples from 5 dpp mouse testis extracts. The silver-stained gel of SRSF1 and normal IgG showed several SRSF1-interacting proteins from 5 dpp mouse testis extracts (Figure 9A). The IP results indicated that SRSF1 was able to effectively IP the testis extracts of 5 dpp mice (Figure 9B). Immunoprecipitation mass spectrometry (IP-MS) data demonstrated the efficient enrichment of SRSF1 (Figure 9C and Supplementary file 4). These data showed that the two samples were highly reproducible, especially for SRSF1 (Figure 9D). Then, GO enrichment analyses of the IP proteins revealed that AS-related proteins could interact with SRSF1 (Figure 9E). A circular heatmap showed that SRSF1 could interact with AS-related proteins (e.g. SRSF10, SART1, RBM15, SRRM2, SF3B6, and SF3A2) (Figure 9F). The co-immunoprecipitation (Co-IP) results indicated that FLAG-SRSF1 interacted with HA-SART1, HA-RBM15, and HA-SRSF10 in 293T cells (Figure 9G). In addition, the fluorescence results showed complete co-localisation of mCherry-SRSF1 with eGFP-SART1, eGFP-RBM15, and eGFP-SRSF10 in 293T cells (Figure 9H). Co-IP suggested that the RRM1 domain of SRSF1 interacted with HA-SART1, HA-RBM15, and HA-SRSF10 in 293T cells (Figure 9I). Determining the complex structures of these interactions is valuable, in which molecular docking has played an important role (Yan et al., 2017). HDOCK is a novel web server of our hybrid docking algorithm of template-based modelling and free docking (Yan et al., 2017). The HDOCK analysis results depicted the RRM1 domain of SRSF1 with SRSF10, SART1, and RBM15 docking based on a hybrid strategy (Figure 9J). Together, the above data show that SRSF1 may interact with SRSF10, SART1, and RBM15 to regulate AS in 5 dpp mouse testes.
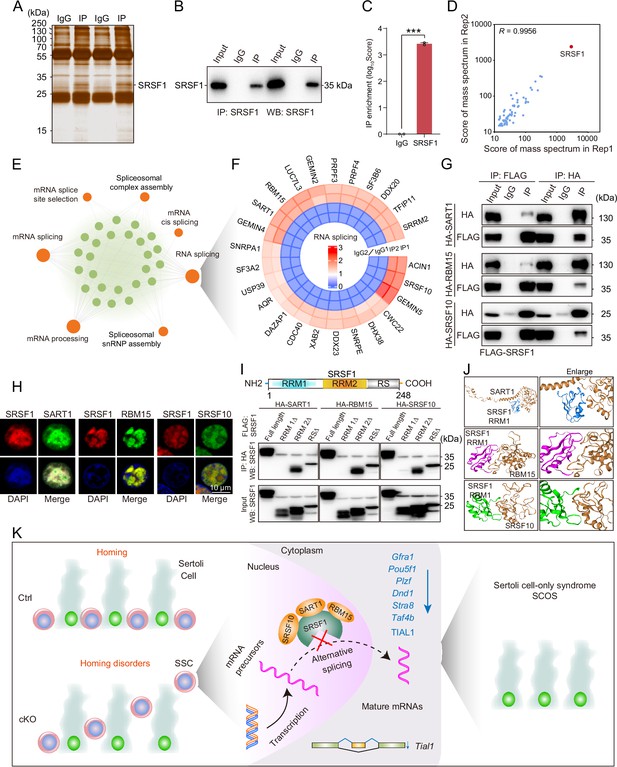
SRSF1 recruits alternative splicing (AS)-related proteins to modulate AS in testes.
(A) Silver-stained gel of SRSF1 and control immunoprecipitates from 5 days postpartum (dpp) mouse testis extracts. (B) IP experiment was performed in 5 dpp mouse testis extracts. (C) IP of SRSF1 from immunoprecipitation mass spectrometry (IP-MS) data. (D) Pearson’s correlation analysis showed the coefficient between the two samples for IP-MS data. (E) Network showing Gene Ontology (GO) enrichment analyses of SRSF1-binding proteins. (F) Circular heatmap of AS-related proteins. (G) Co-immunoprecipitation (Co-IP) experiment for detecting the SRSF1 association between SART1, RBM15, and SRSF10 in 293T cells. (H) SRSF1-mCherry cotransfected with SART1-eGFP, RBM15-eGFP, and SRSF10-eGFP 293T cells is shown. DNA was stained with DAPI. Scale bar, 10 μm. (I) Co-IP experiment for detecting the SRSF1-truncated protein association between SART1, RBM15, and SRSF10 in 293T cells. (J) A schematic diagram of protein interactions is shown. (K) Schematic illustration of the molecular mechanisms by which SRSF1 regulates homing of mouse precursor spermatogonial stem cells (SSCs).
