Mobile barrier mechanisms for Na+-coupled symport in an MFS sugar transporter
eLife assessment
In an important study that will be of interest to the mechanistic membrane transport community, the authors capture the first cryoEM structure of the inward-facing melibiose transporter MelB, a well-studied model transporter from the major facilitator (MFS) superfamily. CryoEM experiments and supporting biophysical experiments provide solid evidence for transporter conformational changes.
https://doi.org/10.7554/eLife.92462.3.sa0Important: Findings that have theoretical or practical implications beyond a single subfield
- Landmark
- Fundamental
- Important
- Valuable
- Useful
Solid: Methods, data and analyses broadly support the claims with only minor weaknesses
- Exceptional
- Compelling
- Convincing
- Solid
- Incomplete
- Inadequate
During the peer-review process the editor and reviewers write an eLife Assessment that summarises the significance of the findings reported in the article (on a scale ranging from landmark to useful) and the strength of the evidence (on a scale ranging from exceptional to inadequate). Learn more about eLife Assessments
Abstract
While many 3D structures of cation-coupled transporters have been determined, the mechanistic details governing the obligatory coupling and functional regulations still remain elusive. The bacterial melibiose transporter (MelB) is a prototype of major facilitator superfamily transporters. With a conformation-selective nanobody, we determined a low-sugar affinity inward-facing Na+-bound cryoEM structure. The available outward-facing sugar-bound structures showed that the N- and C-terminal residues of the inner barrier contribute to the sugar selectivity. The inward-open conformation shows that the sugar selectivity pocket is also broken when the inner barrier is broken. Isothermal titration calorimetry measurements revealed that this inward-facing conformation trapped by this nanobody exhibited a greatly decreased sugar-binding affinity, suggesting the mechanisms for substrate intracellular release and accumulation. While the inner/outer barrier shift directly regulates the sugar-binding affinity, it has little or no effect on the cation binding, which is supported by molecular dynamics simulations. Furthermore, the hydron/deuterium exchange mass spectrometry analyses allowed us to identify dynamic regions; some regions are involved in the functionally important inner barrier-specific salt-bridge network, which indicates their critical roles in the barrier switching mechanisms for transport. These complementary results provided structural and dynamic insights into the mobile barrier mechanism for cation-coupled symport.
Introduction
Cation-coupled transporters play critical roles in many aspects of cell physiology and pharmacokinetics and are involved in many serious diseases, including cancers, metabolic diseases, neurodegenerative diseases, etc. Their specific locations and functions make them viable drug targets (César-Razquin et al., 2015; Lin et al., 2015). Following decades of effort, the structures of many transporters have been determined by x-ray crystallography and, more recently, by cryoEM (Abramson et al., 2003; Cherezov et al., 2007; Kumar et al., 2014; Huang et al., 2003; Boudker et al., 2007; Yamashita et al., 2005; Gouaux, 2009; Pedersen et al., 2013; Ethayathulla et al., 2014; Yan, 2015; Drew et al., 2021; Guan, 2022). However, we still do not have a deep understanding of the structural basis for substrate accumulation to high concentrations, the mechanisms for the obligatorily coupled transport, as well as the cellular regulation of transporters’ activities to meet metabolic needs.
Salmonella enterica serovar Typhimurium melibiose permease (MelBSt) is the prototype Na+/solute transporter of the major facilitator superfamilies (MFS) (Ethayathulla et al., 2014; Guan, 2018; Markham et al., 2021; Hariharan and Guan, 2017; Hariharan et al., 2018; Guan and Hariharan, 2021; Blaimschein et al., 2023; Granell et al., 2010; Ganea et al., 2001; Chae et al., 2010; Panda et al., 2023). It catalyzes the stoichiometric symport of galactopyranoside with Na+, H+, or Li+ (Guan et al., 2011). Crystal structures of sugar-bound states have been determined, which reveals the sugar recognition mechanism (Guan and Hariharan, 2021). In all solved x-ray crystal structures, MelBSt is in an outward-facing conformation; the observed physical barrier of the sugar translocation, named the inner barrier, prevents the substrate from moving into the cytoplasm directly. It is well-known that transporters change their conformation to allow the substrate translocation from one side of the membrane to the other and conformational dynamics is the intrinsic feature necessary for their functions (Abramson et al., 2003; Huang et al., 2003; Boudker et al., 2007; Yan, 2015; Drew et al., 2021; Guan and Kaback, 2006; Khare et al., 2009).
To understand how the sugar substrate and the coupling cations dictate transporter function, we have established thermodynamic binding cycles in MelBSt using isothermal titration calorimetry (ITC) measurements and identified that the positive cooperativity of the binding of cation and melibiose as the core symport mechanism (Hariharan and Guan, 2017); furthermore, the binding of the second substrate changes the thermodynamic feature of heat capacity change from positive to negative, suggesting primary dehydration processes when both substrates concurrently occupy the transporter (Hariharan and Guan, 2021). While the structural origins have not yet been completely determined yet, they may be related to forming an occluded intermediate conformation, a necessary state for a global conformational transition.
To determine structurally unresolved states of MelBSt, nanobodies (Nbs) were raised against the wild-type MelBSt. One group of Nbs (Nb714, Nb725, and Nb733) has been identified to interact with the cytoplasmic side of MelBSt and their binding completely inhibits MelBSt transport activity (Katsube et al., 2023). MelBSt bound with Nb725 or Nb733 retained its binding to Na+, but its affinity for melibiose is inhibited. The MelBSt/Nb733 complex also retained the affinity to its physiological regulator dephosphorylated EIIAGlc of the glucose-specific phosphoenolpyruvate:phosphotransferase system (PTS). EIIAGlc, the central metabolite regulator in bacterial catabolic repression (Postma et al., 1993; Deutscher et al., 2014; Guan, 2021), greatly inhibited the galactoside binding to non-PTS permeases MelBSt (Hariharan and Guan, 2021) and the H+-coupled lactose permease (LacY) (Hariharan et al., 2015), but still retained the binding of Na+ to MelBSt (Katsube et al., 2023). Thus, the functional modulation of the two Nbs on MelBSt mimics the binding of EIIAGlc. The crystal structure of dephosphorylated EIIAGlc binding to the maltose permease MalFGK2, an ATP-binding cassette transporter, has been determined (Chen et al., 2013), but there is no structural information on EIIAGlc binding to the MFS transporters. Determination of the structures of MelBSt in complex with this group of Nbs is important and will reveal critical information to understand the manner by which EIIAGlc regulates the large group of MFS sugar transporters.
In the current study, we applied cryoEM single-particle analysis (cryoEM-SPA) and determined the near-atomic resolution structure of MelBSt complexed with a Nb725_4, a modified Nb725 with a designed scaffold which enables a NabFab binding (Bloch et al., 2021). The use of the Nb provided a Na+-bound lower sugar-affinity inward-facing conformation of MelBSt. Furthermore, our studies using this conformation-selective Nb in combination with hydrogen/deuterium exchange mass spectrometry (HDX-MS) demonstrate a powerful approach for characterizing protein conformational flexibility (Masson et al., 2019; Zheng et al., 2019), and also provides critical dynamic information to understand how the substrate binding drives the conformational transition necessary for the substrate translocation in MelBSt.
