Suggestion of creatine as a new neurotransmitter by approaches ranging from chemical analysis and biochemistry to electrophysiology
eLife assessment
This study presents valuable observations on a potential role of creatine (Cr) as a novel neurotransmitter. The data provide solid evidence that Cr is present in synaptic vesicles. If, in the future, a receptor can be described, it will support the claim that Cr is synaptically released and binds to a post-synaptic receptor. This would be of wide interest to the field of neuroscience.
https://doi.org/10.7554/eLife.89317.4.sa0Valuable: Findings that have theoretical or practical implications for a subfield
- Landmark
- Fundamental
- Important
- Valuable
- Useful
Solid: Methods, data and analyses broadly support the claims with only minor weaknesses
- Exceptional
- Compelling
- Convincing
- Solid
- Incomplete
- Inadequate
During the peer-review process the editor and reviewers write an eLife Assessment that summarises the significance of the findings reported in the article (on a scale ranging from landmark to useful) and the strength of the evidence (on a scale ranging from exceptional to inadequate). Learn more about eLife Assessments
Abstract
The discovery of a new neurotransmitter, especially one in the central nervous system, is both important and difficult. We have been searching for new neurotransmitters for 12 y. We detected creatine (Cr) in synaptic vesicles (SVs) at a level lower than glutamate and gamma-aminobutyric acid but higher than acetylcholine and 5-hydroxytryptamine. SV Cr was reduced in mice lacking either arginine:glycine amidinotransferase (a Cr synthetase) or SLC6A8, a Cr transporter with mutations among the most common causes of intellectual disability in men. Calcium-dependent release of Cr was detected after stimulation in brain slices. Cr release was reduced in Slc6a8 and Agat mutants. Cr inhibited neocortical pyramidal neurons. SLC6A8 was necessary for Cr uptake into synaptosomes. Cr was found by us to be taken up into SVs in an ATP-dependent manner. Our biochemical, chemical, genetic, and electrophysiological results are consistent with the possibility of Cr as a neurotransmitter, though not yet reaching the level of proof for the now classic transmitters. Our novel approach to discover neurotransmitters is to begin with analysis of contents in SVs before defining their function and physiology.
Introduction
Neural signaling depends on chemical transmission between neurons and their target cells (Du Bois-Reymond, 1877; Langley, 1901b; Elliot, 1904; Elliott, 1905; Dale, 1914; Loewi, 1921). Neurotransmission depends on chemicals such as neurotransmitters, neuromodulators, and neuropeptides. Decades of work, sometimes with convoluted paths, were involved before a molecule was established as a classic neurotransmitter (Rao, 2019). Initial hints about cholinergic signaling were obtained in the 1800s (Bernard, 1857; Langley and Dickinson, 1890; Langley, 1905; Langley, 1906). Choline (Strecker, 1862; Liebreich, 1865) and acetylcholine (ACh) (Baeyer, 1867) were discovered decades before their pharmacological effects were found around the turn of 20th century (Hunt and Taveau, 1906; Mott and Halliburton, 1899; Hunt, 1900). Henry Dale and colleagues realized similarities of ACh and parasympathetic stimulation (Dale, 1914; Ewins, 1914), but it was not until 1929 when ACh was detected in the body (Dale and Dudley, 1929) and 1934 when ACh was proven a neurotransmitter in the peripheral nervous system (PNS) (Feldberg and Gaddum, 1934; Brown and Feldberg, 1936; Dale et al., 1936). It took nearly 100 y from the finding of the effects of supradrenal gland damage (Addison, 1855) or removal (Schäfer, 1908), the observation of an activity in the supradrenal gland (Oliver and Schäfer, 1895), the isolation of an inactive derivative (Abel, 1898; Abel and Crawford, 1897; Abel, 1899; Abel, 1901), and the successful isolation of adrenaline (Takamine, 1901; Takamine, 1902; Aldrich, 1901), the notice of similarities between adrenaline and sympathetic stimulation (Elliot, 1904; Elliott, 1905; Langley, 1901a; Barger and Dale, 1910; Cannon et al., 1933), to the mid-1940s when Ulf von Euler proved that noradrenaline (NA) was the neurotransmitter of the sympathetic nerves (von Euler, 1946; von Euler, 1948; von Euler, 1956). While it is not easy to establish a molecule as a neurotransmitter in the PNS, it is even harder to establish a central nervous system (CNS) neurotransmitter. Three decades elapsed between the time when ACh was proven to be a PNS neurotransmitter and the time when it was established as a CNS neurotransmitter (Mitchell, 1963; Collier and Mitchell, 1966; Collier and Mitchell, 1967) and two decades between NA as a peripheral transmitter and a central transmitter (Carlsson et al., 1962; Björklund et al., 1968).
If a neurotransmitter acts only in the CNS, but not in the PNS, it is much more difficult to discover or to prove. Most neurotransmitters were discovered for their effects on peripheral tissues, with muscle contraction or relaxation as a major readout. Glutamate (Glu) (Robbins, 1958; Curtis et al., 1959; Curtis and Watkins, 1960; Curtis and Watkins, 1963) and gamma-aminobutyric acid (GABA) (Curtis and Watkins, 1960; Roberts and Frankel, 1950; Udenfriend, 1950; Florey, 1954; Florey and McLennan, 1955) were discovered partly because of their peripheral effects and partly because of their effects on spinal neurons. There is no reason for central neurotransmitters to also act peripherally, but relatively little efforts have been reported to find small-molecule neurotransmitters acting only on CNS neurons with no peripheral bioassays available. Premature assumptions and technical difficulties are among the major reasons why the hunt for neurotransmitters has not been a highly active area of research over the last three decades.
Are there more neurotransmitters and how can they be discovered? Classic neurotransmitters are stored in synaptic vesicles (SVs) (Fatt and Katz, 1950; Fatt and Katz, 1952; Del Castillo and Katz, 1954; Robertson, 1953; Palade and Palay, 1954; Palay, 1954; De Robertis and Bennett, 1954; De Robertis and Bennett, 1955; del Castillo and Katz, 1956). They are released upon electric stimulation before being degraded enzymatically or taken up into the presynaptic terminal by cytoplasmic transporters and into SVs by vesicular transporters (Axelrod et al., 1959; Radian and Kanner, 1985; Radian et al., 1986; Guastella et al., 1990; Pacholczyk et al., 1991; Blakely et al., 1991). Most of the major textbooks list either three (Purves et al., 2001; Purves et al., 2016; Bear et al., 2016) or four (Kandel et al., 2013; Kandel et al., 2021; Siegel and Sapru, 2011) criteria of a neurotransmitter: presence in presynaptic neurons, release upon stimulation, action on postsynaptic neurons, mechanism of removal. Some molecules commonly accepted as neurotransmitters still do not meet all the criteria listed in different textbooks, but they nonetheless play important functional roles in the CNS and their defects cause human diseases. Over time, different small molecules have been proposed to function as neurotransmitters (e.g., Björklund et al., 1968; Felix and Künzle, 1974), but none satisfies all the criteria. Robust and reliable detection of the candidate molecule in SVs is often, though not always, the problem (cf. Chantranupong et al., 2020).
Beginning in 2011, we have been actively searching for new neurotransmitters in the mammalian brain. We have tried different approaches, including searching for neuroactive substances in the cerebral spinal fluid (CSF) and following transporters potentially localized in the SVs. One approach that we have now taken to fruition is the purification of the SVs from mouse brains coupled with chemical analysis of their contents. We have found known transmitters such as Glu, GABA, ACh, and 5-hydroxytryptamine (5-HT). But more importantly, we have reproducibly detected creatine (Cr) in SVs.
Cr was discovered in 1832 by Michel-Eugène Chevreul (Chevreul, 1835; Liebig, 1847) and has long been considered as an energy buffer in the muscle and the brain (Wyss and Kaddurah-Daouk, 2000; Brosnan and Brosnan, 2007; Wallimann et al., 2011). Half of Cr in a mammalian animal is thought to come from diet and the rest from endogenous synthesis (Braissant et al., 2011). Most of the Cr is present in the muscle, but it is also present in the brain. Although most of the endogenous Cr is synthesized in the kidney, the pancreas, and the liver (Wyss and Kaddurah-Daouk, 2000; Ohtsuki et al., 2002), Cr is also synthesized in the brain (Braissant et al., 2011; Braissant et al., 2001; Braissant et al., 2007).
Solute carriers (SLC) contribute to both cytoplasmic and vesicular transporters. With 19 members in humans, family 6 (SLC6) are secondary active transporters relying on electrochemical Na+ or H+ gradients (Nelson, 1998; Chen et al., 2004; Höglund et al., 2005; Bröer and Gether, 2012; Iversen, 2006; Bröer, 2006). SLC6 is also known as the neurotransmitter transporter (NTT) family because some members transport neurotransmitters such as GABA (by SLC6A1 or GABA transporter 1, GAT1; SLC6A13 or GAT2; SLC6A11 or GAT3) (Guastella et al., 1992; Clark et al., 1992; Borden et al., 1992; Lopez-Corcuera et al., 1992), NA (by SLC6A2 or NA transporter [NET]) (Pacholczyk et al., 1991), dopamine (by SLC6A3 or DAT) (Giros et al., 1991; Kilty et al., 1991; Shimada et al., 1991), 5-HT (by SLC6A4 or serotonin transporter [SERT]) (Blakely et al., 1991; Hoffman et al., 1991), and glycine (by SLC6A9, or GlyT1; SLC6A5 or GlyT2) (Guastella et al., 1992; Smith et al., 1992; Liu et al., 1992). Cr is transported by SLC6A8 (also known as CrT, CT1, or CRCT) (Mayser et al., 1992; Guimbal and Kilimann, 1993; Gonzalez and Uhl, 1994; Nash et al., 1994; Schloss et al., 1994; Sora et al., 1994; Barnwell et al., 1995). In addition to peripheral organs and tissues, SLC6A8 is also expressed in the nervous system where it is mainly in neurons (Braissant et al., 2001; Schloss et al., 1994; Happe and Murrin, 1995; Saltarelli et al., 1996; Mak et al., 2009). SLC6A8 protein could be found on the plasma membrane of neurons (Mak et al., 2009; Speer et al., 2004; Lowe et al., 2015).
The functional significance of SLC6A8 in the brain is supported by symptoms of humans defective in SLC6A8. Mutations in SLC6A8 were found in human patients with intellectual disability (ID), delayed language development, epileptic seizures, and autistic-like behaviors (Salomons et al., 2001; Margherita Mancardi et al., 2007). They are collectedly known as Cr transporter deficiency (CTD), with ID as the hallmark. Particular vulnerability of language development has been observed in some SLC6A8 mutations which had mild ID but severe language delay (Battini et al., 2011). CTD contributes to approximately 1–2.1% of X-linked mental retardation (Rosenberg et al., 2004; Newmeyer et al., 2005; Clark et al., 2006; Lion-François et al., 2006; Arias et al., 2007; Puusepp et al., 2010; Cheillan et al., 2012; van de Kamp et al., 2014; Joncquel-Chevalier Curt et al., 2015). While CTD is highly prevalent in ID males, it is also present in females, with an estimated carrier frequency of 0.024% (DesRoches et al., 2015).
Slc6a8 knockout mice (Skelton et al., 2011; Kurosawa et al., 2012; Baroncelli et al., 2016) showed typical symptoms of human CTD patients with early and progressive impairment in learning and memory. Mice with brain- and neuronal-specific knockout of Slc6a8 showed deficits in learning and memory without changes in locomotion caused by peripheral involvement of Slc6a8 (Udobi et al., 2018; Molinaro et al., 2019). Deletion of Slc6a8 from dopaminergic neurons in the brain caused hyperactivity (Abdulla et al., 2020). These results demonstrate that SLC6A8 is functionally important in neurons.
Cr deficiency syndromes (CDS) are inborn errors of Cr metabolism, which can result from defects in one of the three genes: guanidinoacetate methyltransferase (GAMT) (Stöckler et al., 1994), arginine-glycine amidinotransferase (AGAT) (Bianchi et al., 2000; Item et al., 2001), and SLC6A8 (Salomons et al., 2001). That they all show brain disorders indicates the functional importance of Cr in the brain (Stockler-Ipsiroglu et al., 2014; Stockler-Ipsiroglu et al., 2015; Khan et al., 2016; Fons and Campistol, 2016).
Here we first biochemically purified SVs from the mouse brain and discovered the presence of Cr, as well as classic neurotransmitters Glu and GABA, ACh and 5-HT, in SVs. We then detected calcium (Ca2+)-dependent releases of Cr, Glu, and GABA but not ACh and 5-HT when neurons were depolarized by increased extracellular concentrations of potassium (K+). Both the level of Cr in SVs and that of Cr released upon stimulation were decreased significantly when either the gene for Slc6a8 or the gene for Agat were eliminated genetically. When Cr was applied to slices from the neocortex, the activities of pyramidal neurons were inhibited. Furthermore, we confirmed that Cr was taken up by synaptosomes and found that Cr uptake was significantly reduced when the Slc6a8 gene was deleted. Finally, we found that Cr was transported into SVs. Thus, multidisciplinary studies with biochemistry, genetics, and electrophysiology have suggested that Cr is a new neurotransmitter, though the discovery of a receptor for Cr would prove it.
Results
Detection of Cr in SVs from the mouse brain
To search for new neurotransmitters, we tried several approaches. For example, we used Ca2+ imaging to detect neuroactive substances in the cerebrospinal fluid (CSF), but it was difficult to rule out existing neurotransmitters and select responses from potentially new neurotransmitters. We also transfected cDNAs for all human SLCs into dissociated cultures of primary neurons from the mouse brain and found that more than 50 out of all SLCs could be localized in SVs. However, when we used CRISPR-Cas9 to tag some of the candidate SLCs in mice, some of them were found to be expressed outside the CNS, indicating that, while ectopic expression of these candidate SLCs could be localized on SVs, the endogenous counterparts were not localized on SVs.
Here, we report our approach using the purification of SVs as the first step (Figure 1—figure supplement 1A; Whittaker et al., 1964; Nagy et al., 1976; Matthew et al., 1981; De Camilli et al., 1983; Huttner et al., 1983; Burger et al., 1989; Burger et al., 1991; Maycox et al., 1992). Synaptophysin (Syp) is a specific marker for SVs (Jahn et al., 1985; Wiedenmann and Franke, 1985; Leube et al., 1987; Südhof et al., 1987) and an anti-Syp antibody was used to immunoisolate SVs (Burger et al., 1989; Burger et al., 1991; Jahn and Sudhof, 1994; Martineau et al., 2013; Bradberry et al., 2022). Visualization by electronic microscopy (EM) (Figure 1—figure supplement 1B, left panel) showed that the purified vesicles were homogeneous, with an average diameter of 40.44 ± 0.26 nm (n = 596 particles) (Figure 1—figure supplement 1B, right panel), consistent with previous descriptions of SVs (Ahmed et al., 2013; Takamori et al., 2006).
Immunoblot analysis with 20 markers of subcellular organelles of neurons and 1 marker for glia (Figure 1—figure supplement 1C) indicates that our purifications were highly effective, with SV markers detected after purification with the anti-Syp antibody, but not that with the control immunoglobulin G (IgG). SV proteins included Syp (Jahn et al., 1985; Wiedenmann and Franke, 1985; Leube et al., 1987; Südhof et al., 1987), synaptotagmin (Syt1) (Geppert et al., 1994), synatobrevin2 (Syb2) (Link et al., 1992; Schiavo et al., 1992), SV2A (Bajjalieh et al., 1992), H+-ATPase (Cidon and Sihra, 1989), and vesicular neurotransmitter transporters for glutamate (VGLUT1, VGLUT2) (Takamori et al., 2000) and GABA (VGAT) (McIntire et al., 1997). Immunoisolation by the anti-Syp antibody did not bring down markers for the synaptic membrane (with SNAP23 as a marker) (Kunii et al., 2021; Ravichandran et al., 1996; Suh et al., 2010), postsynaptic components (with PSD95 and GluN1 as markers) (Woods and Bryant, 1991; Cho et al., 1992), the Golgi apparatus (with GM130 and Golgin 97 as markers) (Nakamura et al., 1995; Griffith et al., 1997), early endosome (with early endosome-associated 1 [EEA1] as a marker) (Mu et al., 1995), the lysosome (with LC3B and cathepsinB as markers), the cytoplasma (with glyceraldehyde-3-phophate dehydrogenase [GAPDH] as a marker), mitochondria (with voltage-dependent anion channel [VDAC] as a marker), cytoplasmic membrane (with calcium voltage-gated channel subunit alpha 1 [CACNA1A] as a marker), axonal membrane (with glucose transporter type 4 [GluT4] as a marker), and glia membrane (with myelin basic protein [MBP] as a marker). These results indicated that the SVs we obtained were of high integrity and purity.