-
Figure 9—source data 1
IP experiment was performed in 5 days postpartum (dpp) mouse testis extracts.
- https://cdn.elifesciences.org/articles/89316/elife-89316-fig9-data1-v1.pdf
-
Figure 9—source data 2
Co-immunoprecipitation (Co-IP) experiment for detecting the SRSF1 association between SART1, RBM15, and SRSF10 in 293T cells.
- https://cdn.elifesciences.org/articles/89316/elife-89316-fig9-data2-v1.pdf
-
Figure 9—source data 3
Co-immunoprecipitation (Co-IP) experiment for detecting the SRSF1-truncated protein association between SART1, RBM15, and SRSF10 in 293T cells.
- https://cdn.elifesciences.org/articles/89316/elife-89316-fig9-data3-v1.pdf
Discussion
Failure of spermatogonia survival led to SCOS
Disturbed spermatogenesis can cause SCOS and ultimately male sterility (Jiao et al., 2021). In recent years, it has been reported that many spermatogonia-related gene deletions have disrupted SSC self-renewal and differentiation in patient and mouse models (La and Hobbs, 2019; Tan and Wilkinson, 2020; Wang et al., 2021). SCOS was observed in Ddx5, Tial1/Tiar, Uhrf1, Pramef12, Dot1l, and Rad51 deletion mouse models (Beck et al., 1998; Legrand et al., 2019; Lin et al., 2022; Qin et al., 2022; Wang et al., 2019; Zhou et al., 2022). Mouse models are still of great significance and reference for human SCOS studies, and they will provide a better understanding of how SCOS occurs and develops over time. Interestingly, our mouse model had SCOS (Figure 3D–I and Figure 4). The absence of germ cells represents classical SCOS in adult mouse testes (Figure 4; Wang et al., 2023). In addition, we found abnormal expression of spermatogonia-related genes (e.g. Gfra1, Pou5f1, Plzf, Dnd1, Stra8, and Taf4b) in cKO mouse testes (Figure 7F–H). These differentially expressed genes regulate SSC self-renewal and differentiation in mouse testes (Kanatsu-Shinohara and Shinohara, 2013; La and Hobbs, 2019; Tan and Wilkinson, 2020). Thus, this provided an opportunity for us to better study the underlying molecular mechanisms. These data indicate that SRSF1 deficiency impairs spermatogonia survival, leading to SCOS in male mice.
The formation of SSC pools and the establishment of niches are essential for spermatogenesis
The earliest event in the development of the SSC population is the migration of prospermatogonia from the centre of seminiferous cords where they have resided since sex determination of the embryonic gonad to the basement membrane (Oatley and Brinster, 2012). In mice, this process is also known as homing of precursor SSCs, which occurs in the initial 3 dpp and then develops into SSCs at 4–6 dpp for continuous self-renewal and differentiation (Lee and Shinohara, 2011; McLean et al., 2003; Oatley and Brinster, 2012; Tan and Wilkinson, 2020). Therefore, homing analysis of precursor SSCs was performed in 5 dpp cKO mouse testes. Interestingly, the VASA and SOX9 co-staining results demonstrated that partial germ cells could not complete homing in 5 dpp cKO testes (Figure 6A and B). Further, immunohistochemical staining for FOXO1 and statistical results indicated that germ cells in which FOXO1 is expressed in the nucleus similarly undergo abnormal homing (Figure 6C–E). Germ cells that do not migrate to the basement membrane are unable to form SSC pools and establish niches (McLean et al., 2003). These SSCs that lose their ecological niche will cease to exist. In our data, TUNEL results showed that apoptosis significantly increased in 7 dpp cKO mouse testes. At once, the quantifications of germ cells per tubule showed a significant reduction in 7 dpp and 14 dpp cKO testes, especially 14 dpp cKO testes (Figure 5B). In conclusion, SRSF1 is crucial for the formation of SSC pools and the establishment of niches through homing of precursor SSCs.