Results
CDR grafting to generate a hybrid Nb and its functional characterization
To facilitate structure determination of MelBSt complexed with the conformation-selective Nb by cryoEM-SPA, a novel universal tool based on a NabFab was applied to increase the effective mass of small particles (Bloch et al., 2021) since MelBSt (mass, 53.5 kDa) has limited extramembrane mass. A hybrid Nb725_4 was created by complementarity-determining region (CDR) grafting or antibody reshaping (Riechmann et al., 1988) to transfer the binding specificity of MelBSt Nb725 to the TC-Nb4 recognized by the NabFab (Bloch et al., 2021; Figure 1—figure supplement 1). Extensive in vivo and in vitro functional characterization revealed that the hybrid Nb725_4 possesses the binding properties of its parents Nbs (Nb725 and TC-Nb41) with slightly compromised affinities to MelBSt (Katsube et al., 2023). Nb725_4 binds to MelBSt intracellularly and inhibits MelB-mediated melibiose fermentation and active transport, with an equilibrium dissociation constant (Kd) of 3.64 ± 0.62 µM, compared to 1.58 ± 0.42 µM for Nb725 (Figure 1a–d; Figure 1—source data 1; Table 1).
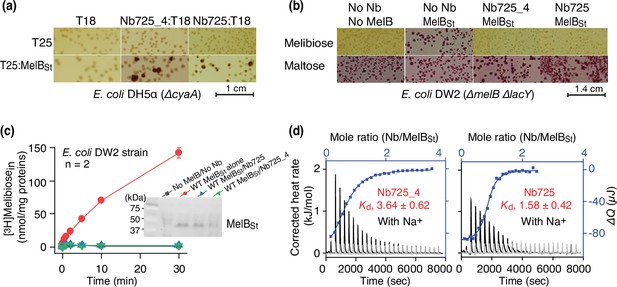
Functional characterizations of the hybrid Nb725_4.
(a) In vivo two-hybrid interaction assay. Two compatible plasmids encoding T25:MelBSt and Nb:T18 were transformed into E. coli DH5α cyaA cells and plated on the maltose-containing MacConkey agar plate as described in ‘Materials and methods’. The irregular red colonies are typical of a positive two-hybrid test indicating the protein-protein interactions. The image for the two hybrids T25:MelBSt and Nb725:T18 was reused from Figure 3a in Katsube et al., 2023 under a Creative Commons Attribution (CC BY 4.0) license. (b) Inhibition of melibiose fermentation by Nbs. Two compatible plasmids encoding MelBSt and Nb725 or Nb725_4 were transformed into E. coli DW2 cells [ΔmelBΔlacYZ] and plated on the MacConkey agar plate containing maltose (as the positive control) and melibiose (for testing transport activity of MelBSt) as the sole carbon source. The image of MelBSt/Nb725 was reused (Katsube et al., 2023). (c) [3H]Melibiose transport assay with E. coli DW2 cells. The cells transformed by two compatible plasmids encoding the MelBSt and Nb725 or Nb725_4 were prepared for [3H]melibiose transport assay at 0.4 mM (specific activity of 10 mCi/mmol) and 20 mM Na+ as described in ‘Materials and methods’. The cells transformed with the two empty plasmids without MelB or Nb were the negative control. Inset, western blot. MelBSt expression under the co-expression system was analyzed by isolating the membrane fractions. An aliquot of 50 μg was loaded on each well and MelBSt protein was probed by the HisProbe-HRP Conjugate. (d) Nb binding to MelBSt by isothermal titration calorimetry (ITC) measurements. As described in ‘Materials and methods’, the thermograms were collected with the Nano-ITC device (TA Instrument) at 25°C. Exothermic thermograms shown as positive peaks were obtained by titrating Nbs (0.3 mM) into the MelBSt-free buffer (gray) or MelBSt (35 μM)-containing buffer (black) in the Sample Cell and plotted using bottom/left (x/y) axes. The binding isotherm and fitting of the mole ratio (Nb/MelBSt) vs. the total heat change (ΔQ) using one-site independent-binding model were presented by top/right (x/y) axes. The dissociation constant Kd was presented at mean ± SEM (number of tests = 6–7).
-
Figure 1—source data 1
Original photographs that were taken from in vivo two-hybrid interaction assay and used to prepare Figure 1a.
- https://cdn.elifesciences.org/articles/92462/elife-92462-fig1-data1-v1.pdf
-
Figure 1—source data 2
Original photographs that were taken from sugar fermentation assay results and used to prepare Figure 1b.
- https://cdn.elifesciences.org/articles/92462/elife-92462-fig1-data2-v1.pdf
-
Figure 1—source data 3
Western blot to detect MelBSt expression when co-expressing with Nb725 or Nb725_4 (unlabelled).
Membranes were prepared from E. coli DW2 cells that were transformed with two compatible plasmids derived from pACYC and pCS19 encoding MelBSt and Nb725_4 or Nb725, respectively, and used for the [3H]melibiose active transport assay. After protein concentration determination, 50 μg of total membrane proteins of each sample was analyzed by SDS-15%PAGE and western blot using anti-His tag antibody (HisProbe-HRP Conjugate) as described in the Materials and methods. The western blot result was imaged by the ChemiDoc MP Imaging System (Bio-Rad). MelBSt protein expression presented as the inset in Figure 1c. The bands migrating near 25 kDa are non-specific and also presented in the membranes with no MelB nor Nb.
- https://cdn.elifesciences.org/articles/92462/elife-92462-fig1-data3-v1.jpg
-
Figure 1—source data 4
Western blot to detect MelBSt expression when co-expressing with Nb725 or Nb725_4 (labelled).
Membranes were prepared from E. coli DW2 cells that were transformed with two compatible plasmids derived from pACYC and pCS19 encoding MelBSt and Nb725_4 or Nb725, respectively, and used for the [3H]melibiose active transport assay. After protein concentration determination, 50 μg of total membrane proteins of each sample was analyzed by SDS-15%PAGE and western blot using anti-His tag antibody (HisProbe-HRP Conjugate) as described in the Materials and methods. The western blot result was imaged by the ChemiDoc MP Imaging System (Bio-Rad). MelBSt protein expression presented as the inset in Figure 1c is highlighted by the box. The bands migrating near 25 kDa are non-specific and also presented in the membranes with no MelB nor Nb.
- https://cdn.elifesciences.org/articles/92462/elife-92462-fig1-data4-v1.pdf
Nanobodies (Nbs) binding.
Titrant | Titrate condition | Kd(µM) | Number of tests | Fold of affinity change | p-Value* |
---|---|---|---|---|---|
Nb725_4 | MelBSt/Na+ | 3.64 ± 0.62† | 7 | ||
MelBSt/Na+/melibiose | 11.81 ± 0.72 | 6 | -3.24 | <0.01 | |
MelBSt/Na+/α- †NPG | 14.63 ± 1.27 | 2 | -4.02 | <0.01 | |
MelBSt/Na+/EIIAGlc | 2.14 ± 0.11 | 2 | +1.70 | >0.05 | |
NabFab | 52.53 ± 13.03‡ | 2 | |||
Nb725 | MelBSt/Na+ | 1.58 ± 0.42 | 6 | ||
MelBSt/Na+/melibiose | 8.88 ± 1.24 | 5 | -5.62 | <0.01 | |
MelBSt/Na+/α-NPG | 12.58 ± 0.60 | 2 | -7.96 | <0.01 | |
MelBSt/Na+/EIIAGlc | 1.18 ± 0.12 | 2 | +1.34 | >0.05 | |
NabFab | 36.02 | 1 | |||
Anti-Fab Nb | NabFab | 112.90 ± 16.33‡ | 2 |
-
*
Unpaired t-test.