To detect and quantify small molecules as candidate transmitters present in the purified SVs, capillary electrophoresis-mass spectrometry (CE-MS) was optimized and utilized (Figure 1A, Figure 1—figure supplement 1A; Martineau et al., 2013; Tie et al., 2012). We found that the levels of classical neurotransmitters such as Glu, GABA, ACh, and 5-HT were significantly higher in SVs pulled down by the anti-Syp antibody than those in lysates pulled down by the control IgG (Figure 1A–E). Consistent with previous reports (Burger et al., 1991; Martineau et al., 2013), significant enrichment of neurotransmitters was observed only from SVs immunoisolated at near 0°C, but not at the room temperature (RT) (Figure 1A–E). By contrast, another small molecule, alanine (Figure 1G), was not elevated in SVs compared to the control.
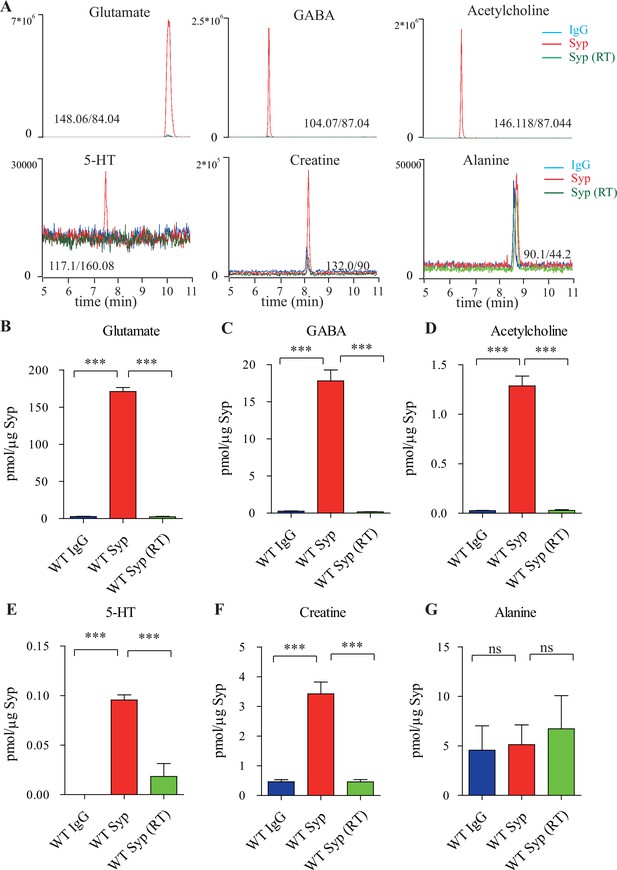
Presence of creatine (Cr) in synaptic vesicles (SVs) from the mouse brain.
(A) Representative raw traces from capillary electrophoresis-mass spectrometry (CE-MS) of indicated molecules from samples immunoisolated by the control immunoglobulin G (IgG) (blue) at 0–2°C, the monoclonal anti-synaptophysin (anti-Syp) antibody at 0–2°C (red), and the anti-Syp antibody at room temperature (RT, green). Q1/Q3 for identifying targets are indicated. (B–G) Quantification of the amounts of indicated molecules. The amount of a molecule was divided by the amount of the anti-Syp antibody bound to magnetic beads. Note glutamate (Glu) (B), gamma-aminobutyric acid (GABA)(C), acetylcholine (ACh) (D), 5-hydroxytryptamine (5-HT) (E), Cr (F), but not alanine (G) was higher in SVs pulled down by the anti-Syp antibody at 0–2°C than those pulled down by the IgG control or those pulled down at the RT. n = 10 (B–E, G) or 14 (F) samples per group, *** p<0.001, ns, not significant. One-way ANOVA with Tukey’s correction.
-
Figure 1—source data 1
Data for Figure 1B-G.
- https://cdn.elifesciences.org/articles/89317/elife-89317-fig1-data1-v1.xlsx
The amount of Glu was 171.1 ± 5.4 pmol/μg anti-Syp antibody (n = 10, Figure 1B), approximately 10 times that of GABA (n = 10,17.81 ± 1.47 pmol/μg anti-Syp antibody, Figure 1C). The amount of ACh was 1.29 ± 0.10 pmol/μg anti-Syp antibody (n = 10, Figure 1D), approximately 0.072 that of GABA. The amount of 5-HT was 0.096 ± 0.017 pmol/μg anti-Syp antibody (n = 10, Figure 1E). Thus, our purification and detection methods were highly reliable and sensitive enough to detect established neurotransmitters.
Under the same conditions, we also detected Cr in SVs (n = 14, Figure 1A, F). Amount of Cr in the SVs was found to be 3.43 ± 0.40 pmol/μg anti-Syp antibody (Figure 1F), which was approximately 2% of Glu, 19% of GABA, 266% of ACh, and 3573% of 5-HT. It is unlikely that these could be attributable to different levels for different neurotransmitters in each SV, but more likely attributable to the relative abundance of SVs containing different neurotransmitters. Also, 85–90% neurons in the mouse brain were glutamatergic while 10–15% were GABAergic (Meyer et al., 2011; Olbrich and Braak, 1985; Tremblay et al., 2016), which can explain our detection of Glu as approximately 10 times that of GABA (Figure 1B, C). Similarly, cholinergic neurons (5.67 × 105) (Li et al., 2018) represented 0.81% of total number of neurons (approximately 70 million) in the mouse brain (Herculano-Houzel et al., 2006), serotonergic neurons (approximately 26,000) for 0.037% of total neurons (Herculano-Houzel et al., 2006; Ishimura et al., 1988). Assuming that the content of each neurotransmitter in a single SV is similar, extrapolation from the above data would suggest that approximately 1.3–2.15% of neurons in the mouse brain are creatinergic.
To distinguish whether small molecules co-purified with SVs were in the SVs (Burger et al., 1989; Burger et al., 1991), or that they were just associated with the outside of SVs (Takamori et al., 2006), we tested the dependence of the presence of these molecules in the SVs on temperature and on the electrochemical gradient of H+. Cr was significantly reduced in SVs purified at RT compared to that immunoisolated at near 0°C (Figure 1F), supporting the presence of Cr inside, instead of outside, SVs.
Classical neurotransmitters are stored in SVs with an acidic environment inside (pH of 5.6–6.4) (Egashira et al., 2016; Mani and Ryan, 2009; Egashira et al., 2015). To further verify the storage of Cr in SVs and examine the role of H+ electrochemical gradient, we applied pharmacological inhibitors during purification (Chantranupong et al., 2020; Qian et al., 2021). The proton ionophore FCCP (carbonyl cyanide-4-(tri-fluoromethoxy) phenylhydrazone) was used to dissipate H+ electrochemical gradient (Qian et al., 2021; Schenck et al., 2009). FCCP significantly reduced the amount of Cr as well as classical neurotransmitters in SVs (Figure 1—figure supplement 2A–E). The extent of FCCP-induced reduction was correlated with the value of pKa or PI (isoelectric point) for different molecule: 5-HT (with pKa predicted to 10 and 9.31, Figure 1—figure supplement 2E) > Cr (PI of ~7.94, Figure 1—figure supplement 2A) > GABA (PI of 7.33, Figure 1—figure supplement 2C) > Glu (PI of 3.22, Figure 1—figure supplement 2B). Nigericin, a K+/H+ exchanger which dissipates ΔpH (Qian et al., 2021; Schenck et al., 2009), also reduced the amount of Cr and classical neurotransmitters in SVs (Figure 1—figure supplement 2A–E). Furthermore, in the presence of FCCP or nigericin, SV Cr was reduced to a level comparable to that pulled down by control IgG (Figure 1—figure supplement 2A), demonstrating the storage of Cr in SVs was dependent on H+ gradient. As a control, the non-neurotransmitter molecule alanine in SVs was not changed by the inhibitors (Figure 1—figure supplement 2F).
Reduction of SV Cr in mouse mutants lacking Slc6a8
SLC6A8, located on the X chromosome, encodes a transporter for Cr and its loss-of-function (LOF) mutations caused behavioral deficits in humans (Salomons et al., 2001; Margherita Mancardi et al., 2007) and mice (Skelton et al., 2011; Kurosawa et al., 2012; Baroncelli et al., 2016; Udobi et al., 2018; Molinaro et al., 2019; Abdulla et al., 2020). To investigate whether SLC6A8 affects Cr in SVs, we generated Slc6a8 knockout (KO) mice. Exon 1 of the Slc6a8 gene was partially replaced with CreERT2-WPRE-polyA by CRISPR/Cas9 (Figure 2A). Examination by reverse polymerase chain reaction (RT-PCR) (Figure 2—figure supplement 1A, B) and quantitative real-time reverse PCR (qPCR, Figure 2—figure supplement 1C, D) showed that Slc6a8 mRNA was not detected in either male or female mutants, and significantly reduced in female heterozygous (Slc6a8+/-). Consistent with previous reports, the body weights of Slc6a8 KO mice were reduced (Figure 2—figure supplement 2B, D; Skelton et al., 2011; Duran-Trio et al., 2021; Stockebrand et al., 2018). Brain weight was not significantly different between Slc6a8 KO mice and WT mice (Figure 2—figure supplement 2A, C).
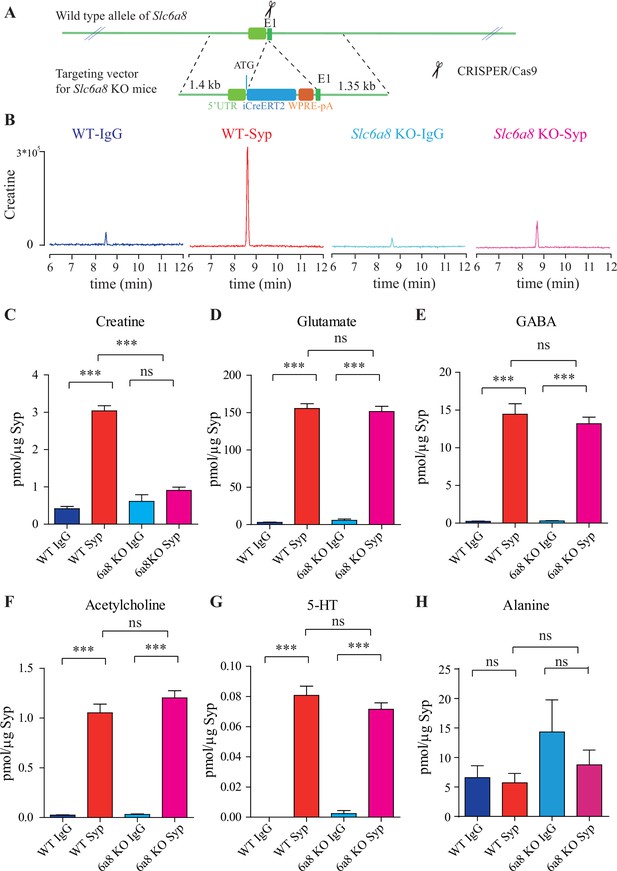
Slc6a8 and creatine (Cr) in synaptic vesicles (SVs).
(A) A schematic illustration of the strategy for generating Slc6a8 knockouts using CRISPR/Cas9. An iCreERT2-WPRE-pA cassette (~3.5 kb) was inserted immediately downstream of ATG in the Slc6a8 gene, substituting bp 4 to bp 51 in exon 1 (E1). (B) Representative raw traces of Cr immunoisolated by control immunoglobulin G (IgG) from WT mice (blue), the anti-synaptophysin (anti-Syp) antibody from WT mice (red), IgG from Slc6a8 KO mice (blue), and the anti-Syp antibody from Slc6a8 KO mice (red). (C–H) Quantification of indicated molecules. Note the selective reduction of Cr in SVs from Slc6a8 KO mice. n = 14 samples per group. ***p<0.001, ns, not significant. One-way ANOVA with Tukey’s correction.
-
Figure 2—source data 1
Data for Figure 2C–H.
- https://cdn.elifesciences.org/articles/89317/elife-89317-fig2-data1-v1.xlsx
When we examined the contents of SVs isolated by the anti-Syp antibody vs the control IgG, significant reduction was only observed for Cr, but not classical neurotransmitters (Figure 2B–H, Figure 2—figure supplement 3A–E). While Cr pulled down by IgG was not significantly different between Slc6a8-/Y and Slc6a8+/Y mice, SV Cr purified by the anti-Syp antibody from Slc6a8-/Y was reduced to approximately 1/3 that of the WT (Slc6a8+/Y) littermates (n = 14, Figure 2B and C). Compared to the IgG control, Cr in SVs was enriched in WT mice, but not in Slc6a8-/Y mice (Figure 2B and C). In both Slc6a8-/Y and Slc6a8+/Y mice, classical neurotransmitters in SVs were all enriched as compared to IgG controls (Figure 2D–G, Figure 2—figure supplement 3A–D). The amounts of Glu (Figure 2D, Figure 2—figure supplement 3A), GABA (Figure 2E, Figure 2—figure supplement 3B), ACh (Figure 2F, Figure 2—figure supplement 3C), and 5-HT (Figure 2G, Figure 2—figure supplement 3D) in SVs were not different between Slc6a8-/Y and Slc6a8+/Y mice. Molecules not enriched in SVs from WT mice, such as alanine, were also unaffected by Slc6a8 KO (Figure 2H, Figure 2—figure supplement 3E).
It is unlikely that the specific reduction of Cr in SVs from Slc6a8 KO mice was due to technical artifacts. First, the possibility of less SVs obtained from Slc6a8 KO mice was precluded by immunoblot analysis, as assessed by SV markers Syp, Syt, and H+-ATPase (Figure 2—figure supplement 4A). Second, data collected by high-resolution MS (Q Exactive HF-X, Thermo Scientific, Waltham, MA) also revealed selective decrease of SV Cr (m/z = 132.0576) from Slc6a8 KO mice (n = 8, Figure 2—figure supplement 4B–G), as quantified by the peak area. Peak areas for Glu (n = 8, Figure 2—figure supplement 4B, m/z = 148.0604), GABA (n = 8, Figure 2—figure supplement 4C, m/z = 104.0712), ACh (n = 8, Figure 2—figure supplement 4D, m/z = 146.1178), and alanine (n = 8, Ala, Figure 2—figure supplement 4E, m/z = 90.055) were not significantly different between SVs immmunoisolated with the anti-Syp antibody and control IgG from WT and Slc6a8 KO mice. However, peak areas (Figure 2—figure supplement 4G) and amplitude of Cr (n = 8, Figure 2—figure supplement 4F) signal were significantly increased in SVs from WT mice (anti-Syp antibody vs IgG), but not that from Slc6a8 KO mice.
Reduction of SV Cr in mouse mutants lacking Agat
AGAT is the enzyme catalyzing the first step in Cr synthesis (Braissant et al., 2001; Guthmiller et al., 1994) and its absence also led to Cr deficiency in the human brain and mental retardation (Bianchi et al., 2000; Item et al., 2001).
To investigate the requirement of AGAT for SV Cr, we utilized Agat ‘knockout-first’ mice (Figure 6A; Skarnes et al., 2011). The targeting cassette containing Frt (Flip recombination sites)-flanked EnS2A, an IRES::lacZ trapping cassette, and a floxed neo cassette were inserted downstream of exon 2 to interfere with normal splicing of Agat pre-mRNA. Examination by RT-PCR (Figure 3—figure supplement 1A) and quantitative RT-PCR (Figure 3—figure supplement 1B) showed reduction of Agat mRNA in Agat+/- and absence of Agat mRNA in Agat-/- mice. Body weight (Figure 3—figure supplement 2B), but not brain weight (Figure 3—figure supplement 2A), of Agat-/- mice was lower than both Agat+/+ and Agat+/- mice, which were similar to Slc6a8 KO mice.