Abnormal AS impaired the survival of spermatogonia
AS is commonly found in mammals, especially in the brain and testes (Mazin et al., 2021; Merkin et al., 2012; Wang et al., 2008). AS plays essential roles in the posttranscriptional regulation of gene expression during many developmental processes, such as SSC self-renewal and differentiation (Chen et al., 2018; Song et al., 2020). Recently, BUD31-mediated AS of Cdk2 was shown to be required for SSC self-renewal and differentiation (Qin et al., 2023). Srsf10 depletion disturbed the AS of genes, including Nasp, Bclaf1, Rif1, Dazl, Kit, Ret, and Sycp1 (Liu et al., 2022). UHRF1 interacts with snRNAs and regulates AS of Tle3 in mouse SSCs (Zhou et al., 2022). Mettl3-mediated m6A regulates AS of Sohlh1 and Dazl (Xu et al., 2017). We found that SRSF1 acts as an alternative RNA splicing regulator and directly interacts with Tial1/Tiar transcripts to regulate splicing events in spermatogonia (Figure 8E–G). Additionally, expression levels of TIAL1/TIAR isoform X2 were significantly suppressed (Figure 8J). Interestingly, Tial1/Tiar transcript levels were not inhibited (Figure 8H and I). These results suggested that SRSF1 explicitly regulates the expression of Tial1/Tiar via AS. Studies have reported that TIAL1/TIAR is essential for primordial germ cell development in mouse testes (Beck et al., 1998). Tial1/Tiar deletion impairs germ cell survival leading to SCOS, consistent with our phenotype (Figures 3E–I–4A–C; Beck et al., 1998). Taken together, SRSF1 may affect germ cell survival by directly binding and regulating Tial1/Tiar expression through AS.
We found that SRSF1 could interact with AS-related proteins (e.g. SRSF10, SART1, RBM15, SRRM2, SF3B6, and SF3A2) (Figure 9F). A recent study reported that SRSF10 deficiency impaired spermatogonia differentiation but did not affect homing of precursor SSCs (Liu et al., 2022). However, our data showed that SRSF1 is essential for homing of mouse precursor SSCs. Therefore, this suggests that SRSF1 has a specific function in the homing of precursor SSCs independent of SRSF10.
SRSF1-mediated posttranscriptional regulation during homing of precursor SSCs provides new insights into the treatment of human reproductive diseases
Aberrant homing of precursor SSCs often lead to gametogenic failure, resulting in subfertility or infertility (Jiao et al., 2021; Kanatsu-Shinohara and Shinohara, 2013; La and Hobbs, 2019; Song and Wilkinson, 2014). Loss-of-function mutations in humans and corresponding knockout/mutated mice have been extensively researched (Jiao et al., 2021). However, AS-related posttranscriptional regulation during meiosis has not been well studied. In recent years, there have been reports that the RNA-binding proteins SRSF10, UHRF1, BUD31, and BCAS2 regulate AS in mouse SSCs (Liu et al., 2022; Liu et al., 2017; Qin et al., 2023; Zhou et al., 2022). This study used a multiomics approach to perform in-depth analyses of SRSF1-mediated posttranscriptional regulatory mechanisms to enrich the field. It also provides new ideas and insights for clinical diagnosis and treatment.
In summary, this study demonstrates that SRSF1 plays a critical role in posttranscriptional regulation to implement homing of precursor SSCs (Figure 9K). Thus, the posttranscriptional regulation of SRSF1-mediated splicing is resolved during the formation of SSC pools and the establishment of niches.