-
†
Mean ± SEM.
-
‡
nM.
The parent Nb725 had a poor binding to the NabFab (Figure 1—figure supplement 1), but Nb725_4 exhibited greatly increased affinity to NabFab at a Kd value of 52.53 ± 13.03 nM (Figure 1c, Table 1). The anti-Fab Nb binding to NabFab was twofold poorer than Nb725_4 at a Kd value of 112.90 ± 16.33 nM (Figure 1—figure supplement 1d, Table 1). The complex containing all four proteins, including MelBSt, Nb725_4, NabFab, and anti-Fab Nb, showed peak-shift in the gel-filtration chromatography (Figure 1—figure supplement 1e).
Nb725_4 binding properties were further analyzed in the presence of the MelB ligands. As expected, the presence of melibiose yielded six- and threefold inhibition, and α-NPG afforded eight- and fourfold inhibition, on the binding of Nb725 and Nb725_4, respectively (Table 1, Figure 1—figure supplement 2). The physiological regulatory protein EIIAGlc (Table 1, Figure 1—figure supplement 2) showed no inhibition on the binding affinity of both Nbs.
Various ligands binding to the Nb/MelBSt complex in the presence of Na+ were also examined with ITC. As published (Katsube et al., 2023), the melibiose binding was undetectable in the presence of Nb725 (Figure 1—figure supplement 3a). To characterize the sugar effect quantitatively, the α-NPG binding was carried out. Interestingly, the exothermic binding observed in the absence of the Nb changed to endothermic binding reactions in the Nb-bound states; and also, the α-NPG affinity decreased by 21- or 32-fold for Nb725 or Nb725-4, respectively (Table 2, Figure 1—figure supplement 3b). Inhibitions of galactosides have been observed in the case of EIIAGlc/MelBSt complex (Hariharan and Guan, 2014; Hariharan et al., 2015). Collectively, galactosides binding to MelBSt were mutually exclusive with Nbs (Nb725, Nb725_4, or Nb733) or EIIAGlc, and the negative cooperativity implied that MelBSt conformations favored by galactosides and those binders differ. This conclusion was further supported by the Na+ or EIIAGlc binding to the MelBSt/Nb complexes (Table 2, Figure 1—figure supplement 3c and d), which showed no inhibition for Na+ affinity and even slightly better binding for EIIAGlc. Therefore, MelBSt complexed with Nb725_4 retains its physiological functions, and its conformation is expected to be similar when trapped by either of those Nbs and EIIAGlc but is different from the sugar-bound outward-facing structure (PDB ID 7L17) (Guan and Hariharan, 2021).
Nanobody (Nb) effects on MelBSt binding to sugar, Na+, and EIIAGlc.
Titrant | Titrate condition | Kd(µM) | Number of tests | Fold of affinity change | p-Value* | Reference |
---|---|---|---|---|---|---|
Melibiose | MelBSt/Na+ | 1430 ± 30.0† | 5 | / | ||
MelBSt/Na+/Nb725_4 | /‡ | 3 | ||||
MelBSt/Na+/Nb725 | / | 3 | ||||
MelBSt/Na+/EIIAGlc | / | 3 | Hariharan et al., 2015 | |||
α-NPG | MelBSt/Na+ | 16.46 ± 0.21 | 5 | / | ||
MelBSt/Na+/Nb725_4 | 531.55 ± 19.25 | 2 | -32.29 | <0.01 | ||
MelBSt/Na+/Nb725 | 353.55 ± 37.25 | 2 | -21.48 | <0.01 | ||
MelBSt/Na+/EIIAGlc | 76.13 ± 4.52 | 2 | -4.63 | <0.01 | Hariharan et al., 2015 | |
Na+ | MelBSt | 261.77 ± 49.15 | 4 | / | ||
MelBSt/Nb725_4 | 345.04 ± 9.92 | 2 | -1.32 | >0.05 | ||
MelBSt/Nb725 | 182.50 ± 11.30 | 2 | +1.43 | >0.05 | ||
MelBSt/EIIAGlc | 253.50 ± 11.00 | 2 | +1.03 | >0.05 | Katsube et al., 2023 | |
EIIAGlc | MelBSt/Na+ | 3.76 ± 0.29 | 5 | / | ||
MelBSt/Na+/Nb725_4 | 4.32 ± 0.64 | 4 | -1.15 | >0.05 | ||
MelBSt/Na+/Nb725 | 1.94 ± 0.10 | 4 | +1.94 | <0.01 |
-
*
Unpaired t-test.
-
†
Mean ± SEM.
-
‡
Not detectable.
Inward-facing MelBSt trapped by Nb725_4
CryoEM-SPA was used to image the complex consisting of MelBSt in nanodiscs, Nb725_4, NabFab, and anti-Fab Nb. The data collection, processing, and evaluation followed the standard protocols (Figure 2—figure supplements 1–4). From a total number of 296,925 selected particles, structures of MelBSt, Nb725_4, and NabFab were determined to a golden standard Fourier shell correlation (GSFSC) resolution of 3.29 Å at 0.143 (Figure 2). The map for anti-Fab Nb was relatively poor and excluded from particle reconstruction. The quality of the Coulomb potential map allowed for unambiguous docking of available coordinates of NabFab (PDB ID 7PHP), a predicted model for Nb725_4 by Alpha-Fold 2 (Jumper et al., 2021; Bryant et al., 2022), and the N-terminal helix bundle from the outward-facing crystal structure of D59C MelBSt (PDB ID 7L17). The C-terminal helix bundle of MelBSt and the ion Na+ were manually built. The final model contains 417 MelBSt residues (positions 2–210, 219–355, and 362–432), with 6 unassigned side chains at the C-terminal domain (Leu293, Tyr355, Arg363, Tyr369, Tyr396, and Met410) due to the map disorder, 122 of Nb725_4 residues 2–123, and 229 of NabFab H-chain residues 1–214, and 210 of NabFab L-chain residues 4–213, respectively. The local resolution estimate showed that the cores of NabFab, Nb725_4, and the N-terminal helix bundle of MelBSt exhibit better resolutions up to 2.84 Å and most regions in the C-terminal helix bundle of MelBSt have resolutions between 3.2 and 3.6 Å (Figure 2—figure supplement 5a). The N-terminal helix bundle has a completely connected density; however, the map corresponding to three cytoplasmic loops at positions 211–218 in the middle loop6-7, positions 356–361 in loop10-11, or the entire C-terminal tail after Tyr432, is missing. The map quality, model statistics, and the model-map matching Q scores generally matched to this reporting resolution (Table 3, Figure 2—figure supplement 5a–c).