Immunoblot analysis showed SVs purified from the brains were not significantly different among Agat+/+, Agat +/-, and Agat-/- mice (Figure 3—figure supplement 3A–D), as supported by quantitative analysis of Syp, Syt, and H+-ATPase (n = 20, with two repeats for 10 samples).
We analyzed small molecules present in SVs from Agat+/+, Agat+/-, and Agat-/- mice. Cr was significantly enriched in SVs from all three genotypes compared to the IgG control (+/-). However, the level of Cr from Agat-/- mice was significantly lower than those from Agat+/+ and Agat+/- (n = 10, Figure 3A and B).
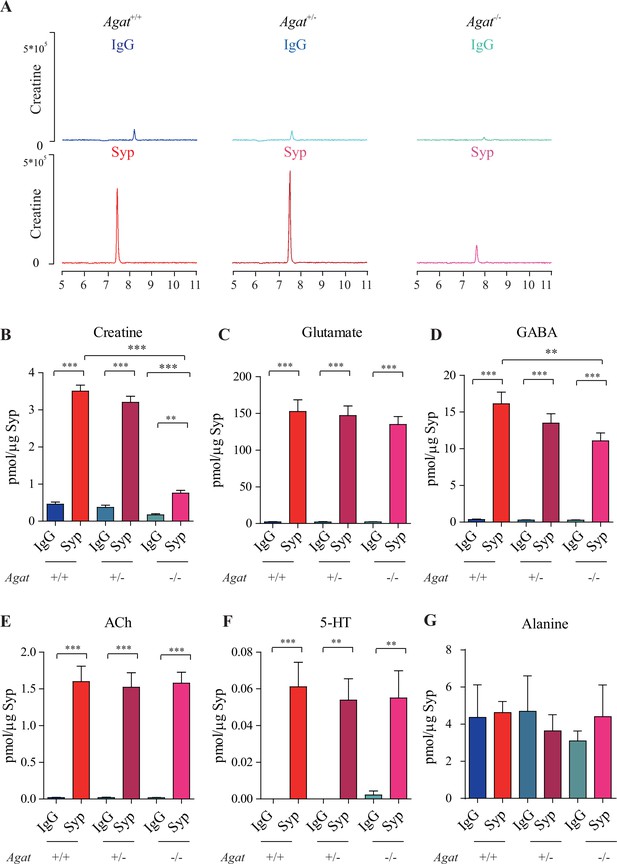
Reduction of synaptic vesicle (SV) creatine (Cr) in Agat knockout mice.
(A) Representative raw traces of Cr, pulled down by immunoglobulin G (IgG) or anti-synaptophysin (anti-Syp) from Agat+/+, Agat +/-, and Agat-/- mice. (B–G) Quantification of indicated molecules. Cr was significantly decreased in Agat-/- mice compared to Cr in Agat+/+ or Agat +/- mice (B). Gamma-aminobutyric acid (GABA) was significantly decreased in SVs from Agat-/- mice compared to Agat+/+ mice (D) but the difference was smaller than that of Cr. Glutamate (Glu) (C), acetylcholine (ACh) (E), 5-hydroxytryptamine (5-HT) (F), and alanine was not different among Agat+/+, Agat +/-, and Agat-/-mice. n = 10 samples per group. ***p<0.001, ns, not significant. One-way ANOVA with Tukey’s correction.
-
Figure 3—source data 1
Data for Figure 3B–G.
- https://cdn.elifesciences.org/articles/89317/elife-89317-fig3-data1-v1.xlsx
Glu (Figure 3C), ACh (Figure 3E), and 5-HT (Figure 3F) were all enriched in SVs (compared to IgG controls) and not significantly different among Agat+/+, Agat+/-, and Agat-/- mice. GABA in SVs from Agat-/- mice was also decreased from Agat+/+ mice by 30%, to an extent less than that of Cr (78.4%). Alanine was not different among three genotypes of mice (n = 6, Figure 3G). Thus, Cr and GABA, but not other neurotransmitters, in SVs were reduced in Agat KO mice.
Pattern of SLC6A8 expression indicated by knockin mice
We generated Slc6a8HA knockin mice by CRISPR/Cas9. Three repeats of the hemagglutinin (HA) tag (Kolodziej and Young, 1991), the T2A sequence (Ryan et al., 1991; Ahier and Jarriault, 2014; Daniels et al., 2014), and CreERT2 (Sauer and Henderson, 1989; Gu et al., 1993; Feil et al., 1997; Indra et al., 1999) were inserted in-frame at the C terminus of the SLC6A8 protein (Figure 4A).
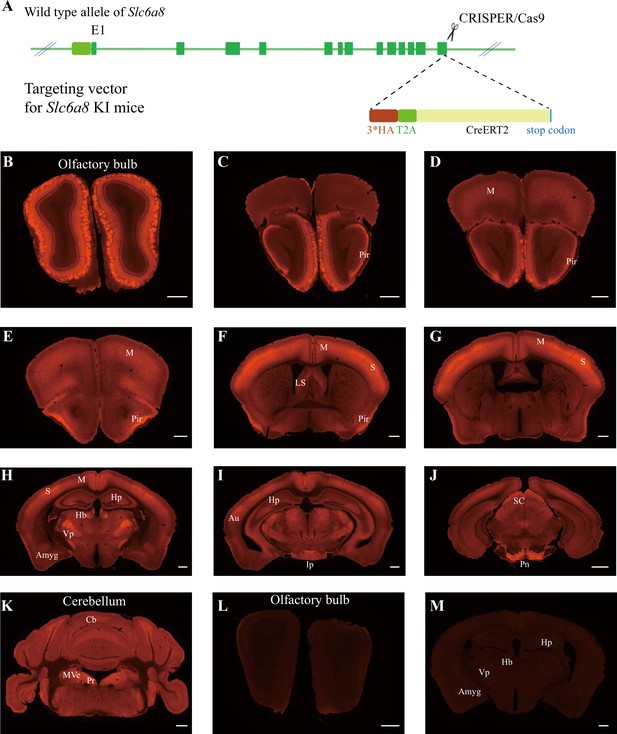
Expression pattern of SLC6a8.
(A) A diagram illustrating the generation of Slc6a8HA KI mice. 3*HA, T2A, and CreERT2 were inserted in-frame, before the stop codon, to the C terminus of SLC6A8 protein. (B–K) Low-magnification photomicrographs of coronal sections immunohistochemically labeled with the anti-HA antibody in Slc6a8HA mice. (I, M) immunostaining with the anti-HA antibody in control WT mice. Pir, piriform cortex; M, motor cortex; LS, lateral septum; Hp, hippocampus; Hb, habenular nucleus; Vp, ventral posterior nucleus of thalamus; Auauditory cortex; Amyg, amygdala; Ip, interpeduncular nucleus; Pn, pontine nucleus; Cb, cerebellum; Pr, prepositus; SC, superior colliculus; MVe, medial vestibular nucleus. Scale bar: 500 μm.
To examine the expression pattern of SLC6A8, we performed immunocytochemistry with an antibody against the HA epitope in Slc6a8HA and WT mice. Slc6a8HA mice showed positive signals in the olfactory bulb (Figure 4B), the piriform cortex (Figure 4C–F), the somatosensory cortex (Figure 4F and G), the ventral posterior thalamus (Figure 4H), the interpeduncular nucleus (Figure 4I), and the pontine nuclei (Figure 4J). In addition, moderate levels of immunoreactivity were observed in the motor cortex (Figure 4D–H), the medial habenular nucleus (Figure 4H), the hippocampus (Figure 4H), and the cerebellum (Figure 4K). These results were consistent with previous reports (Mak et al., 2009; Lowe et al., 2015). WT mice were negative for anti-HA antibody staining (Figure 4L and M).
Ca2+-dependent release of Cr upon stimulation
Classical neurotransmitters are released from the SVs into the synaptic cleft in a Ca2+-dependent manner after stimulation. For example, high extracellular potassium (K+) stimulated Ca2+-dependent release of Glu, GABA, and other neurotransmitters in brain slices (Hamberger et al., 1979b; Hamberger et al., 1979a; McBride et al., 1983; Nadler et al., 1977; Keith et al., 1993).
Thus, 300-μm-thick coronal slices of the mouse brain within 1–2 mm posterior to the bregma were used because the cortex, the thalamus, the habenular nucleus, and the hippocampus were positive for SLC6A8 (cf., Figure 4H). We monitored the effect of K+ stimulation by recording neurons in the slices. Immediately after K+ stimulation, pyramidal neurons in the CA1 region of the hippocampus were depolarized, firing a train of action potentials and reaching a large depolarization plateau in less than 1 min (Figure 5A). K+-induced depolarization persisted for several minutes before returning to the baseline and being washed within 10 min. Thus, superfusates in 1 min fraction at the time points of 1.5 min before (control) and after K+ stimulation, and 10 min after the wash were collected (Figure 5A), and the metabolites in the superfusates were analyzed by CE-MS.
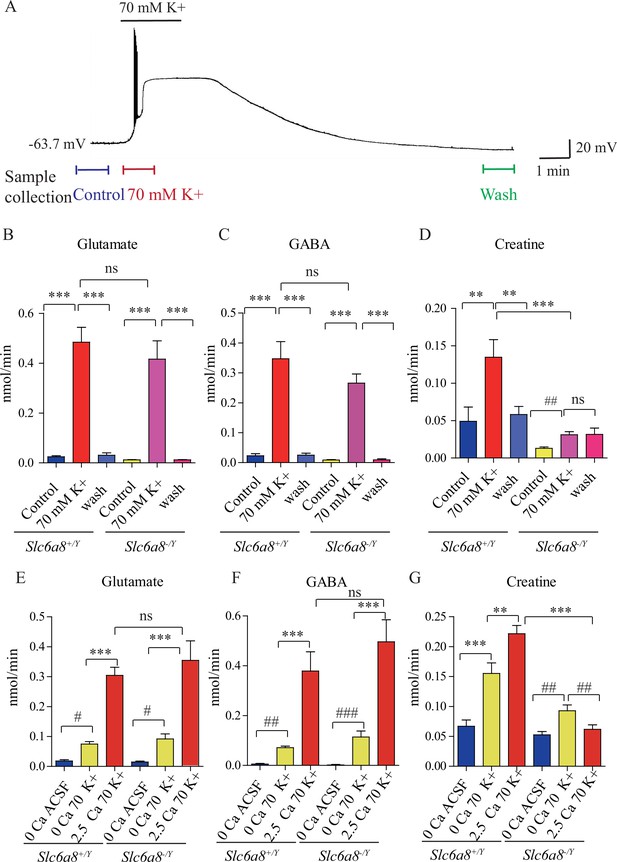
Creatine (Cr) release in brain slices from WT and Slc6a8 knockout mice.
(A) Neuronal depolarization induced by 70 mM K+ and time points for collecting the release sample. ‘Control’ samples were collected 1.5 min to 0.5 min before K+ stimulation, ‘70 mM K+’ artificial cerebrospinal fluid (ACSF) samples were collected during 70 mM K+ stimulation, and ‘wash’ samples were collected 10 min after washout with ACSF. Efflux of glutamate (Glu) (B), gamma-aminobutyric acid (GABA) (C), or Cr (D) from WT or Slc6a8 KO male mice (n = 7 samples per group). Note that a small amount of Cr released in Slc6a8 KO mice did not return to the baseline after 10 min of washing. (E–G) Ca2+-dependent release of Glu, GABA, and Cr in WT and Slc6a8 KO mice (n = 5 samples per group). ***p<0.001, ns, not significant. One-way ANOVA with Tukey’s correction. #p<0.05, ##p<0.01, paired t-test.
-
Figure 5—source data 1
Data for Figure 5B–G.
- https://cdn.elifesciences.org/articles/89317/elife-89317-fig5-data1-v1.xlsx
In the presence of Ca2+, depolarization with elevated extracellular K+ led to robust release of Glu and GABA in slices from WT (Slc6a8+/Y) mice (n = 7 per group, Figure 5B and C). After 10 min wash, levels of Glu and GABA returned to the baseline (Figure 5B and C). In the presence of Ca2+, depolarization with elevated K+ led to robust release of Cr. Extracellular Cr returned to the baseline after 10 min wash (Figure 5D). For quantification, the stimulated releases of metabolites were calculated by subtracting the basal levels from the total releases in response to K+ stimulation. In the presence of Ca2+, K+ stimulation induced the efflux of Glu, GABA, and Cr at 0.46, 0.33, and 0.086 nmol/min, respectively (n = 7 per group) (Figure 5B–D). From the detection limits of ACh and 5-HT in our system, we inferred that the efflux rate for ACh was lower than 0.001 nmol/min and that for 5-HT lower than 0.003 nmol/min. The efflux rate for Cr in brain slices is lower than those of Glu and GABA, but higher than those for ACh and 5-HT.
Ca2+ dependence of transmitter release was examined by comparing responses to ACSF without Ca2+ or elevated K+ (supplemented with 1 mM EGTA), elevated extracellular K+ in the absence of Ca2+ (supplemented with 1 mM EGTA), or K+ in the presence of 2.5 mM Ca2+ (Figure 5E–G, n = 5 per group). In the absence of Ca2+, elevated K+ stimulated the release of a small but significant amount of Glu and GABA, with efflux rates at 0.056 nmol/min and 0.066, respectively (Figure 5E and F). In the presence of 2.5 mM Ca2+, elevated K+ further augmented the release of Glu and GABA by 5–6 times, confirming previously reported Ca2+-dependent release of neurotransmitters in response to depolarization (Hamberger et al., 1979b; McBride et al., 1983).
Cr was also released both in a Ca2+-dependent and a Ca2+-independent manner (Figure 5G). More Cr was released in response to K+ stimulation in the presence of 2.5 mM Ca2+ than that in the absence of Ca2+. These results demonstrate Ca2+-dependent release of Cr upon stimulation.
Reduced Cr release in Slc6a8 and Agat mutant mice
We examined whether Slc6a8 KO affected K+-induced release of Cr. While Glu and GABA were released in slices from Slc6a8 KO (Slc6a8+/Y) mice at levels not significantly different from those of WT mice (Figure 5B and C), release of Cr in response to K+ stimulation was significantly reduced in Slc6a8-/Y mice compared to Slc6a8+/Y mice (Figure 5D). The basal level of Cr in Slc6a8 KO mice was lower than that of WT mice. In addition, K+ stimulation-induced release of Cr persisted to some extent even after 10 min of washout (Figure 5D), possibly due to the inability of presynaptic terminals in Slc6a8 KO mice to reuptake Cr in the synaptic cleft (Figure 8).
Experiments with slices from brains of Slc6a8 KO (Slc6a8-/Y) mice showed that Ca2+-dependent release of either Glu or GABA was not affected by the genotype of Slc6a8 (Figure 5E and F). By contrast, Ca2+-dependent release of Cr was abolished in Slc6a8-/Y slices. Interestingly, Ca2+-independent release of Cr was reduced by a third, but did not reach statistical significance, in Slc6a8-/Y slices. In the absence of Ca2+, the basal level of Cr was not changed in Slc6a8 KO mice. Taken together, these results indicate that there is Ca2+-dependent release of Cr upon stimulation and that SLC6A8 is required specifically for Ca2+-dependent release of Cr, but not for Ca2+-dependent release of other neurotransmitters such as Glu and GABA, or for Ca2+-independent release of Cr.
Knockout of Agat (Figure 6A) selectively reduced K+ evoked release of Cr, but not those of Glu or GABA (n = 5 per group, Figure 6B–D). Although K+ stimulation still elicited Cr release from brain slices of Agat+/-, the efflux rate in Agat-/- mice was reduced to less than 10% that in Agat+/+ mice and 20% that in Agat+/-.
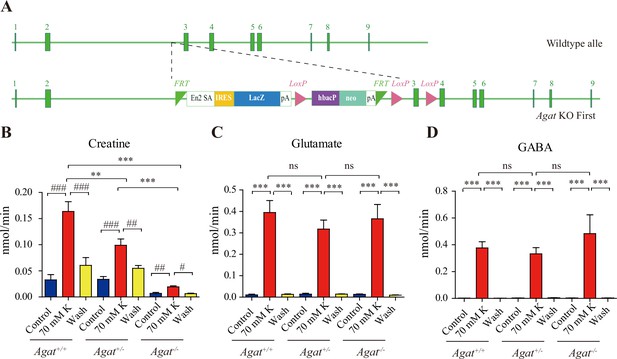
Creatine (Cr) release in WT and arginine:glycine amidinotransferase (AGAT) knockout mice.