Materials and methods
Mouse strains
Request a detailed protocolC57BL/6N and ICR mice were purchased from Beijing Vital River Laboratory Animal Technology Co., Ltd. Srsf1Fl/Fl mice were generated in the laboratory of Prof. Xiangdong Fu (University of California, San Diego, CA, USA) and were kindly provided by Prof. Yuanchao Xue (Institute of Biophysics, Chinese Academy of Sciences, Beijing, China) (Xu et al., 2005). Vasa-Cre mice were obtained from The Jackson Laboratory (Gallardo et al., 2007). To generate Srsf1 cKO mice, Vasa-Cre mice were crossed with Srsf1Fl/Fl mice. The primers used for PCR to genotype Srsf1Fl/Fl and Vasa-Cre mice are shown in Supplementary file 5. All mice were bred and housed under specific pathogen-free conditions with a controlled temperature (22 ± 1°C) and exposed to a constant 12 hr light-dark cycle in the animal facilities of China Agricultural University. All experiments were conducted according to the guidelines and with the approval of the Institutional Animal Care and Use Committee of China Agricultural University (No. AW80401202-3-3).
Cell
Request a detailed protocolHEK293T cells were obtained from the American Type Culture Collection (ATCC) and cultured in Dulbecco’s modified Eagle’s medium (C11995500BT, Gibco) supplemented with 10% fetal bovine serum (SE200-ES, VISTECH), Penicillin-Streptomycin Solution (C0222, Beyotime). All cells were maintained in a humidified incubator containing 5% CO2 at 37°C. In addition, all of the cells used in the study tested negative in routine tests for Mycoplasma species using RT-PCR.
Fertility test
Request a detailed protocolFor 15 days, two 8-week-old ICR female mice were caged with one 8-week-old male control (Ctrl) or cKO mouse. The mice were kept individually after the appearance of the vaginal plug, and the dates were recorded. Male mice continue to be caged after 2 days. The number of pups from each female was recorded each day, and the date of parturition was recorded.
Immunostaining and histological analyses
Request a detailed protocolMouse testes were fixed with 4% paraformaldehyde (PFA, P6148-500G, Sigma-Aldrich) in PBS (pH 7.4) at 4°C overnight, dehydrated in graded ethanol solutions, vitrified with xylene, and embedded in paraffin. Testis sections were cut at a 5 μm thickness for immunostaining and histological analyses. For histological analyses, sections were dewaxed in xylene, rehydrated in a graded ethanol solution, and stained with haematoxylin-eosin. After sealing the slides with neutral resin, a Ventana DP200 system was used for imaging. For immunofluorescence analyses, antigen retrieval was performed by microwaving the sections with sodium citrate buffer (pH 6.0). After blocking with 10% normal goat serum at room temperature for 1 hr, the sections were incubated with primary antibodies (Supplementary file 6) in 5% normal goat serum overnight at 4°C. After washing with PBS, the sections were incubated with secondary antibodies (Supplementary file 6) at room temperature in the dark for 1 hr. The slides were mounted in an antifade mounting medium with DAPI (P0131, Beyotime). Photographs were taken with a Nikon A1 laser scanning confocal microscope and a Zeiss OPTOME fluorescence microscope. For immunohistochemistry analyses, antigen retrieval was performed by microwaving the sections with sodium citrate buffer (pH 6.0). Five dpp testis sections were prepared as described in the instructions for Immunohistochemistry Kit (PV-9001, ZSGB-BIO). After sealing the slides with neutral resin, a Ventana DP200 system was used for imaging.
Whole-mount immunostaining
Request a detailed protocolThe testes were collected and dispersed with 5 ml syringes. Blown-out tubules were fixed in PFA at 4°C for 4 hr. The tubules were washed three times with PBS (pH 7.4) for 5 min each and stored at 4°C. The tubules were permeated with 0.3% Triton X-100 for 1 hr at 4°C. Then, whole-mount staining followed the immunofluorescence protocol.
TUNEL apoptosis analyses
Request a detailed protocolSeven dpp testis sections were prepared as described in the instructions for the TUNEL Apoptosis Assay Kit (C1088, Beyotime). Photographs were taken with a Nikon A1 laser scanning confocal microscope and a Zeiss OPTOME fluorescence microscope. Apoptosis rate was derived from the number of TUNEL-positive signals in tubules as a percentage of the total number of germ cells.
RT-PCR and RT-qPCR
Request a detailed protocolTotal RNA was extracted by using RNAiso Plus (9109, Takara), and the concentration was measured with a Nano-300 ultramicro spectrophotometer (Allsheng). cDNA was obtained according to the instructions of a TIANScript II RT kit (KR107, TIANGEN). The expression of transcripts of the target gene was measured by using a LightCycle 96 instrument (Roche) with Hieff UNICON SYBR green master mix (11198ES08, Yeasen). AS analyses were performed on a RePure-A PCR instrument (BIO-GENER). Primers were synthesised by Sangon Biotech (Supplementary file 5). The expression level of Gapdh or Actb was used as the control, and this value was set as 1. Other samples’ relative transcript expression levels were obtained by comparing them with the control results.