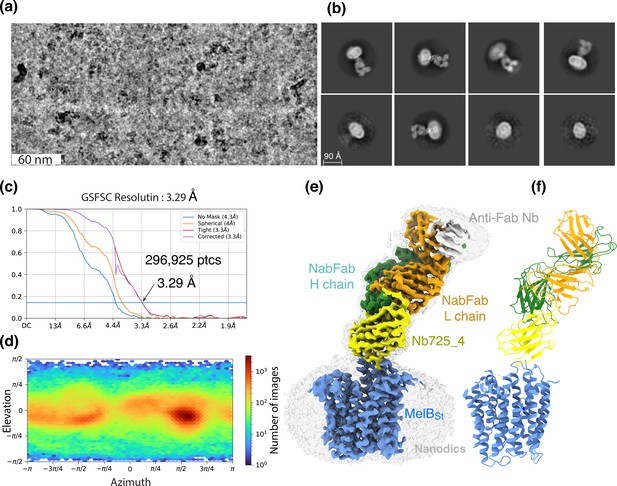
CryoEM single-particle analysis (cryoEM-SPA).
The samplecoum containing the wild-type MelBSt in lipids nanodiscs, the MelBSt-specific Nb725_4, NabFab, and anti-Fab Nb at 1.5 mg/mL in 20 mM Tris–HCl, pH 7.5, and 150 mM NaCl was prepared as described in ‘Materials and methods’. Images were collected using Titan Krios TEM with a K3 detector of S2C2, Stanford, CA. The particle reconstructions and modeling were performed as described in ‘Materials and methods’. The final volume did not include the anti-Fab Nb during Local Refinement due to relatively poor densities. (a) The raw image after motion correction. (b) Representative 2D classes generated by CryoSPARC program. MelBSt in nanodiscs, Nb725_4, NabFab, and anti-Fab Nb can be easily recognized. (c) Golden standard Fourier shell correlation (GSFSC) resolution was calculated by cryoSPARC Validation (FSC) using two half maps generated by the CryoSPARC Local Refinement program. The number of particles used for the volume reconstruction is presented. (d) Particle distribution of orientations over azimuth and elevation angles generated by CryoSPARC Local Refinement program. (e, f) The structure of MelBSt/Nb725_4/NaFab complex. The volume (e) and cartoon representation (f) were colored by polypeptide chains as indicated. Nanodiscs were transparent and colored in light gray. Sphere and sticks in panel (f) highlighted Na+ and its ligands.
CryoEM data collection and structure determination statistics.
MelBSt/Nb725m/NabFab | |||||
---|---|---|---|---|---|
EMDB | EMD-41062 | ||||
PDB | 8T60 | ||||
Non-tilted collection | Tilted collection | ||||
Data collection | |||||
Microscope | Krios-TEMBETA | Krios-TEMBETA | |||
Voltage (kV) | 300 | 300 | |||
Number of movies | 14,094 | 8716 | |||
Electron dose (e-/Å2) | 50.00 | 50.00 | |||
Defocus range (μm) | −0.8 to –1.8 | −0.8 to –1.8 | |||
Pixel size (Å) | 0.86 | 0.86 | |||
Plate tilt angel (°) | / | 30 | |||
Data processing | |||||
Initial number of particles | 7,632,727 | 2,887,147 | |||
Combined final number of particles | 203,876 | ||||
Symmetry imposed | C1 | ||||
Map resolution* (Å) | 3.29 | ||||
B factor | 101.4 | ||||
Model refinement | |||||
Chains | 5 | ||||
Non-hydrogen atoms | 7503 | ||||
Protein residues | 978 | ||||
Mean B-factor | |||||
Protein | 97.35 | ||||
Na+ ion | 88.64 | ||||
RMS deviations | |||||
Bond lengths (Å) | 0.003 | ||||
Bond angles (°) | 0.509 | ||||
MolProbity score | 1.82 | ||||
Clash score | 4.09 | ||||
Poor rotamers (%) | 1.98 | ||||
Ramachandran plot | |||||
Favored (%) | 93.48 | ||||
Allowed (%) | 6.52 | ||||
Outliers (%) | 0.00 | ||||
Model resolution (Å)† | 3.2/3.3/3.5 | ||||
-
*
Resolution determined by Fourier shell coefficient threshold of 0.143 for corrected masked map.
-
†
Resolution determined between the model and the resolved map by Fourier shell coefficient threshold of 0/0.143/0.5.
The NabFab structure in this complex is virtually identical to that in the NorM complex (PDB ID 7PHP) (Bloch et al., 2021) with rmsd of all atoms of 1.334 Å (Figure 2—figure supplement 6a–c). When the alignment focused on the NabFab H-chain, the two NabFab/Nb binary complexes in both complexes were organized similarly (Figure 2—figure supplement 6d), and the binding of Nbs with their corresponding transporters varied.
The structure shows that the Nb725_4 is bound to the cytoplasmic side of MelBSt at an inward-open conformation (Figure 2—figure supplement 7a–c), which supports the functional analyses (Katsube et al., 2023) and reveals the MelBSt conformation for the Nb binding. The contact surfaces match in shape and surface potential with a contact area of 435 A2. CDR-1 (Arg30, Asp31, Asn32, and Ala33) and CDR-2 (Tyr52, Asp53, Leu54, Tyr56, Thr57, and Ala58) form interactions with two cytoplasmic regions of MelBSt, the tip between helices IV and V, that is, loop4-5 (Pro132, Thr133, Asp137, Lys138, and Arg141) and the beginning region of the middle loop6-7 (Tyr205, Ser206, and Ser207) (Figure 2—figure supplement 7c). The Nb binding was stabilized by salt-bridge and hydrogen-bonding interactions at both corners of this stretch of inter-protein contacts. In the published outward-open conformation of MelBSt, such a binding surface for recognizing Nb725_4 does not exist. Most regions of the middle loop, the C-terminal helix bundle, and the C-terminal tail helix must be displaced to permit the binding, and some of those regions are completely disordered in this inward-facing structure. All data supported that Nb725_4 only binds to MelBSt in an inward-open state. Thus, it is a conformation-selective Nb.
Nb725_4, Nb725, and another Nb733 (Katsube et al., 2023) behave similarly; all inhibit the sugar binding and transport. We conclude that all three binders are the inward-facing conformation-specific negative modulators of MelBSt. Further, ITC measurements (Figure 1—figure supplement 2) showed that MelBSt binds to either of three Nbs in the absence or presence of EIIAGlc or binds to EIIAGlc in the absence or presence of either Nbs. The complex composed of MelBSt, EIIAGlc, and Nb725 (or Nb733; Katsube et al., 2023) can be isolated (Figure 2—figure supplement 8).
MelBSt trapped in a Na+-bound, low sugar-affinity state
Between helices II and IV (Figure 3a and b), one Na+ cation was modeled, liganded by four side -chains, Asp55, Asn58, Asp59 (helix II), and Thr121 (helix IV), at distances of between 2.1 and 2.8 Å. The backbone carbonyl oxygen of Asp55 is also in close proximity to the bound Na+. The local resolution of this binding pocket is approximately 2.9–3.2 Å. The model to map Q-scores for Na+ and the binding residues and side chains are generally greater than the expected scores at this resolution except for the Thr121 side chain, which has a lower score (Pintilie et al., 2020). The observation of this cation-binding pocket is supported by previous extensive data (Hariharan and Guan, 2017; Ding and Wilson, 2001; Katsube et al., 2022). Cys replacement at position Asp59 changes the symporter to a uniporter (Guan and Hariharan, 2021). The D59C mutant does not bind to Na+ or Li+ as well as loses all three modes of cation-coupled transport but catalyzes melibiose fermentation in cells (melibiose concentration-driven transport) (Guan and Hariharan, 2021) or melibiose exchange in membrane vesicles (Ethayathulla et al., 2014). Asp55 also plays a critical role in the binding of Na+ or Li+ and in the Na+- or Li+-coupled transport (Ethayathulla et al., 2014; Hariharan and Guan, 2017). Extensive studies of Escherichia coli MelB also show that both negatively charged residues are involved in cation binding (Granell et al., 2010; Pourcher et al., 1993; Zani et al., 1993). Thr121 has been determined to be critical for Na+, not H+ nor Li+ (Katsube et al., 2022); and the Cys mutation at Asn58 also loses Na+ binding (Ethayathulla et al., 2014). Interestingly, the Na+ recognition can be selectively eliminated by specific mutations. Further, the Na+ binding is supported by MD simulations at both inward and outward states using MD simulations in the absence of the Nb (Figure 3—figure supplement 1), which reveals the ideal coordination geometry for Na+ binding.