(A) A schematic diagram illustrating the strategy of Agat knockout first. With the Agat gene (also known as Gatm) shown in the upper part, and the gene targeting strategy in the lower part. The homologous arm is approximately 10 kb. A targeting cassette, containing Frt-flanked lacZ and neomycin, was inserted downstream of exon 2. At the same time, exon 3 of Agat was flanked by two loxP sites. K+-induced release of glutamate (Glu) (C) and gamma-aminobutyric acid (GABA) (D) were not significantly different among Agat+/+, Agat+/-, and Agat-/- mice, whereas that of Cr (B) was significantly lower in AGAT-/- mice than those in Agat+/+ and Agat+/--.
-
Figure 6—source data 1
Data for Figure 6B–D.
- https://cdn.elifesciences.org/articles/89317/elife-89317-fig6-data1-v1.xlsx
Cr inhibition of neocortical neurons
Our own data (Figure 4H) and previous reports (Mak et al., 2009; Lowe et al., 2015) have shown SLC6A8 in the neocortex, with dense SLA6A8-HA immunoreactive fibers in layer 4 (Figure 7—figure supplement 1A; Eliseeva and Durinian, 1975; Yamawaki et al., 2021). Layer 5 neurons in the somatosensory cortex have been reported to express SLC6A8 previously (Mak et al., 2009; Lowe et al., 2015). To investigate electrophysiological effects of Cr, we performed whole-cell patch-clamp recordings from the pyramidal neurons in layer 4/5 of the somatosensory cortex (Figure 7A and B, Figure 7—figure supplement 1).
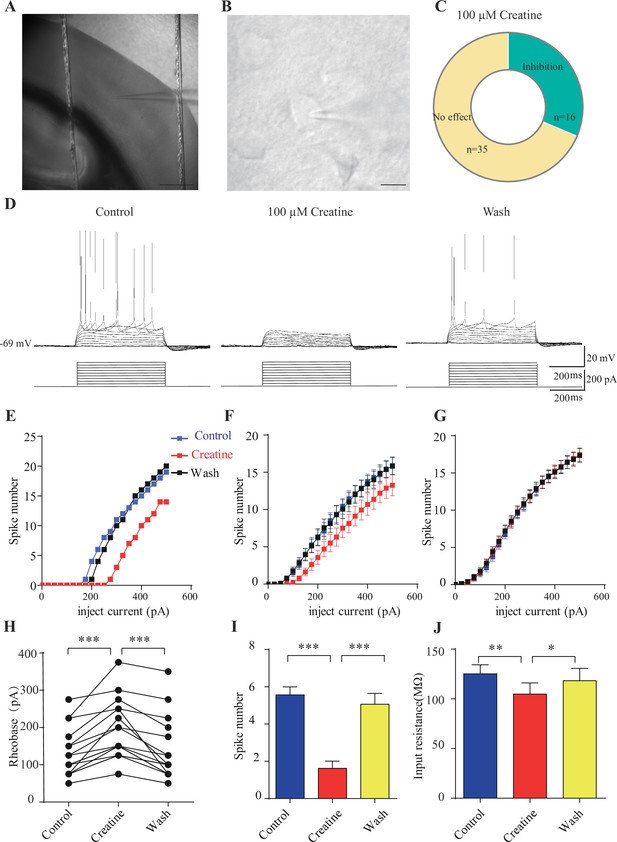
Inhibitory effects of creatine (Cr) on cortical neurons.
(A) A photograph showing recording at layer 4 in the somatosensory cortex. Scale bar: 10 μm. (B) Patch-clamp recording of a pyramidal neuron. Scale bar: 10 μm. (C) Ratios of Cr-responsive and -unresponsive neurons in the region. (D) Representative raw electrophysiological traces showing inhibition of evoked firing by Cr, with the lower panel showing the stimulus protocol. (E) Evoked spike numbers in response to different current injections from (D). (F) Relationship between evoked spike numbers and different current injections to neurons that were inhibited by Cr (n = 16). (G) The same for Cr-unresponsive neurons (n = 35). (H) Rheobase for Cr-responsive neurons. (I) Evoked spike number when these neurons were injected with current of rheobase + 50 pA. (J) input resistance. *p<0.05; **p<0.01; ***p<0.001, paired t-test.
-
Figure 7—source data 1
Data for Figure 7E–J.
- https://cdn.elifesciences.org/articles/89317/elife-89317-fig7-data1-v1.xlsx
Medium-sized pyramidal neurons (Figure 7B) with a membrane capacitance (Cm) of 114.96 ± 3.92 pF (n = 51, Figure 7—figure supplement 2A) were recorded. These neurons exhibited regular firing patterns (Scala et al., 2019; Stumpf et al., 2018) in response to depolarization current injection (Figure 7D, Figure 7—figure supplement 3A) with moderate maximal evoked spiking frequencies of 10–30 spikes per 500 ms (Figure 7E–G), increasing of inter-spike intervals during depolarizing steps (Figure 7D, Figure 7—figure supplement 3A), high action potential amplitude (81.64 ± 1.06 mV, Figure 7—figure supplement 2E), and large spike half-width (1.12 ± 0.031 ms, Figure 7—figure supplement 2F).
Cr was bath-applied only after the evoked firing pattern reached a steady state. Of the 51 neurons, 16 were inhibited by 100 μM creatine (Figure 7B–F). Fewer spikes were evoked in Cr-responsive neurons in response to depolarizing current injections during Cr application (25 pA step, 500 ms) (Figure 7D–F). The inhibitory effect of Cr was reversible (Figure 7D–F), typically observed within 2–3 min following Cr application (with maximal effect from 2 to 8 min) and disappeared after 10–25 min washout. This could be repeated by a second application of Cr. The rheobase, defined as the minimal electrical current necessary to elicit an action potential, was increased during bath application of Cr (Figure 7H). The inhibitory effect was most obvious at near spike threshold. When a neuron was depolarized with a current of 50 pA above rheobase, the number of evoked spikes was decreased dramatically during Cr application (Figure 7I). Cr also mildly inhibited the input resistance (Figure 7J), slightly hyperpolarized resting membrane potential (Figure 7—figure supplement 2C), or reduced amplitude of afterhyperpolarization (AHP) followed by the first evoked action potential (Figure 7—figure supplement 2G). The spike threshold (Figure 7—figure supplement 2D), amplitude (Figure 7—figure supplement 2E) and half width (Figure 7—figure supplement 2F) were not changed by Cr.
The remaining 35 neurons were not responsive to Cr (Figure 7C and G, Figure 7—figure supplement 3). Cr did not change electrical parameters tested, including evoked firing rates (Figure 7G, Figure 7—figure supplement 3A, B and D), rheobase (Figure 7—figure supplement 2C), resting membrane potential (Figure 7—figure supplement 2C), spike threshold amplitude (Figure 7—figure supplement 2E), and half width (Figure 7—figure supplement 2F). In addition, electrical properties of responsive neurons and unresponsive neurons were not significantly different. With the limited number of neurons recorded, the ratio of responsive neurons appeared higher in layer 4 or border of layer 4/5, than the deeper layer in layer 5 (Figure 7—figure supplement 1).
SLC6A8-dependent uptake of Cr into the synaptosomes
Along with enzymatic degradation, reuptake by transporters serves as an important way to remove neurotransmitters released into the synaptic cleft. As synaptosomes contain the apparatus for neurotransmission, they are often used for studying uptake of neurotransmitters (Gulyássy et al., 2020).
To investigate whether Cr uptake into synaptosomes required SLC6A8, we first examined whether SLC6A8 was present in synaptosomes. Using Slc6a8HA knockin mice and an anti-HA antibody, we found that Slc6a8-HA was present and enriched in crude synaptosomal fraction (P2 fraction in Figure 8A, enrichment score: P2/H = 1.76 ± 0.15, n = 4) and synaptosomal fraction prepared using a discontinuous Ficoll gradient (Sy and 4-Sy fractions in Figure 8A, enrichment score: Sy/H = 2.02 ± 0.14, n = 4). The integrity of synaptosomes was confirmed by multiple markers of the synaptosomes (Gulyássy et al., 2020), including the presynaptic membrane marker SNAP25 (Antonucci et al., 2016) and the SV marker Syp (synaptophysin Syp) (Jahn et al., 1985; Wiedenmann and Franke, 1985; Leube et al., 1987; Südhof et al., 1987) in presynaptic terminals, the postsynaptic density marker PSD95 (Woods and Bryant, 1991; Cho et al., 1992) and the postsynaptic membrane protein GluN1 (Cserép et al., 2012), the synaptic membrane protein SNAP23 (Kunii et al., 2021; Ravichandran et al., 1996; Suh et al., 2010), the plasma membrane marker Na+-K+-ATPase (Cameron et al., 1994; Mersel et al., 1987; Sun et al., 1988), and the mitochondria marker VDAC (Gulyássy et al., 2020). These were all enriched in our synaptosomal preparations. The cytosol marker GAPDH was also present in synaptosomes, whereas the oligodendrocyte marker MBP was nearly absent, suggesting that myelin pollution was largely avoided (Gulyássy et al., 2020).

Creatine (Cr) uptake into synaptosomes and synaptic vesicles (SVs).
(A) Markers for subcellular organelles detected in synaptosomes prepared from WT mice or mice with Slc6a8 gene fused in frame to the HA epitope. SLC6A8-HA was enriched in synaptosomes (Sy or 4-Sy) or crude synaptosomes (P2) (see ‘Materials and methods’), as were other markers for the subcellular organelles in synaptosomes but not the marker for myelin (MBP). (B) Representative electron micrographs and histograms of size distribution in synaptosomes isolated from WT (Slc6a8+/Y) and Slc6a8 KO (Slc6a8-/Y) mice by Ficoll density-gradient centrifugation. Sy, synaptosome; Mt, mitochondria; PSD, postsynaptic density. Bar, 20 nm. (C) Cr uptake into synaptosomes (n = 5 per group). The two left columns were results from WT mice and the two right columns from Slc6a8 knockout mice. The control baseline was [14C]-Cr uptake at 0°C at 10 min. Cr uptake into synaptosomes at 37°C measured at 10 min was observed in WT synaptosomes. Uptake into Slc6a8 knockout synaptosomes was significantly reduced compared to the WT synaptosomes. ***p<0.001, one-way ANOVA with Tukey’s correction. (D) uptake of [13C]-Cr into immunoisolated SVs in the presence or absence of ATP (n = 11 samples per group). *p<0.05, paired t-test.
-
Figure 8—source data 1
Original western blot files for Figure 8A.
- https://cdn.elifesciences.org/articles/89317/elife-89317-fig8-data1-v1.zip
-
Figure 8—source data 2
Labelled western blot files for Figure 8A.
- https://cdn.elifesciences.org/articles/89317/elife-89317-fig8-data2-v1.zip
-
Figure 8—source data 3
Labelled western blot files for Figure 8A.
- https://cdn.elifesciences.org/articles/89317/elife-89317-fig8-data3-v1.zip
We have also used EM to confirm the quality of our synaptosome preparations. As reported previously (Gulyássy et al., 2020; Schrimpf et al., 2005), synaptosomes were composed of membrane bounded structures (Sy in Figure 8B) filled with synaptic vesicles (SV in Figure 8B), sometimes with a segment of postsynaptic membrane along with the postsynaptic density (PSD in Figure 8B) and mitochondria (Mt in Figure 8B). The sizes of synaptosomes from WT mice and Slc6a8 knockout mice were similar, with areas of 0.245 ± 0.01 μm2 (n = 302 particles) and 0.247 ± 0.01 μm2 (n = 317 particles), respectively (Figure 8B).
We then examined whether SLC6A8 participated in Cr uptake into the synaptosomes. A mixture of 18 μM [14C]-Cr (with a total radioactivity of 0.4 μCi) and 5 μM Cr was used, and uptake at 0°C measured at 10 min was the baseline (Fykse and Fonnum, 1988). Cr uptake into synaptosomes from WT mice was stimulated approximately sevenfold at 37°C (Uptake, Figure 8C) compared to 0°C (Ctrl, Figure 8C). Cr uptake into synaptosomes from Slc6a8 knockout mice was less than three times compared to its control, and was decreased to approximately 1/3 of that of WT mice (Figure 8C). Thus, SLC6A8 is necessary for uptake of Cr into the synaptosomes.
Cr uptake into SVs
Classical neurotransmitters were taken up in SVs in an ATP-dependent manner (Burger et al., 1991; Cidon and Sihra, 1989; Schenck et al., 2009; Fykse and Fonnum, 1988; Bellocchio et al., 2000). We examined whether Cr could be transported into SVs.
We used 10 μg anti-Syp antibody to purify SVs from mouse brains. Purified SVs were preincubated for 30 min to allow sufficient leakage of endogenous Cr, before being mixed with 1 mM [13C]-Cr in the presence or absence of 4 mM ATP and placed at 25℃ for 10 min to allow adequate uptake. The SV content of [13C]-Cr was then examined by CE-MS and high-performance liquid chromatography-mass spectrometry (HPLC-MS). Significantly more [13C]-Cr were taken up by SVs in the presence of ATP, with about 10.3 pmol [13C]-Cr transported into SVs (1.03 pmol/μg α-Syp or transportation rate of 0.103 pmol/min, n = 11, Figure 8D).
In summary, Cr could be transported into SVs in an ATP-dependent manner. At this point, we do not know what is the transporter(s) on the SVs for Cr uptake. SLC6A8 is only found in plasma membrane, not on SVs, and is not a candidate for Cr uptake into SVs.
Discussion
While no neurotransmitter has been proven in a single paper, supportive evidence suggesting Cr as a possible new neurotransmitter has been presented here to the extent of any single previous papers.
At various times and by different researchers, taurine (Curtis and Watkins, 1960; Saransaari and Oja, 2008), proline (Felix and Künzle, 1974), D-aspartic acid (D’Aniello et al., 2011), hydrogen sulfide (Abe and Kimura, 1996), agmatine (Reis and Regunathan, 2000), DOPA (Misu et al., 2002), estradiol (Balthazart and Ball, 2006), β-alanine (Tiedje et al., 2010), and protons (Du et al., 2014) have been suspected as neurotransmitters, but they do not meet all the criteria. Some of the suspected molecules can be released upon stimulation or removed by transporters. Often, they have not been reproducibly found in SVs (Chantranupong et al., 2020).
Our discovery of Cr in SVs significantly raised the priority of testing the candidacy of Cr, and our further investigations have led to more evidence suggesting Cr as a neurotransmitter: (1) Cr is stored in SVs; (2) Ca2+-dependent release of Cr upon stimulation has been observed; (3) both Cr storage in SVs and Cr release are reduced when either the gene for Slc6a8 or the gene for Agat was deficient; and (4) Cr inhibits activities of pyramidal neurons in the neocortex; (5) Cr uptake into synaptosomes requires SLC6A8; and (6) Cr uptake into SVs was ATP-dependent.
Of the above results, 1, 3, 4, and 6 are reported for the first time in this article. Furthermore, we have demonstrated that detection of Cr in SVs was lower than those for Glu and GABA, but higher than those for ACh and 5-HT, placing Cr at a level in the middle of known central transmitters (Figures 1—3). The storage of Cr in SVs is dependent on preserved H+ gradient (Figure 1—figure supplement 2) and Cr can be transported into SVs (Figure 8D).
There was a single previous report of Ca2+-dependent release of [3H]Cr and endogenous Cr in response to electrical stimulation (Almeida et al., 2006). We now provide evidence that Cr was released in response to extracellular K+ stimulation (within 1–2 min) (Figures 5 and 6). Furthermore, Cr release was reduced when either the Slc6a8 or Agat gene was removed (Figures 5 and 6). Although the Ca2+-dependent component of K+-evoked Cr release was smaller compared to those of Glu and GABA, it nevertheless existed and was totally abolished by Slc6a8 knockout (Figure 5). The reported electrically evoked Cr release showed more Ca2+ dependence (Almeida et al., 2006). Taken together, our data and previous report (Almeida et al., 2006) supported a role of Cr as a neurotransmitter. Our observation of extremely low efflux rates of 5-HT or ACh may have arisen from very limited numbers of cholinergic (Li et al., 2018) or serotoninergic neurons (Ishimura et al., 1988) in the sliced sections and rapid enzymatic degradation of these neurotransmitters.