RNA-seq
Request a detailed protocolTotal RNA was extracted from mouse testes according to the above protocol at 5 dpp. Briefly, mRNA was purified from total RNA using poly-T oligo-attached magnetic beads. After fragmentation, we established a transcriptome sequencing library and assessed library quality on an Agilent Bioanalyzer 2100 system. The clustering of the index-coded samples was performed on a cBot Cluster Generation System using a TruSeq PE Cluster kit v3-cBot-HS (Illumina) according to the manufacturer’s instructions. After cluster generation, the library preparations were sequenced on the Illumina NovaSeq platform, and 150 bp paired-end reads were generated. After quality control, all downstream analyses were performed on clean, high-quality data. The reference genome index was built, and paired-end clean reads were aligned to the reference genome using HISAT2 software (version 2.0.5). FeatureCounts (version 1.5.0) counted the reads mapped to each gene. Then, the FPKM value of each gene was calculated based on the length of the gene and the read count mapped to this gene. Differential expression analyses of cKO/Ctrl mouse testes (three biological replicates per condition) were performed using the DESeq2 R package (version 1.20.0). Genes with a padj ≤0.05 identified by DESeq2 were considered differentially expressed.
AS analyses
Request a detailed protocolrMATS software (version 3.2.5) was used to analyse the AS events in cKO mouse germ cells based on RNA-seq data. Five types of AS events (SE, RI, MXE, A5SS, and A3SS) were revealed by rMATS software. Our threshold for screening differentially significant AS events was an FDR of less than 0.05. Splicing events with an FDR less than 0.05 and an inclusion-level difference with a significance of at least 5% (0.05) were considered statistically significant. IGV (2.16.0) software was used to visualise and confirm AS events based on RNA-seq data.
GO enrichment analyses
Request a detailed protocolThe GO enrichment analyses of differentially expressed genes and AS genes were implemented with the clusterProfiler R package (version 3.4.4), in which gene length bias was corrected. All expressed genes (TPM sum of all samples ≥1) are listed background. GRCm38/mm10 was used as a mouse reference genome, and the Benjamini-Hochberg multiple methods was applied to adjust for multiple testing. GO enrichment analyses with corrected p values of less than 0.05 were significantly enriched for differentially expressed genes and AS genes.
Western blotting
Request a detailed protocolTotal protein was extracted with cell lysis buffer (P0013, Beyotime) containing PMSF (1:100, ST506, Beyotime) and a protease inhibitor cocktail (1:100, P1005, Beyotime). Protein concentration was determined with the BCA Protein Assay Kit (CW0014S, CWBiotech). The protein lysates were electrophoretically separated on sodium dodecyl sulfate-polyacrylamide gels and electrically transferred to polyvinylidene fluoride membranes (IPVH00010, Millipore). The membranes were blocked in 5% skimmed milk for 1 hr and incubated with the primary antibodies (Supplementary file 6) for one night at 4°C. Then, the membranes were incubated with secondary antibodies (Supplementary file 6) at room temperature for 1 hr. The proteins were visualised using a Tanon 5200 chemiluminescence imaging system following incubation with BeyoECL Plus (P0018S, Beyotime).
IP, IP-MS, Co-IP
Request a detailed protocolTotal protein was extracted with cell lysis buffer (P0013, Beyotime) containing PMSF (1:100, ST506, Beyotime) and a protease inhibitor cocktail (1:100, P1005, Beyotime). After incubation on ice for 20 min, the lysate was added to 20 μl of protein A agarose (P2051-2 ml, Beyotime) for pre-clearing at 4°C for 1 hr. Two micrograms of an SRSF1 antibody (sc-33652, Santa Cruz Biotechnology) and a normal mouse IgG (sc-3879, Santa Cruz Biotechnology) were added to the lysate and the mixture was incubated overnight at 4°C. The next day, 60 μl of protein A agarose was added to the lysate, which was then incubated at 4°C for 4 hr. The agarose complexes containing antibodies and target proteins were washed three times for 5 min at 4°C. IP and Co-IP were performed according to the above western blotting protocol. The complex was sent to the protein mass spectrometry laboratory for IP-MS analyses using a Thermo Q-Exactive high-resolution mass spectrometer (Thermo Scientific, Waltham, MA, USA). Raw data from the mass spectrometer were preprocessed with Mascot Distiller 2.4 for peak picking. The resulting peak lists were searched against the uniport mouse database using Mascot 2.5 search engine.