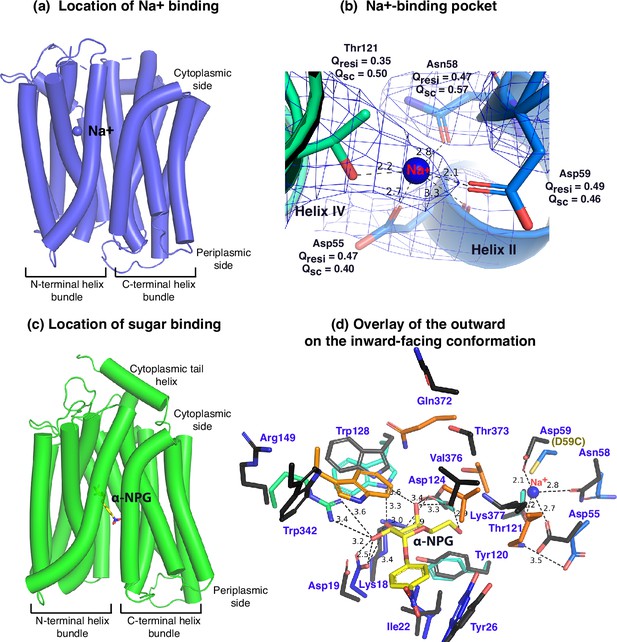
Na+- and sugar-binding pockets of MelBSt.
(a) Location of the Na+ binding site. The inward-facing cryoEM structure of the WT MelBSt is displayed in cylindrical helices with the cytoplasmic side on the top. One bound Na+ ion within the N-terminal helix bundle was shown in the blue sphere. (b) Na+-binding pocket. The isomesh map of the inward-facing conformation was created by the Pymol program using level 10 and carve of 1.8. The Na+ coordinates are shown in dashed lines (Å) and interacting residues are shown in sticks. Qres, Q score for residue; Qsc, Q score for side chain. (c) Location of the galactoside-binding stie. The outward-facing x-ray crystal structure of D59C MelBSt mutant is displayed in cylindrical helices with the cytoplasmic side on the top (PDB ID 7L17). One α-nitrophenyl galactoside (α-NPG) molecule is shown in the stick colored in yellow between the N- and C-terminal helix bundles. (d) Superimposed sugar- and cation-binding pockets. The α-NPG-bound outward-facing structure in c was aligned with the inward-facing cryoEM structure based on the 2–200 region. The residues in the sugar- and cation-binding pockets of the inward-facing cryoEM structure are colored in black and labeled in blue. D59C of the α-NPG-bound outward-facing structure is indicated in the parentheses. The α-NPG and Na+ are colored yellow and blue, respectively.
The crystal structure of sugar-bound D59C MelBSt (Figure 3c and d, Figure 3—figure supplement 2), which retains an intact sugar-binding and translocation pathway, shows that the sugar-binding pocket is formed by both N- and C-terminal bundles (Guan and Hariharan, 2021). Due to the Cys mutation, the cation-binding pocket is loosely packed and this mutant does not bind Na+ or Li+. When overlaying the outward-facing crystal structure upon the inward-facing cryoEM structures (Figure 3d, sticks in black), little variation of the positioning of the sugar-binding residues on the N-terminal bundle is observed except for Arg149, but all C-terminal residues involved in the sugar-binding pocket show greater displacements. In the cation-site mutant, Lys377 forms a salt-bridge interaction with Asp55; in the Na+-bound structure, Lys377 is closer to Asp124, and Asp55 is liganded with Na+.
The loosely arranged sugar-binding residues observed in the Nb725_4-bound conformation provide the structural foundation for measured lower sugar affinities (Table 2), so this structure represents an intracellular Na+-bound sugar-releasing conformation. Since the positioning of the key residues for galactoside specificity (Lys18, Asp19, and Asp124) exhibits virtually no change, it is conceivable that this conformation retains the initial recognition of sugar for reversal reactions, a common feature for carrier transporters (Guan et al., 2011; Guan and Kaback, 2009).
Conformational changes between inward- and outward-facing states
The outward-facing structure was trimmed to match the residues resolved in the inward structure. The superposition of the outward- and inward-facing MelBSt exhibited rmsd of all atoms of 5.035 Å (Figure 3—figure supplement 3), and the major displacement exists at both ends of transmembrane helices. The alignment based on the N-terminal positions 2–200 or the C-terminal positions 231–432 exhibits a clear difference in the angle of the domain packing (Figure 3—figure supplement 3b and c). The focused alignment of positions 2–200 or 231–432 exhibits greatly reduced rmsd values (Figure 3—figure supplement 3d and e). The N-terminal domains at both conformations are virtually the same, especially for the helices I, II, and IV that host the sugar- and cation-specificity determinant pockets (Figure 3—figure supplement 3f). Some structural rearrangements within 3–5 Å exist in the C-terminal bundle, especially on both ends of cavity-lining helices (VII, VIII, X, and XI) and their link or extended loop (Figure 3—figure supplement 3g and h). Thus, the conformational changes between the outward- and inward-facing states can be adequately described by a rocker-switch-like movement with some structural rearrangements in the C-terminal bundle as also indicated in Figure 3—figure supplement 4.
Substrate translocation barriers
The two structures clearly reveal the two essential barriers critical for substrate translocation, the thicker inner barrier in the outward structure and the thinner outer barrier in the inward structure (Figure 4a and d). The core of the inner barrier is formed by three-helix pairs from both domains (V/VIII, IV/X, and back II/XI), and stabilized by an extensive charged network (Figure 4b; Ethayathulla et al., 2014; Markham et al., 2021; Guan and Hariharan, 2021; Amin et al., 2014). The N-terminal Arg141 (helix V) forms salt-bridge interactions with the C-terminal Asp351 and Asp354 (helix X), and this core interaction links with four other charged residues to stabilize the cytoplasmic closure by forming an inner barrier. In addition, the inner barrier is also stabilized by two peripheral amphiphilic helices at the cytoplasmic side (in the middle loop and the C-terminal tail) (Figure 4b). The entire middle loop6-7 across the two domains interact with broader areas (the N-terminal loop2-3, loop4-5, and helices II and IV, as well as the C-terminal loop10-11, helices X and XI, and the C-terminal tail). The C-terminal tail helix interacts with both N- and C-terminal domains (helices V and X). The contacting area between the two bundles (based on positions 2–212 and 213–450) is 2218 Å (Lin et al., 2015) with nine salt-bridge and five hydrogen-bounding interactions.