Cr uptake from the extracellular space into the cells was reported twice previously, once with brain slices showing sodium-dependent uptake of [3H]Cr (Almeida et al., 2006) and once with synaptosomes (Peral et al., 2010). Our new results have not only replicated the synaptosome Cr uptake experiment but also shown the requirement of SLC6A8, a membrane transporter expressed in synaptosomes (Figure 8A), for Cr uptake into synaptosomes. Transportation of Cr into synaptosomes by Slc6A8 may function for both the clearance of Cr from the synaptic cleft and recycling of Cr into SVs residing in neurons (Figures 6D and 8B).
In summary, in addition to confirming and extending previous results which have stood alone for more than a decade without replication or follow-up, we have obtained entirely new results suggesting the candidacy of Cr as a neurotransmitter. We discuss below the criteria for a neurotransmitter, Cr as a neurotransmitter, and the implications of Cr as a neurotransmitter.
Criteria of a neurotransmitter
The criteria for establishing a non-peptide small molecule as a neurotransmitter have varied from time to time and from author to author.
Some textbooks simply state that a neurotransmitter is stored presynaptically, released upon stimulation, and active on postsynaptic neurons. The details of these three criteria can vary. For example, one textbook stipulates that “the substance must be present within the presynaptic neuron; the substance must be released in response to presynaptic depolarization, and the release must be Ca2+ dependent; specific receptors for the substance be present on the postsynaptic cell” (Purves et al., 2001; Purves et al., 2016). Another states that “the molecule must be synthesized and stored in the presynaptic neuron; the molecule must be released by the presynaptic axon terminal upon stimulation; the molecule, when experimentally applied, must produce a response in the postsynaptic cell that mimics the response produced by the release of neurotransmitter from the presynaptic neuron” (Bear et al., 2016).
The neuroscience textbook most widely used internationally for the last four decades lists four criteria for a neurotransmitter (Kandel et al., 2013; Kandel et al., 2021): it is synthesized in the presynaptic neuron; it is present within vesicles and is released in amounts sufficient to exert a defined action on the postsynaptic neuron or effector organ; when administered exogenously in reasonable concentrations, it mimics the action of the endogenous transmitter; and a specific mechanism usually exists for removing the substance from the synaptic cleft. These are similar, but not identical, to the classic textbook on neurotransmitters: a neurotransmitter “should be synthesized and released presynaptically; it must mimic the action of the endogenous compound that is release on nerve stimulation; and where possible, a pharmacological identity is required where drugs that either potentiate or block postsynaptic responses to the endogenously released agent also act identically to the suspected neurotransmitter that is administered” (Cooper et al., 2002). The pharmacological criterion is listed in another textbook (Squire et al., 2012).
Some authors note difficulties in establishing a CNS neurotransmitter. For example, a specialized neurotransmitter book states that “the candidate neurotransmitter should be present in the presynaptic terminal, be released when the presynaptic terminal is active, and when applied experimentally, induce faithful responses in the postsynaptic neuron. In practice, since central nervous system neurons continuously integrate diverse excitations and inhibitions, the last criterion is relaxed to demonstrating merely changes in such activity” (Robinson, 2001).
Solomon Snyder, a leading scientist of classic neurotransmitters, neuropeptides and their receptors, wrote that “designating a molecule as a transmitter depends on the criteria employed, the most common of which are that the substance is synthesized in neurons, released by their terminals, mimics the effects of physiologic neurotransmission and possess a mechanism for inactivation. However, with each new candidate the rules have been modified and broadened” (Barañano et al., 2001).
Evidence supporting Cr as a neurotransmitter
Sixteen small molecules have been listed as neurotransmitters in the classic textbook (Kandel et al., 2013; Kandel et al., 2021). Among them, adenosine, arachidonic acid, nitric oxide, and carbon monoxide do not meet all four criteria at present. Cr appears to be better than these in meeting the criteria for a central neurotransmitter.
The results obtained by us in this article have satisfied the criteria of Robinson, 2001 for Cr to be a CNS neurotransmitter.
The four criteria of Snyder and colleagues (Barañano et al., 2001) have been mostly met but the physiological neurotransmission would require more research because a specific synapse(s) would have to be defined and studied for putative creatinergic neurotransmission. This can take much longer in the CNS than the PNS. Some commonly accepted neurotransmitters have never satisfied this criterion in a strict sense. The mechanism of Cr removal criterion is met not only by the Cr uptake in brain slices (Almeida et al., 2006) and in synaptosomes (Peral et al., 2010), but also by our demonstration that SLC6A8 is required for synaptosome uptake of Cr.
The four criteria of Kandel et al., 2013 and Kandel et al., 2021 are mostly satisfied with some details requiring further research. The synthesis requirement is usually not strict because there are transmitters synthesized in some cells and transported into others where they function as transmitters. Our discovery of Cr in SVs can replace the synthesis requirement because the presence in neuronal SVs provide sufficient evidence that Cr is located in the right location to function as a neurotransmitter. The level of Cr in SVs is higher than those of ACh and 5-HT (Figures 1 and 2). The amount of released Cr is in the same order of magnitude as those of Glu and GABA (Figures 5 and 6). The criterion of a specific mechanism of removal was met by Cr uptake experiments in slices (Almeida et al., 2006) and in synaptosomes (Peral et al., 2010), and further strengthened by our finding of SLC6A8 involvement in synaptosome uptake of Cr (Figure 8).
Here, we report that Cr, at a concentration comparable to classical neurotransmitters, inhibits pyramidal neurons in specific regions of the mouse brain, with approximate 1/3 of pyramidal neurons responding to 100 μM Cr (Figure 7). In previous reports, 100 μM to 2 mM of GABA (Brown and Scholfield, 1979; Pringle et al., 1996; Osmanović and Shefner, 1990), 50 μM to 2 mM of Glu (Akaike et al., 1987; Cole et al., 1989), 1–100 μM of DA (Akaike et al., 1987), and 0.1–100 μM of 5-HT (Beck and Goldfarb, 1985; Reynolds et al., 1988; Mendiguren et al., 2022; Xie et al., 2012; Gorelova and Reiner, 1996; Okuhara and Beck, 1994) were bath-applied to investigate the physiological functions of neurotransmitters. Our results revealed that, when bath-applied, Cr could inhibit cortical neurons at 100 μM within several minutes, with a time course similar to that of 5-HT (Sizer et al., 1992; Xie et al., 2012; Okuhara and Beck, 1994) and DA (Akaike et al., 1987), but significantly slower than that of Glu (Cole et al., 1989), GABA (Roberts and Frankel, 1950; Pringle et al., 1996), and 5-HT (Mendiguren et al., 2022).
In a recent report, knockout of the Slc6a8 gene increased excitation of cortical neurons (Ghirardini et al., 2023). Electrophysiological characterization of pyramidal neurons in the prefrontal cortex (PFC) found increased evoked firing frequency. Because we have shown that Cr inhibit a fraction of pyramidal neurons in the neocortex (Figure 8), this article provides in vivo evidence consistent with the possibility of Cr as an inhibitory neurotransmitter.
Differences between Cr and classic neurotransmitters
At this point, we do not have a molecularly defined receptor for Cr, only inferring its presence from the electrophysiological responses to Cr. We speculate that Cr may act on G-protein-coupled receptors (GPCRs), rather than the fast-acting ligand-gated ion channels, such as AMPA or NMDA receptors for Glu and GABAA receptor for GABA. There have been previous reports of Cr effects on neurons, including Cr as a partial agonist for GABAA receptors (De Deyn and Macdonald, 1990; De Deyn et al., 2001; Neu et al., 2002; Koga et al., 2005). These effects require very high concentrations of Cr (in the 10 mM range). There was also a report of the opposite effect: that Cr (at a concentration above 500 μM) increased neuronal excitability through NMDA receptors after incubation for 60 min, with a time course significantly slower than those of classic neurotransmitters (Royes et al., 2008).
Ca2+-independent component of Cr release induced by extracellular K+ was more prominent than those of Glu or GABA. One possibility was that Ca2+-independent Cr release came from glia because high GAMT levels were reported in astrocytes (Schmidt, 2004) and oligodendrites (Schmidt, 2004; Rosko et al., 2023). As reported, other neuromodulators such as taurine can be released from astrocytes (Philibert et al., 1989) or slices (Saransaari and Oja, 2006) in a Ca2+-independent manner. In addition, in the absence of potassium stimulation, Ca2+ depletion increased release of taurine in cultured astrocytes (Takuma et al., 1996) or in striatum in vivo (Molchanova et al., 2005). Similarly, in Slc6a8 KO slices, Ca2+ depletion (Figure 5G) also increased Cr baseline compared to that in normal ACSF (Figure 5D).
With much longer history of research, ACh and 5-HT now have more evidence in other aspects than Cr as a central transmitter, especially because there are many agonists and antagonists for ACh and 5-HT to prove an additional criterion that is required in some (Cooper et al., 2002; Squire et al., 2012), but not the majority of, textbooks for a neurotransmitter. The pharmacology criterion will take some time and effort because so far no effort has been made to find agonists or antagonists for Cr.
Implications of SLC6A8 and Cr
It is notable that SLC6A8 belongs to the NTT family, with multiple members already shown to transport neurotransmitters (Pacholczyk et al., 1991; Blakely et al., 1991; Guastella et al., 1992; Clark et al., 1992; Borden et al., 1992; Lopez-Corcuera et al., 1992; Giros et al., 1991; Kilty et al., 1991; Shimada et al., 1991; Hoffman et al., 1991; Smith et al., 1992; Liu et al., 1992).
The uptake experiments by others and us indicate that SLC6A8 transports Cr into neurons within the brain. AGAT is also expressed in the brain, but in cells not expressing SLC6A8 (Braissant and Henry, 2008; Braissant et al., 2010). Cr and its precursor were thought to be transported between different cells in the nervous system. When SLC6A8 was completely missing, such as in homozygous SLC6A8-deficient patients, Cr treatment was not effective. But if SLC6A8 was partially active, Cr was effective (Dunbar et al., 2014). Intractable epilepsy in a female with heterozygous SLC6A8 mutation was completely treated by Cr (Mercimek-Mahmutoglu et al., 2010). Our data of inhibitory effect of creatine on cortical neurons might provide a new mechanism to its anti-epileptic activity (Gerbatin et al., 2019).
The absence of SLC6A8 expression in astrocytes whose endfeet lining microcapillary endothelial cells (MCEC) form the blood–brain barrier (BBB) indicates that Cr in the brain does not rely on import from the periphery and is instead mainly synthesized in the brain (Braissant et al., 2011; Braissant et al., 2001; Braissant, 2012). SLC6A8 functions within the brain to transport Cr and its precursors not as a major contributor of Cr transport across the BBB. It is thought to mediate Cr uptake into the presynaptic terminal based on studies of synaptosomes (Figure 8; Peral et al., 2010).
Cr is known to have effects other than an energy source, and Cr supplement has been thought to be beneficial for children, pregnant and lactating women, and old people (Wallimann et al., 2011; Brosnan and Brosnan, 2016). Cr has been reported to improve human mental performance (Watanabe et al., 2002; Rae et al., 2003; McMorris et al., 2006; McMorris et al., 2007; Rae and Bröer, 2015; Wallimann and Harris, 2016). Cr has been used as potential treatment in animal models of neurodegenerative diseases (Andreassen et al., 2001; Andres et al., 2005).
Our work will stimulate further research to distinguish which of the previously suspected effects of Cr is not attributed to its role as an energy storage, but can be attributed to its role as a neurotransmitter.
Search for new neurotransmitters
Our work may stimulate the search for more neurotransmitters. Our discovery indicates that the hunt for neurotransmitters stopped decades ago because of technical difficulties not due to the absence of more neurotransmitters. The fact that most of the known small-molecule neurotransmitters have been found because of their peripheral effects also argues that what is missing is the concerted efforts to uncover central neurotransmitters with no peripheral effects. New neurotransmitters may be discovered from candidates which have been long suspected and from previously unsuspected molecules or even previously unknown molecules.
Innovative approaches should be taken to uncover molecules with no previous suspicions or hints. Highly purified SVs, SVs from different regions of the brain, and SVs with specific SLCs offer some of the starting points for future research.
Materials and methods
Generation of knockout and knockin mice
Request a detailed protocolSlc6a8 knockout and knockin mice were generated using CRISPR-Cas9-mediated genome engineering techniques by Beijing Biocytogen (Beijing, China). Agat ‘knockout-first’ (Skarnes et al., 2011) mice were purchased from CAM-SU GRC (Suzhou, China). All mutations were validated by Southern blot analysis, tail junction PCR, and DNA sequencing. Transgenic mice will be provided upon request.
RT-PCR and qPCR
Request a detailed protocolTotal RNA of whole brains from mice of different genotypes was extracted using the Buffer RZ (Tiangen, no. RK14, Beijing, China) and reverse transcribed into complementary DNA (cDNA) using the RevertAid First-Strand cDNA synthesis kit (Thermo Scientific, K1622). qPCR was performed using the Taq Pro Universal SYBR qPCR Master Mix (Vazyme, Q712-02) on Bio-Rad CFX-96 Touch Real-time PCR System (Bio-Rad, USA). Glyceraldehyde-3-phosphate dehydrogenase (Gapdh) was used as an internal control. ΔCt (difference in cycle threshold) was calculated for each sample (ΔCt = Ct Target gene − Ct GAPDH) for further evaluation of relative mRNA expression levels in different genotypes. The sequence specificities of the primers were examined. Three pairs of primers targeting different genes were used: Slc6a8 forward, 5′-GTCTGGTGACGAGAAGAAGGG-3′, Slc6a8 reverse, 5′-CCACGCACGACATGATGAAGT-3′; Agat forward, 5′-cacagtggaggtgaaggccaatacatat-3′, Agat reverse, 5′-ccgcctcacggtcactcct-3′; Gapdh forward, 5′- AGGTCGGTGTGAACGGATTTG-3′, Gapdh reverse, 5′-TGTAGACCATGTAGTTGAGGTCA-3′.
Primers for reverse PCR were designed to obtain complete coding sequences based on information obtained from the National Center for Biotechnology Information (NCBI): Slc6a8 forward, 5′-atggcgaaaaagagcgctgaaaacg-3′; Slc6a8 reverse, 5′-ttacatgacactctccaccacgacgacc-3′; Agat forward, 5′- atgctacgggtgcggtgtct-3′; Agat reverse, 5′-tcagtcaaagtaggactgaagggtgcct-3′. PCR products were electrophoresed on 1% agarose gels, stained with GelRed, visualized under UV illumination, and photographed.
Immunoblot analysis
Request a detailed protocolSamples were loaded onto 10% polyacrylamide gels with the PAGE system (#1610183, Bio-Rad Laboratories, USA) and run in the SDS running buffer (25 mM Tris, 192 mM glycine, 0.1% SDS, pH 8.8) for 25 min at 80 V followed by 25–45 min at 200 V. Afterward, proteins were transferred to immobilon NC transfer membranes (HATF00010, Millipore) at 400 mA for 2 hr in transfer buffer (25 mM Tris, 192 mM glycine, 20% methanol). Membranes were blocked in 5% fat-free milk powder in TBST (25 mM Tris, 150 mM NaCl, 0.2% Tween-20 [P1397, Sigma, St. Louis, MO], pH 7.4 adjusted with HCl) and incubated overnight with the indicated primary antibodies dissolved in TBST containing 2% BSA.