RIP and RIP-qPCR
Request a detailed protocolAs described previously (Gagliardi and Matarazzo, 2016), RIP was performed using 5 dpp mouse testes. The testes were lysed in cell lysis buffer (P0013, Beyotime) containing PMSF (1:100, ST506, Beyotime), a proteinase inhibitor cocktail (1:100, P1005, Beyotime), DTT (1:50, ST041-2 ml, Beyotime), and an RNase inhibitor (1:20, R0102-10 kU, Beyotime). After incubation on ice for 20 min, the lysate was added to 20 μl of protein A agarose (P2051-2 ml, Beyotime) for pre-clearing at 4°C for 1 hr. Two micrograms of an SRSF1 antibody (sc-33652, Santa Cruz Biotechnology) and a normal mouse IgG (sc-3879, Santa Cruz Biotechnology) were added to the lysate, which was then incubated overnight at 4°C. The next day, 60 μl of protein A agarose was added to the lysate, and the mixture was incubated at 4°C for 4 hr. The agarose complexes containing antibodies, target proteins, and RNA were washed three times for 5 min at 4°C and repeated. Protein-bound RNA was extracted using RNAiso Plus and a Direct-zol RNA MicroPrep Kit. RIP-qPCR was performed according to the above RT-qPCR protocol.
Statistical analyses
Request a detailed protocolPearson’s correlation coefficients (R) were calculated by using the scores of the two samples for MS. GraphPad Prism software (version 9.0.0) was used for the statistical analyses, and the values and error bars represent the mean ± SEM. Significant differences between the two groups were analysed using Student’s t-test. Statistical significance is indicated as follows: exact p value p ≥ 0.05; *p < 0.05; **p < 0.01; ***p < 0.001; ****p < 0.0001.
Data availability
All data generated or analysed during this study are included in this published article, its supplementary information files and publicly available repositories. Mass spec data are included in Supplementary file 7 (Mass spec data). The RNA-seq data were deposited in GEO (https://www.ncbi.nlm.nih.gov/geo/) under accession number GSE227575.
-
NCBI Gene Expression OmnibusID GSE227575. Splicing factor SRSF1 is essential for homing of precursor spermatogonial stem cells in mice.
-
NCBI Gene Expression OmnibusID GSE227303. SRSF1 is crucial for male meiosis through alternative splicing during homologous pairing and synapsis in mice [CLIP-seq].
References
-
Splicing factor SRSF1 promotes breast cancer progression via oncogenic splice switching of PTPMT1Journal of Experimental & Clinical Cancer Research 40:171.https://doi.org/10.1186/s13046-021-01978-8
-
RIP: RNA ImmunoprecipitationMethods in Molecular Biology 1480:73–86.https://doi.org/10.1007/978-1-4939-6380-5_7
-
Foxo1 is required in mouse spermatogonial stem cells for their maintenance and the initiation of spermatogenesisThe Journal of Clinical Investigation 121:3456–3466.https://doi.org/10.1172/JCI57984
-
Possible fetal determinants of male infertilityNature Reviews Endocrinology 10:553–562.https://doi.org/10.1038/nrendo.2014.97
-
Spermatogonial stem cell self-renewal and developmentAnnual Review of Cell and Developmental Biology 29:163–187.https://doi.org/10.1146/annurev-cellbio-101512-122353
-
Splicing factor SRSF1 controls T cell hyperactivity and systemic autoimmunityThe Journal of Clinical Investigation 129:5411–5423.https://doi.org/10.1172/JCI127949
-
A comparison between the morphology and cell kinetics of gonocytes and adult type undifferentiated spermatogonia in the mouseInternational Journal of Andrology 4:475–493.https://doi.org/10.1111/j.1365-2605.1981.tb00732.x
-
Mechanisms regulating mammalian spermatogenesis and fertility recovery following germ cell depletionCellular and Molecular Life Sciences 76:4071–4102.https://doi.org/10.1007/s00018-019-03201-6
-
SRSF1 plays a critical role in invariant natural killer T cell development and functionCellular & Molecular Immunology 18:2502–2515.https://doi.org/10.1038/s41423-021-00766-w