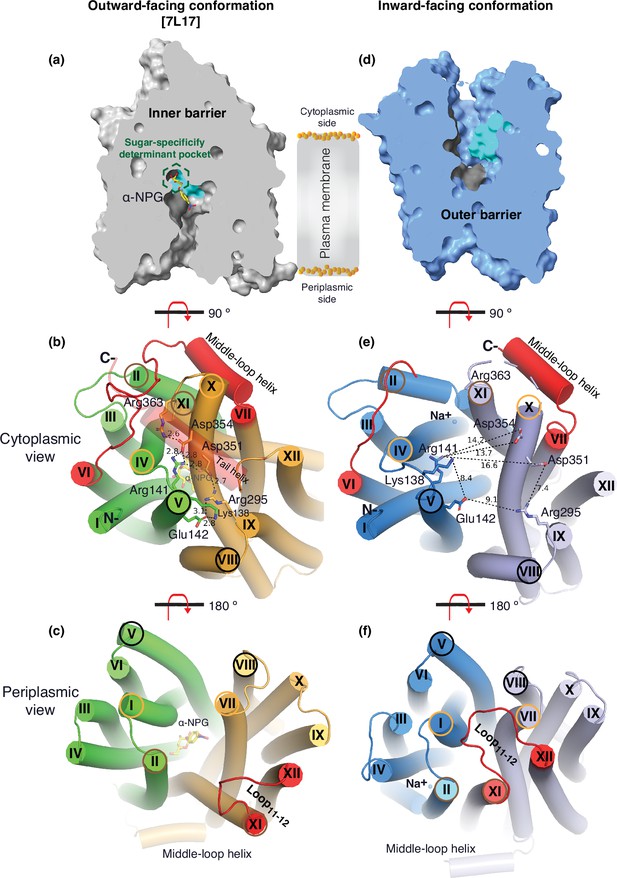
Barriers and sugar-binding pocket.
Outward-facing (PDB ID 7L17; left column) and inward-facing (right column) structures were used to prepare the figures. (a, d) Side view with cytoplasmic side on top. The inner and outer barriers are labeled. The sugar-specificity determinant pocket is highlighted in a dashed hexagon. The residues contributing to the bound α-NPG in N- and C-terminal bundles are colored in dark gray and cyan, respectively. (b, e) Cytoplasmic view. The charged network between the N- and C-terminal bundles is colored in green and bright orange in panel (b), or blue and light blue in panel (e), respectively. The C-terminal tail helix was set in transparent in panel (b) but disordered in the inward-facing conformation in panel (e). The charged residues are highlighted in sticks. Arg363 side chain missed the side chain in the inward-facing structure. (c, f) Periplasmic view. The paired helices involved in either barrier formation are highlighted in the same colored circles. The α-NPG is colored in yellow and Na+ is shown in blue sphere. The cytoplasmic middle loop, the C-terminal tail, and the periplasmic loop11-12 are highlighted in red. Distance between two residues is shown by dashed lines (Å).
The core of the outer barrier in the inward-facing structure is also formed by three pairs of helices from both domains (V/VIII, I/VII, and II/XI) (Figure 4f). The longest loop11-12 at the periplasmic side moves into the middle area and forms contacts with helices I and VII, sealing the periplasmic opening. Notably, helical pair I/VII or IV/X is only involved in the outer or inner barrier, respectively, and the other two cavity-lining helical pairs (V/VIII and II/XI) are engaged in both barriers (Figure 4b and c, e, f). The contact area between the two bundles at the periplasmic side of 1435 Å (Lin et al., 2015) is much smaller. These structural features further supported that the outward-facing state is more stable than the inward-facing MelBSt. In the inward-facing conformation, all of the salt-bridge interactions are broken (Figure 4b and e), and the Arg141 and Lys138 engage in the binding of Nb725_4 (Figure 2—figure supplement 7). Therefore, this cytoplasmic-side salt-bridge network is the inner barrier-specific.
Assessed by the sugar-bound structure (Figure 4a), the substrate-binding pocket is outlined by Trp128 and Trp 342 on the inner barrier and Tyr26 on the outer barrier (Figure 3d, Figure 3—figure supplement 1), part of both barriers. Notably, the major binding residues that involve the multiple hydrogen-bonding interactions with the galactosyl moiety are only part of the inner barrier. In the inward-facing state, the inner barrier is broken, which exposes the binding site to the cytoplasm and also results in the interruption of the sugar-binding pocket (Figure 4d). The C-terminal binding residues moved away due to the displacement of the backbone of helices X/XI, and the sugar-binding residues become loosely organized, resulting in a low sugar-binding affinity. The structural features indicated that the molecular mechanisms for the sugar translocation simply result from the barrier switch generating a low sugar-affinity inward state of MelB. The outer barrier does not contain residues essential for galactoside binding, so the break of the outer barrier has a much less profound effect on the sugar-binding affinity.
Conformational dynamics measured by HDX-MS
Bottom-up HDX-MS measures the exchange rate of amide hydrogens with deuterium by determination of time-dependent peptide level mass spectra (Masson et al., 2019). It is a label-free quantitative technique that can disclose the dynamics of a full-length protein simultaneously. This approach was utilized to determine MelBSt dynamics in this study. The Na+-bound WT MelBSt proteins exist in multiple conformations including at least the outward- and inward-facing states but strongly favor the outward-facing states. The Nb725_4-trapped MelBSt exists only in an inward-facing conformation. A pair of WT MelBSt and WT MelBSt complexed with Nb725_4 was subjected to in-solution HDX-MS experiments. MelBSt yields 86% coverage with 155 overlapping peptides at an average peptide length of 9.3 residues (Figure 5a and b, Figure 5—source data 1, Table 4), leaving 66 non-covered residues; most are located in the middle regions of transmembrane helices or in a few cytoplasmic loops (Figure 6, ball in gray).
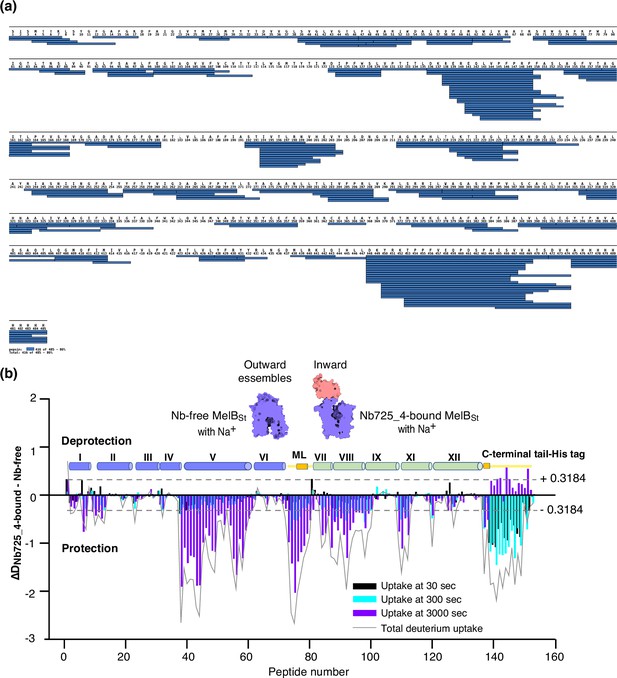
MelBSt dynamics probed by hydrogen/deuterium exchange mass spectrometry (HDX-MS).