Primary antibodies are listed below: rabbit anti-synaptophysin (dilution 1:5000, cat. no. 101002, synaptic systems [SySy], Goettingen, Germany), rabbit anti-synaptotagmin1/2 (dilution 1:2000, cat. no. 105002, SySy), rabbit anti-proton ATPase (dilution 1:1000, cat. no. 109002, SySy), rabbit anti-synaptobrevin 2 (dilution 1:5000, cat. no. 104202, SySy), rabbit anti-SV2A (dilution 1:2000, cat. no. 109003, SySy), rabbit anti-VGlut1 (dilution 1:4000, 135302, SySy), rabbit anti-VGlut2 (dilution 1:2000, 135402, SySy), rabbit anti-VGAT (dilution 1:4000, 131002, SySy), rabbit anti-SNAP23 (dilution 1:2000, cat. no. 111202, SySy), mouse anti-PSD95 (dilution 1:5000, cat. no. 75028, NeuroMab, Davis, CA), mouse anti-GluN1(dilution 1:5000, cat. no. 114011, SySy), rabbit anti-GM130 (dilution 1:1000, cat. no. ab52649, Abcam), rabbit anti-Golgin-97 (dilution 1:2000, cat. no. 13192, Cell Signaling Technology, MA), rabbit anti-EEA1 (dilution 1:2000, cat. no. 3288, Cell Signaling Technology), rabbit anti-LC3B (dilution 1:1000, cat. no. 2775S, Cell Signaling Technology), goat anti-CathepsinB (dilution 1:2000, AF965, R&D Systems, Minneapolis, MN), rabbit anti-GAPDH (dilution 1:1000, cat. no. 2118S, Cell Signaling Technology), rabbit anti-GluT4 (dilution 1:1000, ab33780, Abcam, Cambridge, UK), rabbit anti-CACNA1A (dilution 1:300, 152103, SySy), rabbit anti-VDAC (dilution 1:1000, cat. no. 4661S, Cell Signaling Technology), rabbit anti-MBP (1:1000, cat. no. 295003, SySy), mouse anti-Creatine Kinase B (dilution 1:5000, cat. no. MAB9076, R&D Systems), rabbit anti-HA (dilution 1:2000, CST3724, Cell Signaling Technology), and rabbit anti SNAP25 (dilution 1:2000, ab109105, Abcam, UK) antibodies.
Membranes were washed in three washing steps in TBST (each for 5 min) and incubated with peroxidase-conjugated secondary antibodies for 2–3 hr at 4°C. The second antibodies used were anti-rabbit (dilution 1:5000, A6154, Sigma), anti-mouse (dilution 1:5000, 715-035-150, Jackson ImmunoResearch, West Grove, Philadelphia, USA) or rabbit anti-goat IgG secondary antibodies (dilution 1:1000, cat. no. ab6741, Abcam). After repeated washing, signals were visualized using a ChemiDoc XRS + System (Bio-Rad Laboratories).
Isolation of synaptic vesicles
Request a detailed protocolOur purification procedures for SVs were based on previously established immunoisolation methods (Burger et al., 1989; Martineau et al., 2013). Protein G magnetic beads (cat. no. 88848, Thermo Fisher Scientific, Waltham, MA) were washed three times with IP buffer (100 mM potassium tartrate, 4 mM HEPES-KOH, 2 mM MgCl2, pH 7.4) supplemented with a complete protease inhibitor cocktail (Roche, Basel, Switzerland). Then, 5 μg monoclonal anti-Syp antibody directed against a cytoplasmic epitope (cat. no. 101011, SySy) or control mouse IgG (10400C, Thermo Fisher Scientific) was used to incubate with 20–30 μl beads for 30 min at RT in 2% BSA dissolved in IP buffer. Under this condition, 4–4.5 μg of antibody was coupled, as determined by western blot and Coomassie Blue staining. Immunoisolation of SVs was carried out at 0–2°C to prevent vesicular content leakage (with RT as a control). Briefly, the whole mouse brain was homogenized in 3 ml of IP buffer with a glass/Teflon homogenizer (20 strokes at 2000 rpm, WHEATON, USA, and WIGGENS WB2000-M, Germany) immediately after decapitation. Homogenates were centrifuged for 25 min at 35,000 × g, and the supernatant was adjusted to approximately 3 mg/ml protein (NanoDrop 2000C, Thermo Fisher Scientific). To capture the SVs for content detection, about 200 μl of supernatants (per 5 μg anti-Syp/IgG) was incubated with pre-coupled beads for 2.25 hr under slow rotation at 2°C. Beads were washed six times for further western blot analysis and vesicular content detection. For pharmacological blockade of H+-gradient across SV membrane, the mix of supernatants and pre-coupled beads was diluted into 1.2 ml before the addition of inhibitors.
Determination of vesicular contents
Request a detailed protocolTo extract SV contents, immunoisolates were treated with 50 μl ultra-pure water. Then, 100 μl methanol together with 100 μl acetonitrile was added to precipitate proteins in samples. After centrifugation for 20 min at 16,8000 × g, supernatants were collected and centrifuged for 20 min at 2000 × g to remove beads and proteins. Samples were pre-frozen with liquid nitrogen and vacuum dried at –45°C overnight. Dried samples were kept frozen and resuspended with 50 μl of 0.2 μM 13C-creatine (internal control) immediately before detection.
CE-MS was used to verify and quantify small molecules. CE/MS detection was applied with the coupling of PA800 plus CE system (Beckman Coulter, Brea, CA) and mass spectrometry (TRIPLE QUAD 5500, AB SCIEX or Q Exactive HF-X, Thermo Scientific). Before SV content detection, we optimized MS detection of classical neurotransmitters, Cr, and amino acids in positive ion mode. Firstly, the fragment ions (Q3) for a given molecule (precursor ions, Q1) were determined by either systematic scanning of standard sample solution (0.1 μM in 10% acetate acid) or referring to database (https://www.mzcloud.org). Secondly, optimal values of collision energy (CE), collision cell exit potential (CXP), and declustering potentials (DP) were determined for each pair of Q1/Q3. Thirdly, optimal combination of parameters (Q1/Q3, CE, CXP, DP) was chosen for each molecule. In addition, parameters were adjusted every 2–3 mo for best signal-to-noise ratios.
CE/MS separations were carried out by capillaries (OptiMS silica surface cartridge, Beckman Coulter). The CE background electrolyte was 10% acetate acid. Each new separation capillary was activated with rinsing under 100 psi sequentially with methanol for 10 min (forward), methanol for 3 min (reverse), H2O for 10 min (forward), H2O for 3 min (reverse), 0.1 M NaOH for 10 min (forward), water for 5 min (reverse), 0.1 M HCl for 10 min (forward), followed by water for 10 min and then 10% acetate acid for 10 min (forward) and 3 min (reverse), prior to the first use. Between analyses, the capillary was rinsed with 10% acetate acid under a 100 psi pressure for 5 min (forward) flowed by 75 psi for 4 min. The sample (50 μl) was injected with 2.5–4 psi for 30 s. Separation voltage of 25 kV was applied for 25 min. To maintain stably spray during CE separation, ion spray voltage was applied at 1.7–1.9 kV. MS data were collected 5 min after CE separation. Finally, the capillary was washed with 10% acetate acid for 10 min, followed by methanol for 20 min and then 10% acetate acid for 20 min.
Standard solutions of 0.2 μM 13C-Cr (internal control) and analytes were used to plot standard curves. Linear standard curves (R2 > 0.98, for most cases, R2 > 0.99), calculated from peak area ratios corresponding to analytes and internal standards, were obtained for all molecules tested. The concentration ranges used for standards of Glu, GABA, ACh, 5-HT, Cr, and alanine were 0.03–10 μM, 0.003–1 μM, 0.0003–0.1 μM, 0.003–1 μM, 0.03–1 μM, and 0.03–1 μM, respectively. Standard curves were made at least twice for a given capillary. Analytes of SV contents were calculated using the standard curves and normalized to the amount of anti-Syp antibody conjugated to the beads.
Electron microscopy
Request a detailed protocolAll EM grids were glow discharged for 30 s using a plasma cleaner (Harrick PDC-32G-2, plasma cleaners, Ithaca, NY). To free SVs from beads, 25 μl 0.1 M glycine-HCl (PH = 2) was incubated for 1 min and quickly neutralized with 25 μl 0.1 M Tris (pH = 10). Beads were quickly removed and 2–4 μl aliquots of SVs were applied to the carbon-coated copper grids (Zhong Jing Ke Yi, Beijing, China). After 1 min, the grid was dried with a filter paper (Whatman No. 1), and placed in the water, and then immediately stained using 2% uranyl acetate for 30 s. At last uranyl acetate was removed and the grid was air dried. The grids were examined on a JEM-F200 electron microscope (JEOL, Tokyo, Japan) operated at 200 kV. Images were recorded using a 4k × 4k COMS One view camera (Gatan, Abingdon, UK). Fixation of synaptosomal pellets was performed by immersion with pre-warmed 2.5% glutaraldehyde in 0.1 M phosphate buffer (pH 7.4) at RT for 2 hr. After washing four times with 0.1 M phosphate buffer (pH 7.4) every 15 min, samples were post-fixed with 1% osmium tetroxide (w/v) at 4°C for 1 hr and then washed three times. Following en bloc staining with 2% uranyl acetate (w/v) at 4°C overnight, samples were dehydrated and embedded in fresh resin, polymerized at 65°C for 24 hr. Ultrathin (70 nm) sections were obtained using Leica UC7 ultramicrotome (Leica Microsystems, Wetzlar, Germany) and recorded on 80 kV in a JEOL Jem-1400 transmission electron-microscope (JEOL) using a CMOS camera (XAROSA, EMSIS, Munster, Germany).
Immunohistochemistry
Request a detailed protocolAdult mice were anesthetized by i.p. injection with 2% 2,2,2-tribromoethanol (T48402, Sigma) in saline at a dose of 400 mg/kg and perfused trancardially with 0.9% saline, followed by 4% PFA in PBS (137 mM NaCl, 2.7 mM KCl, 10 mM Na2HPO4, 1.8 mM KH2PO4, pH = 7.4).
Brains were cryoprotected with 30% sucrose in 30% sucrose 0.1 M PB (81 mM Na2HPO4, 19 mM NaH2PO4) and sectioned in the coronal plane (40 μm thick) using a Cryostat (Leica 3050S). For anti-HA immunostaining, we used a rabbit monoclonal anti-HA antibody (1:500 in 0.3% Triton in PBS; 48 hr incubation at 4°C; #3724, Cell Signaling Technology), followed by a goat anti-rabbit Alexa Fluor 546 secondary antibody (1:1000; overnight at 4°C; # A-11035, Invitrogen, Waltham, MA). Sections were mounted in a medium containing 50% glycerol, cover-slipped, and sealed with nail polish. Images were acquired using virtual slide microscope (Olympus VS120-S6-W, Tokyo, Japan) and a laser-scanning confocal microscope (Zeiss 710, Cambridge, UK) and brain structures inferred with an established mouse brain atlas (Paxinos and Franklin., 2013).
Preparations of brain slices
Request a detailed protocolMale C57 mice (of 30–38 days old) were anesthetized with pentobarbital (250 mg/kg) and decapitated. Brains were quickly removed and placed into ice-cold, low-calcium, high-magnesium artificial cerebrospinal fluid (ACSF) with sodium replaced by choline. The medium consisted of 120 mM choline chloride, 2.5 mM KCl, 7 mM MgSO4, 0.5 mM CaCl2, 1.25 mM NaH2PO4, 5 mM sodium ascorbate, 3 mM sodium pyruvate, 26 mM NaHCO3, and 25 mM D-(+)-glucose, and was pre-equilibrated with 95% O2–5% CO2. Coronal brain slices (300 μm thick) were cut with a vibratome (Leica VT1200S). Slices were incubated for 1 hr at 34°C with oxygenated ACSF containing 124 mM NaCl, 2.5 mM KCl, 2 mM MgSO4, 2.5 mM CaCl2, 1.25 mM NaH2PO4, 26 mM NaHCO3, and 10 mM D-(+)-glucose.
Evoked release from brain slices
Request a detailed protocolCoronal brain slices (each 300 μm thick, typically with a wet weight of 17–20 mg) were transferred into a specially designed superfusion chamber with a volume of approximately 200 μl, containing freshly 95% O2/5% CO2 oxygenated ACSF. Slices were equilibrated for 10 min in ACSF at a superfusion rate of 0.9–1.25 ml/min. The ‘control’ sample was collected for 1 min just before high K+ stimulation (K-ACSF, 70 mM KCl replacing equal amount of NaCl). We waited for 30 s to allow K+ stimulus to immerse the slices (dead volume for solution transition of 200 μl and chamber volume of 200 μl), then the sample ‘70 mM K’ in response to K-ACSF was collected for another 1 min. Following 10 min of washout period, we collected the third sample of ‘wash’ for 1 min.
To detect Ca2+-dependent release, slices were pre-incubated for 10 min with normal ACSF and equilibrated with Ca2+-free ACSF (containing 1 mM EGTA to chelate extracellular Ca2+) for 10 min. The baseline sample ‘0 Ca2+ ACSF’ was collected for 1 min. Superfusion solution was changed to Ca2+-free K-ACSF for 2 min and sample ‘0 Ca2+ 70 mM K’ was collected (dead volume for solution transition of 400 μl and chamber volume of 200 μl). Then, the solution was changed back to normal ACSF for 10 min and K-ACSF for 2 min. The sample ‘2.5 mM Ca 70 mM K’ for the last minute was collected.
Samples were subjected to CE-MS in a method similar to SV content detection, except for the following: (1) standards were dissolved in ACSF or other buffers used in release experiment; (2) concentration ranges used for standards of Glu was from 0.003 to 1 μM; and (3) to protect the MS from salt pollution, data were collected from 10 to 20 min during CE separation.
Patch-clamp recordings
Request a detailed protocolSlices were transferred to a recording chamber on an upright fluorescent microscope equipped with differential interference contrast optics (DIC; Olympus BX51WI). Slices were submerged and superfused with ACSF at about 2.8 ml/min at 24–26°C. Whole-cell patch recordings were routinely achieved from layer 4/5 medium-sized pyramidal neurons from the somatosensory cortex. Patch pipettes (3–5 MΩ) contained 140 mM K-gluconate, 10 mM HEPES, 0.5 mM EGTA, 5 mM KCl, 3 mM Na2-ATP, 0.5 mM Na3GTP, and 4 mM MgCl2 (with pH adjusted to 7.3 and osmolarity of 290 mOsm/kg). Current-clamp recordings were carried out with a computer-controlled amplifier (Multiclamp 700B, Molecular Devices) and traces were digitized at 10 kHz (DigiData 1550B, Molecular Devices). Data were collected and analyzed using Clampfitor Clampex 10 software (Molecular Devices).
Cells were characterized by their membrane responses and firing patterns during hyperpolarizing and depolarizing current steps (–100 to +500 pA, increment: 50 pA or 25 pA, 500 ms). Regular spiking pyramidal neurons were identified by moderate maximal spiking frequencies (20–60 Hz, i.e., 10–30 spikes per 500 ms, Figure 7E–G), increasing of inter-spike intervals during depolarizing step (Figure 7B, Figure 7—figure supplement 3A), high action amplitude (Figure 7—figure supplement 2E), and large half width (Figure 7—figure supplement 2F; Scala et al., 2019; Stumpf et al., 2018). After the mean firing frequency evoked by current injections reached the steady state for at least 5 min (typically 20–30 min following the formation of whole-cell configuration), 100 μM Cr was bath-applied for 6 min. Typically, Cr was applied for a second time following washout to reconfirm the effects.
Synaptosome preparation
Request a detailed protocolSynaptosomes were isolated by Ficoll/sucrose density-gradient centrifugation (Gulyássy et al., 2020; Schrimpf et al., 2005; Peral et al., 2010; Booth and Clark, 1978). Whole brains from adult male mice were homogenized with 15 strokes at 900 rpm in buffer A (320 mM sucrose, 1 mM EDTA, 1 mM EGTA, 10 mM Tris–HCl, pH 7.4, with a complete protease inhibitor cocktail; Roche). The homogenate (H fraction) was centrifuged at 1000 × g for 10 min to precipitate the membrane fragments and nuclei (P1 fraction). Supernatant was centrifuged again at 1000 × g for 10 min, and the resulting supernatant (S1) was centrifuged at 12,000 × g for 20 min. Supernatant was the S2 fraction, and the pellet was resuspended with buffer A and centrifuged at 12,000 × g for 20 min. The resulting pellet was crude synaptosomes (P2 fraction), containing synaptosomes with mitochondria and microsomes.
Crude synaptosomes (P2 fraction) was resuspended with 150–200 μl buffer B (320 mM sucrose and 10 mM Tris–HCI [pH 7.4]). The sample was carefully overlaid on the top of a gradient of 2 ml of 7.5% (wt/vol in buffer B) Ficoll and 1.8 ml of 13% (wt/vol in buffer B) Ficoll and centrifuged at 98,000 × g for 45 min at 2–4°C in a swinging-bucket rotor. A myelin band was present near the surface, and the synaptosomes band (fraction Sy) was present at the interface between the 13 and 7.5% Ficoll layers, with the mitochondria being pelleted at the bottom. For further western analysis, the supernatant was divided into six fractions (600 μl for each fraction) and the mitochondria pellet was discarded. The isolated synaptosomes was included in fraction 4.