-
SRSF1 inhibits autophagy through regulating Bcl-x splicing and interacting with PIK3C3 in lung cancerSignal Transduction and Targeted Therapy 6:108.https://doi.org/10.1038/s41392-021-00495-6
-
Alternative splicing during mammalian organ developmentNature Genetics 53:925–934.https://doi.org/10.1038/s41588-021-00851-w
-
Characterization of spermatogonial stem cell maturation and differentiation in neonatal miceBiology of Reproduction 69:2085–2091.https://doi.org/10.1095/biolreprod.103.017020
-
The germline stem cell niche unit in mammalian testesPhysiological Reviews 92:577–595.https://doi.org/10.1152/physrev.00025.2011
-
Bud31-mediated alternative splicing is required for spermatogonial stem cell self-renewal and differentiationCell Death and Differentiation 30:184–194.https://doi.org/10.1038/s41418-022-01057-1
-
Transcriptional control of spermatogonial maintenance and differentiationSeminars in Cell & Developmental Biology 30:14–26.https://doi.org/10.1016/j.semcdb.2014.02.005
-
The function of Pre-mRNA alternative splicing in mammal spermatogenesisInternational Journal of Biological Sciences 16:38–48.https://doi.org/10.7150/ijbs.34422
-
A single-cell view of spermatogonial stem cellsCurrent Opinion in Cell Biology 67:71–78.https://doi.org/10.1016/j.ceb.2020.07.005
-
Alternative splicing in the testesCurrent Opinion in Genetics & Development 12:615–619.https://doi.org/10.1016/s0959-437x(02)00347-7
-
Sertoli cell-only syndrome: advances, challenges, and perspectives in genetics and mechanismsCellular and Molecular Life Sciences 80:67.https://doi.org/10.1007/s00018-023-04723-w
-
HDOCK: a web server for protein-protein and protein-DNA/RNA docking based on a hybrid strategyNucleic Acids Research 45:W365–W373.https://doi.org/10.1093/nar/gkx407
Article and author information
Author details
Funding
National Natural Science Foundation of China (32171111)
- Jiali Liu
Natural Science Foundation of Beijing Municipality (5222015)
- Jiali Liu
The funders had no role in study design, data collection and interpretation, or the decision to submit the work for publication.
Acknowledgements
This work was supported by the National Natural Science Foundation of China (32171111); and the Beijing Natural Science Foundation (5222015). We thank Prof. Yuanchao Xue (Institute of Biophysics, Chinese Academy of Sciences, Beijing, China) for sharing Srsf1Fl/Fl mice, Prof. Shuyang Yu, Hua Zhang, and Chao Wang (China Agricultural University, Beijing, China) for thoughtful discussions and suggestions, and all the members of Prof. Hua Zhang, Chao Wang, and Shuyang Yu laboratory for helpful discussions and comments. We thank Novogene for their assistance with the RNA-seq and CLIP-seq experiments.
Ethics
All experiments were conducted according to the guidelines and with the approval of the Institutional Animal Care and Use Committee of China Agricultural University (No. AW80401202-3-3).
Version history
- Sent for peer review:
- Preprint posted:
- Reviewed Preprint version 1:
- Reviewed Preprint version 2:
- Reviewed Preprint version 3:
- Version of Record published:
Cite all versions
You can cite all versions using the DOI https://doi.org/10.7554/eLife.89316. This DOI represents all versions, and will always resolve to the latest one.
Copyright
© 2023, Sun et al.
This article is distributed under the terms of the Creative Commons Attribution License, which permits unrestricted use and redistribution provided that the original author and source are credited.
Metrics
-
- 1,170
- views
-
- 180
- downloads
-
- 5
- citations
Views, downloads and citations are aggregated across all versions of this paper published by eLife.
Citations by DOI
-
- 5
- citations for umbrella DOI https://doi.org/10.7554/eLife.89316