(a) MelBSt peptide sequence coverage. The peptides of the deuterated MelBSt were determined based on the MelBSt peptide database that was generated by nonspecific digestions of non-deuterated MelBSt as described in ‘Materials and methods’. Peptides were confirmed in the HDX-MS experiment. Blue bar, the covering of each peptide. The amino-acid sequencing identification number should be –1 for each position due to the processed Met at position 1. The 10xHis Tag was included in the data analysis. (b) Residual plots (DNb725_4-bound - Nb-free) against the overlapping peptide numbers for each time point and the sum of uptake. MelBSt alone or bound with Nb725_4 in the presence of Na+ were used to carry out the HDX reactions as described in ‘Materials and methods’. Black, cyan, and blue bars, the deuterium uptake at 30, 300, and 3000 s, respectively; gray curve, the sum of uptake from all three time points. Deprotection, ΔD (DNb725_4-bound – Nb-free) > 0; protection, ΔD < 0. Each sample was analyzed in triplicates. Cylinders indicate the helices and the transmembrane helices are labeled in Roman numerals. The length of the cylinder does not reflect the length of corresponding helices but is estimated for locations of the deuterium-labeled overlapping peptides. Noteworthy, the uncovered regions were not included. ML (cytoplasmic middle loop) and C-terminal tail including the His tag are colored in yellow. Dashed lines, the threshold.
-
Figure 5—source data 1
Output results of HDExaminer software analysis for Figures 5 and 6.
- https://cdn.elifesciences.org/articles/92462/elife-92462-fig5-data1-v1.csv
HDX reaction and labeling details.
Samples measured | WT MelBSt | WT MelBSt complexed with Nb725m |
---|---|---|
HX reaction buffer | 25 mM Tris-HCl, pD 7.5, 150 mM NaCl, 10% Glycerol, and 0.01% DDM | |
Reaction temperature (°C) | 20 | |
HX time course (s) | 0, 30, 300, 3000 | |
Number of peptides | 153 | |
Sequence coverage by labeling | 86% | |
Mean peptide length | 9.3 | |
Average redundancy | 2.9 | |
Replicates (technical) | 3 | |
|ΔD| (Da) | 0.3071 | |
Back exchange rate | Not appliable |
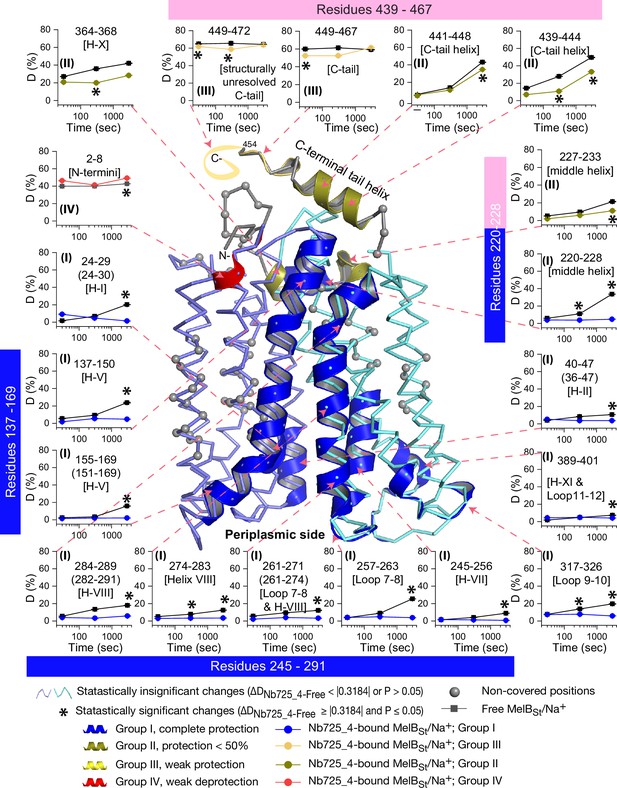
Peptide mapping of hydrogen/deuterium exchange (HDX) results.
HDX results are presented in Figure 5. Any peptide of ΔD with p≤0.05 and ΔD ≥ |0.3184| were treated as significant. The peptides with statistically significant differences at the 3000 s time point were mapped on the outward-facing structure (PDB ID 7L17). Inset, the deuterium uptake time course of representative peptides was plotted as a percentage of deuterium uptake relative to the theoretic maximum number (D%). The peptides, either as a single peptide or a group of overlapping peptides (in the parentheses), are labeled and indicated in the structure by the pink arrow in dashed lines. In the brackets, the corresponding secondary structure or loops. Error bar, SEM; the number of tests, 3. Other symbols are presented within the figure.
The effect of MelBSt structural dynamics imposed by Nb725_4 is presented as the differential deuterium labeling (ΔD). As shown in the residual plot of each peptide between the two states (DNb725_4-bound – Nb-free) (Figure 5b) at three labeling time points, variable levels of protections (less deuterium exchange in the complex state) are observed in MelBSt. A slight deprotection is only observed at the N-terminus. After proper statistical analysis, there are 232 positions (>50% labeled positions) exhibiting insignificant HDX changes (DNb725_4-bound – Nb-free value < |0.3184| or p>0.05) as indicated by ribbon representation (Figure 5b). The remaining 177 positions, not counting the His tag, covered by 71 overlapping peptides show statistically significant changes (DNb725_4-bound – Nb-free value ≥ |0.3184| and p≤0.05) and are highlighted by the cartoon representation (Figure 6). Overall, those regions are mainly distributed on the cavity-lining helices and cytoplasmic peripheral regions including the middle loop and C-terminal tails, as well as the C-terminal periplasmic loops. The corresponding deuterium uptake curves covering three-magnitude time points were plotted (Figure 6—figure supplements 1 and 2) and the representative peptides are displayed as insets around the structure (Figure 6, insets). Most Nb725_4 binding residues are not covered, either not covered by the labeled peptides (Tyr205, Ser206, and Ser207) or by insignificant change (Pro132 and Thr133), except for Asp137, Lys138, and Arg141 that were presented in eight peptides. Depending upon the exchange rate and response to the binding of Nb725_4, the MelBSt HDX can be categorized into four groups.
Group I peptides show low-level HDX exchange rates and Nb725_4 afforded complete inhibition for up to at least 50 min (Figure 6, cartoon and curve in blue). These peptides are located in transmembrane helices, middle-loop helix, and C-terminal periplasmic loops across both domains and most are clustered at the cavity-lining helices. Peptides carrying the Nb-binding residues Asp137, Lys138, and Arg141 are marked in the plots (Figure 6, inset; Figure 6—figure supplements 1 and 2). Group II peptides show faster deuterium uptake over time (Figure 6, cartoon and curve in deep olive). The Nb725_4 binding affords small to moderate protections. The group II peptides are spotted at three cytoplasmic regions; interestingly, all surround the helix X, which plays a critical role in the stability of the inner barrier. At the inward-facing structure, most of those residues are either missing or poorly resolved. The deuterium uptakes in the Nb725_4-bound MelBSt complex likely result from structural flexibility.