For western analysis, fractions H, S1, P1, S2, P2, and Sy were adjusted to 0.5 mg/ml by bicinchoninic acid assay (BCA) method with reference to NanoDrop 2000 Spectrophotometers. 3.35 μg protein was loaded for each lane. Fractions 1–6 were loaded with the same volume (10 μl composed of 6.7 μl sample and 3.3 μl loading buffer) for each lane.
Creatine uptake into synaptosomes
Request a detailed protocolTo remove Ficoll, we diluted the synaptosomal band (480 μl) with 4.3 ml of a pH 7.4 buffer C containing (in mM) 240 mannitol, 10 glucose, 4.8 potassium gluconate, 2.2 calcium gluconate, 1.2 MgSO4, 1.2 KH2PO4, and 25 HEPES-Tris. The sample was then centrifuged at 12,000 × g, and the pellet was resuspended with buffer C. Uptake experiments were either performed at 37°C or at 0°C (control). For each sample, 25–43 μg of synaptosomes (with a volume of 40–50 μl) were added to 360 μl buffer containing (in mmol/l) 100 NaCl, 40 mannitol, 10 glucose, 4.8 potassium gluconate, 2.2 calcium gluconate, 1.2 MgSO4, 1.2 KH2PO4, 25 HEPES, and 25 Tris (pH adjusted to 7.4). A mixture of 18 μM [14C]-creatine (0.4 μCi) and 5 μM creatine was quickly added. After 10 min, uptake was terminated by the addition of 1 ml of NaCl-free ice-cold buffer C. Samples were immediately filtered, under vacuum, through a Whatman GF/C glass filter (1825-025) pre-wetted with buffer C. Filters were further washed with 10 ml of ice-cold buffer C, dissolved in scintillation fluid, and the radioactivity determined by liquid scintillation spectrometry.
Creatine uptake into SVs
Request a detailed protocolThe uptake of 13C-creatine was assayed according to a conventional procedure (Hell et al., 1988) with slight modifications: the immunoisolated SVs by 10 μg Syp antibody (101011, SySy) were resuspended with the uptake buffer (150 mM meglumine-tartrate, 4 mM KCl, 4 mM MgSO4, 10 mM HEPES-KOH [pH 7.4], and cOmplete EDTA-free protease inhibitor cocktail) containing 4 mM Mg-ATP or additional 4 mM MgSO4, followed by preincubation for 30 min at 25℃. The uptake reaction was started by addition of 1 mM 13C-creatine dissolved in the uptake buffer with a final volume of 125 μl (pH at 6.8). After 10 min at 25℃, 1 ml of ice-cold uptake buffer was added to the incubation to stop the reaction, followed by five more times washing. The SV contents were extracted using the protocol described in the determination of vesicular contents part. Then, 100 nM Cr was used as the internal control. CE-MS and LC-MS were used to verify and quantify the creatine contents of samples. A Vanquish UHPLC system coupled to a Q Exactive HF-X mass spectrometer (both instrument from Thermo Fisher Scientific) was used for LC-MS analysis along with SeQuant ZIC-HILIC column (150 mm × 2.1 mm, 3.5 μm, Merck Millipore, 150442) in the positive mode and SeQuant ZIC-pHILIC column (150 mm × 2.1 mm, 5 μm, Merck Millipore, 150460) in the negative mode. For ZIC-HILIC column, the mobile phase A was 0.1% formic acid in water and the mobile phase B was 0.1% formic acid in acetonitrile. The linear gradient was as follows: 0 min, 80% B; 6 min, 50% B; 13 min, 50% B; 14 min, 20% B; 18 min, 20% B; 18.5 min, 80% B; and 30 min, 80% B. The flow rate used was 300 μl/min and the column temperature was maintained at 30°C. For ZIC-pHILIC column, the mobile phase A is 20 mM ammonium carbonate in water, adjusted to pH 9.0 with 0.1% ammonium hydroxide solution (25%), and the mobile phase B is 100% acetonitrile. The linear gradient was as follows: 0 min, 80% B; 2 min, 80% B; 19 min, 20% B; 20 min, 80% B; and 30 min, 80% B. The flow rate used was 150 μl/min, and the column temperature was 25°C. Samples were maintained at 4°C in Vanquish autosampler. Then, 3 µl of extracted metabolites were injected for each run. IP samples were subjected to ZIC-HILIC column in positive mode for major metabolites detection, and then subject to ZIC-pHILIC column in negative mode for orthogonal detection.
Data availability
All data generated or analyzed during this study are included in the manuscript and supporting file.
References
-
Deletion of the creatine transporter (Slc6a8) in dopaminergic neurons leads to hyperactivity in miceJournal of Molecular Neuroscience 70:102–111.https://doi.org/10.1007/s12031-019-01405-w
-
The possible role of hydrogen sulfide as an endogenous neuromodulatorThe Journal of Neuroscience 16:1066–1071.https://doi.org/10.1523/JNEUROSCI.16-03-01066.1996
-
On the blood pressure raising constituent of the suprarenal capsuleJohns Hopkins Hospital Bulletin 8:151–157.
-
On epinephrin, the active constituent of the suprarenal capsule and its compoundsProceedings of the American Physiological Society 3:4–5.
-
Ueber den blutdruckerregenden Bestandtheil der Nebenniere, das EpinephrinHoppe-Seyler´s Zeitschrift Für Physiologische Chemie 28:318–362.https://doi.org/10.1515/bchm2.1899.28.3-4.318
-
BookOn the Constitutional and Local Effects of Disease of the Supra-Renal CapsulesHighley.
-
Small-scale isolation of synaptic vesicles from mammalian brainNature Protocols 8:998–1009.https://doi.org/10.1038/nprot.2013.053
-
A preliminary report on the active principle of the suprarenal glandAmerican Journal of Physiology-Legacy Content 5:457–461.https://doi.org/10.1152/ajplegacy.1901.5.7.457
-
SNAP-25, a known presynaptic protein with emerging postsynaptic functionsFrontiers in Synaptic Neuroscience 8:7.https://doi.org/10.3389/fnsyn.2016.00007
-
The physiological disposition of H3-epinephrine and its metabolite metanephrineThe Journal of Pharmacology and Experimental Therapeutics 127:251–256.
-
Is brain estradiol a hormone or a neurotransmitter?Trends in Neurosciences 29:241–249.https://doi.org/10.1016/j.tins.2006.03.004
-
Atypical neural messengersTrends in Neurosciences 24:99–106.https://doi.org/10.1016/S0166-2236(00)01716-1
-
Chemical structure and sympathomimetic action of aminesThe Journal of Physiology 41:19–59.https://doi.org/10.1113/jphysiol.1910.sp001392
-
Language disorder with mild intellectual disability in a child affected by a novel mutation of SLC6A8 geneMolecular Genetics and Metabolism 102:153–156.https://doi.org/10.1016/j.ymgme.2010.11.005
-
Reversible brain creatine deficiency in two sisters with normal blood creatine levelAnnals of Neurology 47:511–513.
-
A method for differentiating dopamine from noradrenaline in tissue sections by microspectrofluorometryThe Journal of Histochemistry and Cytochemistry 16:263–270.https://doi.org/10.1177/16.4.263
-
A rapid method for the preparation of relatively pure metabolically competent synaptosomes from rat brainThe Biochemical Journal 176:365–370.https://doi.org/10.1042/bj1760365
-
Molecular heterogeneity of the gamma-aminobutyric acid (GABA) transport system. Cloning of two novel high affinity GABA transporters from rat brainThe Journal of Biological Chemistry 267:21098–21104.
-
Rapid and Gentle immunopurification of brain synaptic vesiclesThe Journal of Neuroscience 42:3512–3522.https://doi.org/10.1523/JNEUROSCI.2521-21.2022
-
Endogenous synthesis and transport of creatine in the rat brain: an in situ hybridization studyBrain Research. Molecular Brain Research 86:193–201.https://doi.org/10.1016/s0169-328x(00)00269-2
-
Expression and function of AGAT, GAMT and CT1 in the mammalian brainSub-Cellular Biochemistry 46:67–81.https://doi.org/10.1007/978-1-4020-6486-9_4
-
AGAT, GAMT and SLC6A8 distribution in the central nervous system, in relation to creatine deficiency syndromes: A reviewJournal of Inherited Metabolic Disease 31:230–239.https://doi.org/10.1007/s10545-008-0826-9
-
Dissociation of AGAT, GAMT and SLC6A8 in CNS: relevance to creatine deficiency syndromesNeurobiology of Disease 37:423–433.https://doi.org/10.1016/j.nbd.2009.10.022
-
Creatine and guanidinoacetate transport at blood-brain and blood-cerebrospinal fluid barriersJournal of Inherited Metabolic Disease 35:655–664.https://doi.org/10.1007/s10545-011-9433-2
-
The SLC6 orphans are forming a family of amino acid transportersNeurochemistry International 48:559–567.https://doi.org/10.1016/j.neuint.2005.11.021
-
The solute carrier 6 family of transportersBritish Journal of Pharmacology 167:256–278.https://doi.org/10.1111/j.1476-5381.2012.01975.x
-
Creatine: endogenous metabolite, dietary, and therapeutic supplementAnnual Review of Nutrition 27:241–261.https://doi.org/10.1146/annurev.nutr.27.061406.093621
-
The acetyloholine metabolism of a sympathetic ganglionThe Journal of Physiology 88:265–283.https://doi.org/10.1113/jphysiol.1936.sp003439
-
Depolarization of neurones in the isolated olfactory cortex of the guinea-pig by gamma-aminobutyric acidBritish Journal of Pharmacology 65:339–345.https://doi.org/10.1111/j.1476-5381.1979.tb07835.x
-
Neurons and astroglia express distinct subsets of Na,K-ATPase α and β subunitsMolecular Brain Research 21:333–343.https://doi.org/10.1016/0169-328X(94)90264-X
-
Studies on conditions of activity in endocrine organsAmerican Journal of Physiology-Legacy Content 104:557–574.https://doi.org/10.1152/ajplegacy.1933.104.3.557
-
Cellular localization of brain monoaminesActa Physiologica Scandinavica 56:1–28.https://doi.org/10.1111/j.1748-1716.1962.tb02561.x
-
Screening for primary creatine deficiencies in French patients with unexplained neurological symptomsOrphanet Journal of Rare Diseases 7:96.https://doi.org/10.1186/1750-1172-7-96
-
Characterization of a H+-ATPase in rat brain synaptic vesiclesJournal of Biological Chemistry 264:8281–8288.https://doi.org/10.1016/S0021-9258(18)83180-5
-
The central release of acetylcholine during stimulation of the visual pathwayThe Journal of Physiology 184:239–254.https://doi.org/10.1113/jphysiol.1966.sp007913
-
The central release of acetylcholine during consciousness and after brain lesionsThe Journal of Physiology 188:83–98.https://doi.org/10.1113/jphysiol.1967.sp008125
-
BookThe Biochemical Basis of NeuropharmacologyOxford University Press.https://doi.org/10.1093/oso/9780195140071.001.0001
-
The excitation and depression of spinal neurones by structurally related amino acidsJournal of Neurochemistry 6:117–141.https://doi.org/10.1111/j.1471-4159.1960.tb13458.x
-
Acidic amino acids with strong excitatory actions on mammalian neuronesThe Journal of Physiology 166:1–14.https://doi.org/10.1113/jphysiol.1963.sp007087
-
The action of certain esters and ethers of choline, and their relation to muscarineJournal of Pharmacology and Experimental Therapeutics 6:147–190.
-
The presence of histamine and acetylcholine in the spleen of the ox and the horseThe Journal of Physiology 68:97–123.https://doi.org/10.1113/jphysiol.1929.sp002598
-
Release of acetylcholine at voluntary motor nerve endingsThe Journal of Physiology 86:353–380.https://doi.org/10.1113/jphysiol.1936.sp003371
-
D‐Aspartic acid is a novel endogenous neurotransmitterThe FASEB Journal 25:1014–1027.https://doi.org/10.1096/fj.10-168492
-
Endogenous guanidino compounds as uremic neurotoxinsKidney International 59:S77–S83.https://doi.org/10.1046/j.1523-1755.2001.59780077.x
-
Quantal components of the end-plate potentialThe Journal of Physiology 124:560–573.https://doi.org/10.1113/jphysiol.1954.sp005129
-
Biophysical aspects of neuro-muscular transmissionProgress in Biophysics and Biophysical Chemistry 6:121–170.https://doi.org/10.1016/S0096-4174(18)30106-9
-
Some features of the submicroscopic morphology of synapses in frog and earthwormThe Journal of Biophysical and Biochemical Cytology 1:47–58.https://doi.org/10.1083/jcb.1.1.47
-
Treatment of X-linked creatine transporter (SLC6A8) deficiency: systematic review of the literature and three new casesMolecular Genetics and Metabolism 112:259–274.https://doi.org/10.1016/j.ymgme.2014.05.011
-
Monitoring of vacuolar-type H+ ATPase-mediated proton influx into synaptic vesiclesThe Journal of Neuroscience 35:3701–3710.https://doi.org/10.1523/JNEUROSCI.4160-14.2015
-
Projections of the somatosensory areas of the cerebral cortex into the thalamic nucleiBiulleten’ Eksperimental’noi Biologii i Meditsiny 80:113–117.
-
On the action of adrenalinThe Journal of Physiology 31:xx–xxi.https://doi.org/10.1113/jphysiol.1904.sp001055
-
The action of adrenalinThe Journal of Physiology 32:401–467.https://doi.org/10.1113/jphysiol.1905.sp001093
-
Acetylcholine, a new active principle of ErgotThe Biochemical Journal 8:44–49.https://doi.org/10.1042/bj0080044
-
Spontaneous subthreshold activity at motor nerve endingsThe Journal of Physiology 117:109–128.
-
Regulation of Cre recombinase activity by mutated estrogen receptor ligand-binding domainsBiochemical and Biophysical Research Communications 237:752–757.https://doi.org/10.1006/bbrc.1997.7124
-
The chemical transmitter at synapses in a sympathetic ganglionThe Journal of Physiology 81:305–319.https://doi.org/10.1113/jphysiol.1934.sp003137
-
An inhibitory and an excitatory factor of mammalian central nervous system, and their action on a single sensory neuronArchives Internationales de Physiologie 62:33–53.https://doi.org/10.3109/13813455409145367
-
Creatine defects and central nervous systemSeminars in Pediatric Neurology 23:285–289.https://doi.org/10.1016/j.spen.2016.11.003
-
Uptake of γ‐Aminobutyric acid by a synaptic vesicle fraction isolated from rat brainJournal of Neurochemistry 50:1237–1242.https://doi.org/10.1111/j.1471-4159.1988.tb10599.x
-
Delayed creatine supplementation counteracts reduction of GABAergic function and protects against seizures susceptibility after traumatic brain injury in ratsProgress in Neuro-Psychopharmacology and Biological Psychiatry 92:328–338.https://doi.org/10.1016/j.pnpbp.2019.02.004
-
“Choline/orphan V8-2-1/creatine transporter” mRNA is expressed in nervous, renal and gastrointestinal systemsBrain Research. Molecular Brain Research 23:266–270.https://doi.org/10.1016/0169-328x(94)90233-x
-
Role of the afterhyperpolarization in control of discharge properties of septal cholinergic neurons in vitroJournal of Neurophysiology 75:695–706.https://doi.org/10.1152/jn.1996.75.2.695
-
Molecular cloning of a novel 97-kd Golgi complex autoantigen associated with Sjögren’s syndromeArthritis and Rheumatism 40:1693–1702.https://doi.org/10.1002/art.1780400920
-
A Na(+)-dependent creatine transporter in rabbit brain, muscle, heart, and kidney. cDNA cloning and functional expressionThe Journal of Biological Chemistry 268:8418–8421.
-
Cloning and sequencing of rat kidney L-arginine:glycine amidinotransferase. Studies on the mechanism of regulation by growth hormone and creatineJournal of Biological Chemistry 269:17556–17560.https://doi.org/10.1016/S0021-9258(17)32477-8
-
In situ hybridization analysis of CHOT1, a creatine transporter, in the rat central nervous systemThe Journal of Comparative Neurology 351:94–103.https://doi.org/10.1002/cne.903510109
-
Cellular scaling rules for rodent brainsPNAS 103:12138–12143.https://doi.org/10.1073/pnas.0604911103
-
The repertoire of solute carriers of family 6: identification of new human and rodent genesBiochemical and Biophysical Research Communications 336:175–189.https://doi.org/10.1016/j.bbrc.2005.08.048
-
Note on a blood pressure lowering body in the suprarenal glandThe American Journal of Physiology 3:xviii–xix.
-
On the physiological action of certain cholin derivatives and new methods for detecting cholinBritish Medical Journal pp. 1788–1789.
-
Arginine:glycine amidinotransferase deficiency: the third inborn error of creatine metabolism in humansAmerican Journal of Human Genetics 69:1127–1133.https://doi.org/10.1086/323765
-
Neurotransmitter transporters and their impact on the development of psychopharmacologyBritish Journal of Pharmacology 147 Suppl 1:S82–S88.https://doi.org/10.1038/sj.bjp.0706428
-
Synaptic vesicles and exocytosisAnnual Review of Neuroscience 17:219–246.https://doi.org/10.1146/annurev.ne.17.030194.001251
-
Epitope tagging and protein surveillanceMethods in Enzymology 194:508–519.https://doi.org/10.1016/0076-6879(91)94038-e
-
SNAP23 deficiency causes severe brain dysplasia through the loss of radial glial cell polarityThe Journal of Cell Biology 220:e201910080.https://doi.org/10.1083/jcb.201910080
-
Cyclocreatine treatment improves cognition in mice with creatine transporter deficiencyThe Journal of Clinical Investigation 122:2837–2846.https://doi.org/10.1172/JCI59373
-
III. On the local paralysis of peripheral ganglia, and on the connexion of different classes of nerve fibres with themProceedings of the Royal Society of London 46:423–431.https://doi.org/10.1098/rspl.1889.0051
-
Observations on the physiological action of extracts of the supra-renal bodiesThe Journal of Physiology 27:237–256.https://doi.org/10.1113/jphysiol.1901.sp000869
-
On the stimulation and paralysis of nerve‐cells and of nerve‐endingsThe Journal of Physiology 27:224–236.https://doi.org/10.1113/jphysiol.1901.sp000868
-
Croonian Lecture, 1906.—On nerve endings and on special excitable substances in cellsProceedings of the Royal Society of London. Series B, Containing Papers of a Biological Character 78:170–194.https://doi.org/10.1098/rspb.1906.0056
-
Kreatin und Kreatinin, Bestandtheile des Harns der MenschenJournal Für Praktische Chemie 40:288–292.https://doi.org/10.1002/prac.18470400170
-
Ueber die chemische Beschaffenheit der GehirnsubstanzJustus Liebigs Annalen Der Chemie 134:29–44.https://doi.org/10.1002/jlac.18651340107
-
Tetanus toxin action: Inhibition of neurotransmitter release linked to synaptobrevin proteolysisBiochemical and Biophysical Research Communications 189:1017–1023.https://doi.org/10.1016/0006-291X(92)92305-H
-
Über humorale übertragbarkeit der HerznervenwirkungPflügers Archiv Für Die Gesamte Physiologie Des Menschen Und Der Tiere 189:239–242.https://doi.org/10.1007/BF01738910
-
Expression of a mouse brain cDNA encoding novel gamma-aminobutyric acid transporterThe Journal of Biological Chemistry 267:17491–17493.
-
Distribution of the creatine transporter throughout the human brain reveals a spectrum of creatine transporter immunoreactivityThe Journal of Comparative Neurology 523:699–725.https://doi.org/10.1002/cne.23667
-
Live imaging of synaptic vesicle release and retrieval in dopaminergic neuronsFrontiers in Neural Circuits 3:3.https://doi.org/10.3389/neuro.04.003.2009
-
Storage and uptake of D-serine into astrocytic synaptic-like vesicles specify gliotransmissionThe Journal of Neuroscience 33:3413–3423.https://doi.org/10.1523/JNEUROSCI.3497-12.2013
-
Clathrin-coated vesicles in nervous tissue are involved primarily in synaptic vesicle recyclingThe Journal of Cell Biology 118:1379–1388.https://doi.org/10.1083/jcb.118.6.1379
-
In vitro release of endogenous monoamines and amino acids from several CNS regions of the ratNeurochemical Research 8:245–257.https://doi.org/10.1007/BF00963924
-
Treatment of intractable epilepsy in a female with SLC6A8 deficiencyMolecular Genetics and Metabolism 101:409–412.https://doi.org/10.1016/j.ymgme.2010.08.016
-
Isolation of plasma membranes from neurons grown in primary cultureAnalytical Biochemistry 166:246–252.https://doi.org/10.1016/0003-2697(87)90571-9
-
Is DOPA a neurotransmitter?Trends in Pharmacological Sciences 23:262–268.https://doi.org/10.1016/s0165-6147(02)02013-8
-
The spontaneous and evoked release of acetylcholine from the cerebral cortexThe Journal of Physiology 165:98–116.https://doi.org/10.1113/jphysiol.1963.sp007045
-
Mechanisms of enhanced taurine release under Ca2+ depletionNeurochemistry International 47:343–349.https://doi.org/10.1016/j.neuint.2005.04.027
-
On the physiological action of choline and neurineBritish Medical Journal 1:1082–1083.https://doi.org/10.1136/bmj.1.2001.1082
-
Characterization of putative amino acid transmitter release from slices of rat dentate gyrusJournal of Neurochemistry 29:279–290.https://doi.org/10.1111/j.1471-4159.1977.tb09620.x
-
Characterization of a cis-Golgi matrix protein, GM130The Journal of Cell Biology 131:1715–1726.https://doi.org/10.1083/jcb.131.6.1715
-
Cloning, pharmacological characterization, and genomic localization of the human creatine transporterReceptors & Channels 2:165–174.
-
The family of Na+/Cl- neurotransmitter transportersJournal of Neurochemistry 71:1785–1803.https://doi.org/10.1046/j.1471-4159.1998.71051785.x
-
Activation of GABAA receptors by guanidinoacetate: A novel pathophysiological mechanismNeurobiology of Disease 11:298–307.https://doi.org/10.1006/nbdi.2002.0547
-
Incidence of brain creatine transporter deficiency in males with developmental delay referred for brain magnetic resonance imagingJournal of Developmental and Behavioral Pediatrics 26:276–282.https://doi.org/10.1097/00004703-200508000-00003
-
The blood-brain barrier creatine transporter is a major pathway for supplying creatine to the brainJournal of Cerebral Blood Flow and Metabolism 22:1327–1335.https://doi.org/10.1097/01.WCB.0000033966.83623.7D
-
5-HT1A receptor linked to inward-rectifying potassium current in hippocampal CA3 pyramidal cellsJournal of Neurophysiology 71:2161–2167.https://doi.org/10.1152/jn.1994.71.6.2161
-
Ratio of pyramidal cells versus non-pyramidal cells in sector CA1 of the human Ammon’s hornAnatomy and Embryology 173:105–110.https://doi.org/10.1007/BF00707308
-
The physiological effects of extracts of the suprarenal capsulesThe Journal of Physiology 18:230–276.https://doi.org/10.1113/jphysiol.1895.sp000564
-
Electron microscope observations of interneuronal and neuromuscular synapsesThe Anatomical Record 118:335–336.
-
BookPaxinos and Franklin’s the Mouse Brain in Stereotaxic CoordinatesElsevier/Academic Press.
-
K+-evoked taurine efflux from cerebellar astrocytes: on the roles of Ca2+ and Na+Neurochemical Research 14:43–48.https://doi.org/10.1007/BF00969756
-
The screening of SLC6A8 deficiency among Estonian families with X-linked mental retardationJournal of Inherited Metabolic Disease 33 Suppl 3:S5–S11.https://doi.org/10.1007/s10545-008-1063-y
-
Reconstitution and purification of the sodium- and chloride-coupled gamma-aminobutyric acid transporter from rat brainThe Journal of Biological Chemistry 260:11859–11865.
-
Purification and identification of the functional sodium- and chloride-coupled gamma-aminobutyric acid transport glycoprotein from rat brainThe Journal of Biological Chemistry 261:15437–15441.
-
Oral creatine monohydrate supplementation improves brain performance: a double-blind, placebo-controlled, cross-over trialProceedings. Biological Sciences 270:2147–2150.https://doi.org/10.1098/rspb.2003.2492
-
Creatine as a booster for human brain function. How might it work?Neurochemistry International 89:249–259.https://doi.org/10.1016/j.neuint.2015.08.010
-
The first hormone: AdrenalineTrends in Endocrinology and Metabolism 30:331–334.https://doi.org/10.1016/j.tem.2019.03.005
-
Identification of a novel syntaxin- and synaptobrevin/VAMP-binding protein, SNAP-23, expressed in non-neuronal tissuesJournal of Biological Chemistry 271:13300–13303.https://doi.org/10.1074/jbc.271.23.13300
-
Is agmatine a novel neurotransmitter in brain?Trends in Pharmacological Sciences 21:187–193.https://doi.org/10.1016/s0165-6147(00)01460-7
-
The effects of amino acids on the crustacean neuro-muscular systemThe Anatomical Record 132:492–493.
-
gamma-Aminobutyric acid in brain: its formation from glutamic acidThe Journal of Biological Chemistry 187:55–63.
-
Ultrastructure of two invertebrate synapsesProceedings of the Society for Experimental Biology and Medicine 82:219–223.https://doi.org/10.3181/00379727-82-20071
-
BookMechanisms of Synaptic Transmission. Bridging the GapsOxford University Press.https://doi.org/10.1093/acprof:oso/9780195137613.001.0001
-
High prevalence of SLC6A8 deficiency in X-linked mental retardationThe American Journal of Human Genetics 75:97–105.https://doi.org/10.1086/422102
-
Cerebral creatine deficiency affects the timing of oligodendrocyte myelinationThe Journal of Neuroscience 43:1143–1153.https://doi.org/10.1523/JNEUROSCI.2120-21.2022
-
Cleavage of foot-and-mouth disease virus polyprotein is mediated by residues located within a 19 amino acid sequenceThe Journal of General Virology 72 (Pt 11):2727–2732.https://doi.org/10.1099/0022-1317-72-11-2727
-
X-linked creatine-transporter gene (SLC6A8) defect: a new creatine-deficiency syndromeThe American Journal of Human Genetics 68:1497–1500.https://doi.org/10.1086/320595
-
Expression of the rat brain creatine transporter in situ and in transfected HeLa cellsDevelopmental Neuroscience 18:524–534.https://doi.org/10.1159/000111450
-
BookTaurine in neurotransmissionIn: Lajtha A, Vizi ES, editors. Handbook of Neurochemistry and Molecular Neurobiology. Springer. pp. 325–334.https://doi.org/10.1007/978-0-387-30382-6_13
-
Cre-stimulated recombination at loxP-containing DNA sequences placed into the mammalian genomeNucleic Acids Research 17:147–161.https://doi.org/10.1093/nar/17.1.147
-
On the present condition of our knowledge regarding the functions of the suprarenal capsulesBritish Medical Journal 1:1277–1281.https://doi.org/10.1136/bmj.1.2474.1277
-
The putative rat choline transporter CHOT1 transports creatine and is highly expressed in neural and muscle-rich tissuesBiochemical and Biophysical Research Communications 198:637–645.https://doi.org/10.1006/bbrc.1994.1093
-
The cloning and expression of a human creatine transporterBiochemical and Biophysical Research Communications 204:419–427.https://doi.org/10.1006/bbrc.1994.2475
-
Creatine transporters: a reappraisalMolecular and Cellular Biochemistry 256–257:407–424.https://doi.org/10.1023/b:mcbi.0000009886.98508.e7
-
Arginine:glycine amidinotransferase (AGAT) deficiency: Clinical features and long term outcomes in 16 patients diagnosed worldwideMolecular Genetics and Metabolism 116:252–259.https://doi.org/10.1016/j.ymgme.2015.10.003
-
Ueber einige neue Bestandtheile der SchweinegalleJustus Liebigs Annalen Der Chemie 123:353–360.https://doi.org/10.1002/jlac.18621230310
-
A neuronal role for SNAP-23 in postsynaptic glutamate receptor traffickingNature Neuroscience 13:338–343.https://doi.org/10.1038/nn.2488
-
Adrenalin, the active principle of the suprarenal glands and its mode of preparationAmerican Journal of Pharmacy 73:523–531.
-
The isolation of the active principle of the suprarenal glandThe Journal of Physiology 27:xxix–xxx.https://doi.org/10.1113/jphysiol.1902.sp000893
-
Ca2+ depletion facilitates taurine release in cultured rat astrocytesJapanese Journal of Pharmacology 72:75–78.https://doi.org/10.1254/jjp.72.75
-
Beta-alanine as a small molecule neurotransmitterNeurochemistry International 57:177–188.https://doi.org/10.1016/j.neuint.2010.06.001
-
Identification of gamma-aminobutyric acid in brain by the isotope derivative methodThe Journal of Biological Chemistry 187:65–69.
-
X-linked creatine transporter deficiency: clinical aspects and pathophysiologyJournal of Inherited Metabolic Disease 37:715–733.https://doi.org/10.1007/s10545-014-9713-8
-
BookNoradrenaline. Chemistry, Physiology, Pharmacology and Clinical AspectsSpringfield.
-
Creatine: a miserable life without itAmino Acids 48:1739–1750.https://doi.org/10.1007/s00726-016-2297-x
-
Effects of creatine on mental fatigue and cerebral hemoglobin oxygenationNeuroscience Research 42:279–285.https://doi.org/10.1016/s0168-0102(02)00007-x
-
The separation of synaptic vesicles from nerve-ending particles (‘synaptosomes’)Biochemical Journal 90:293–303.https://doi.org/10.1042/bj0900293
-
Creatine and creatinine metabolismPhysiological Reviews 80:1107–1213.https://doi.org/10.1152/physrev.2000.80.3.1107
Article and author information
Author details
Funding
Chinese Academy of Medical Sciences (2019RU003)
- Yi Rao
Chinese Institute for Brain Research
- Yi Rao
Peking-Tsinghua Center for Life Sciences
- Yi Rao
Changping Laboratory
- Yi Rao
National Natural Science Foundation of China (32061143017)
- Yi Rao
National Natural Science Foundation of China (81473189)
- Xiling Bian
The funders had no role in study design, data collection and interpretation, or the decision to submit the work for publication.
Acknowledgements
We are grateful to Drs. Xiaohui Zhang, Xinxiang Zhang, Minmin Luo, Qingchun Guo, Yiqun Liu, Yingchun Hu, and Wuping Ge for discussion, suggestions, and technical support; Jiang Chen, Jing Cai, Yulin Jiang, Jinhuan Ou, Xiaomeng Deng, Jiawen Liu, and Meng Wu for technical assistance; and CIBR, Peking-Tsinghua Center for Life Sciences, Changping Laboratory, Chinese Academy of Medical Sciences (2019RU003), and National Natural Science Foundation of China (Project 32061143017 to YR and 81473189) for support. Research in the Rao lab has never been contaminated by the Chinese Brain Initiative.
Ethics
All animal procedures were approved by the Animal Center of Peking University and Animal Care. Experiments were carried out in accordance with the guidelines of Institutional Animal Care and Use Committee (IACUC) of Peking University (LSC-RaoY-9 and LSC-RaoY-10).
Version history
- Preprint posted:
- Sent for peer review:
- Reviewed Preprint version 1:
- Reviewed Preprint version 2:
- Reviewed Preprint version 3:
- Version of Record published:
Cite all versions
You can cite all versions using the DOI https://doi.org/10.7554/eLife.89317. This DOI represents all versions, and will always resolve to the latest one.
Copyright
© 2023, Bian, Zhu, Jia et al.
This article is distributed under the terms of the Creative Commons Attribution License, which permits unrestricted use and redistribution provided that the original author and source are credited.
Metrics
-
- 7,412
- views
-
- 607
- downloads
-
- 14
- citations
Views, downloads and citations are aggregated across all versions of this paper published by eLife.
Citations by DOI
-
- 11
- citations for umbrella DOI https://doi.org/10.7554/eLife.89317
-
- 3
- citations for Reviewed Preprint v1 https://doi.org/10.7554/eLife.89317.1