Group III peptides show higher-level HDX exchange rates of greater than 60% for both bound and free states even at 30 s (Figure 6, drawing in yellow). Nb725_4 only affords short-time, weak protections. They are located in the C-terminal tail unstructured region, and most of them are structurally unresolved due to severe disorders. Group VI peptide only contains the short N-terminal tail showing a medium-level uptake (40–50%) across all labeling times, and Nb725_4 affords a slight deprotection due to allosteric changes upon the Nb725_4 binding (Figure 6, cartoon and curve in red). The results show that groups III and IV peptides covering both tails are solvent-accessible dynamic regions, which is consistent with the notion that they play little role in transport-critical conformational transitions.
The 30 s deuterium uptake in the absence of Nb725_4 for the first two groups was further analyzed. Among the five group I and four group II members with faster exchanges as judged by the shortest reaction time of 30 s (Figure 6—figure supplement 3), except for the two periplasmic loop7-8 (peptide 261–271) and loop9-10 (peptide 317–326), others are clustered on the cytoplasmic side. In the outward-facing structure (Figure 7a), the spotted peptides pack against one another: helix-V (peptide 137–150) with helix-VIII (peptide 284–289), and the middle-loop helix (peptide 220–233) with helix-X (peptide 364–368). All surround the conformation-dependent charged network. Notably, this short peptide 137–150 links the sugar-binding residue Arg149 to the charged network by residues Lys138, Arg141, and Glu142 (Figure 4b). In the inward-facing structure (Figure 7b), these packed helices were moved against one another. Thus, these higher dynamic regions may play important roles in responding to the ligand binding and initiating the global conformational transition.
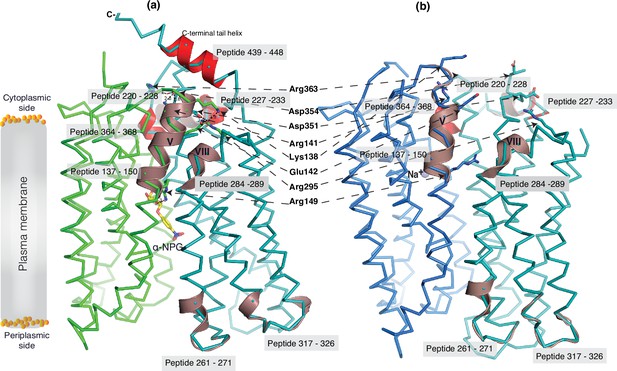
Dynamic regions of MelBSt.
The peptides that exhibited faster hydrogen/deuterium exchange (HDX) rates (>5% at 30 s) were mapped on both the α-NPG-bound outward-facing structure (7L17) in panel (a) and the inward-facing cryoEM Nb725_4-bound structure in the panel (b), respectively. All peptides are labeled and highlighted in a transparent gray box. The charged residues forming the inner barrier-specific salt-bridge network are highlighted in sticks and indicated by black arrows in dashed lines. In the Nb725_4-bound structure (b), the C-terminal tail was disordered. The cartoon colored in dirty violet, the group I peptides with faster HDX rate; the cartoon colored in red, group II peptides. The α-NPG is highlighted in yellow and Na+ is shown in blue sphere.
Discussion
Formation and stabilization of a high-energy inward-facing conformation of MelBSt in a form suitable for structure determination by cryoEM-SPA was a technically challenging sample preparation problem, which we solved by the formation of a complex of MelBSt with a conformation-selective binder and fiducial NabFab. The resulting structure, a high-energy inward-facing, low sugar-affinity state of MelBSt with a bound Na+, represents the intracellular sugar-releasing/rebinding state in the stepped-binding model for the mechanism of coupled transport (Figure 8; Guan, 2018; Guan and Hariharan, 2021). Our previous results on the cooperativity of binding (Hariharan and Guan, 2021) supported the contention that Na+ binding increases the melibiose affinity to allow melibiose uptake at lower available concentrations, a critical evolved mechanism for cation-coupled transporters. The new Na+-bound sugar-releasing inward-facing cryoEM structure provides a structural basis to support the transport model based on a sequential process of the sugar-binding after Na+ binding first and sugar release prior to Na+ release (Figure 8).
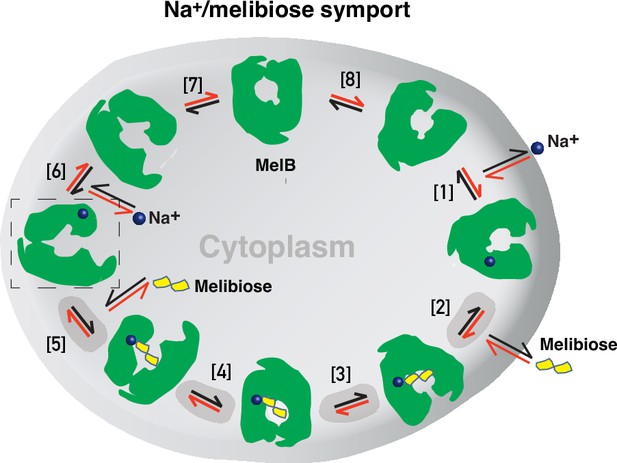
Stepped-binding model for the Na+/melibiose symport catalyzed by MelB.
Eight states are postulated including transient intermediates. In this reversal reaction, the cation binds prior to the sugar and releases after sugar release. Melibiose active transport or inflex begins at step [1] and proceeds via the red arrows clockwise around the circle, with one melibiose and one cation inwardly across the membrane per cycle. Melibiose efflux begins at step [6] and proceeds via the black arrows anticlockwise around the circle, with one melibiose and one cation outwardly across the membrane per circle. Melibiose exchange begins at step [6], and only takes four steps involving steps [2–5] as highlighted in gray color. The low-sugar affinity inward-facing Na+-bound cryoEM structure represents the state after the release of sugar, as indicated by a dashed box.
The alternating-access movement has been widely used to describe the conformational change of transporters (Abramson et al., 2003; Huang et al., 2003; Boudker et al., 2007; Yan, 2015; Drew et al., 2021; Guan and Kaback, 2006; Khare et al., 2009). This description focuses on how the substrates access their binding site or leave the protein. The core symport mechanism for coupled substrate translocations is the mobile barriers involving the inner and outer barriers as proposed originally by Peter Mitchell (Mitchell, 1990; Figure 4a and d). Our binding thermodynamic cycle study on the determination of heat capacity change (ΔCp) (Hariharan and Guan, 2021) showed positive values with the binding of one substrate, suggesting the dominance of hydrations and opening of the transporter, and negative values with the second substrate binding, suggesting dehydration and transition to the occluded intermediate state as indicated at step (3) in Figure 8. The data fit well with a barrier mechanism that prevents a single substrate molecule from crossing the protein to favor obligatory co-translocation. When this inner barrier breaks as a result of the conformational switch induced by the binding of two substrates, the sugar-binding specific pocket which is part of this inner barrier also breaks (Figure 4e), creating the intracellular sugar low-affinity state and the sugar-releasing path. Collectively, based on structural and thermodynamical analyses, all show that cooperative binding is integral to barrier switching in the core symport mechanism.
For substrate translocation, historically, a two-binding-site walking model was proposed: a high-affinity site at the outer surface and a low-affinity site at the inner surface. In this functional and structural paradigm, a substrate molecule moves across the protein from its high-affinity binding site to a low-affinity site for subsequent release. This canonical model has been challenged in LacY as the same binding affinity was measured at both the inner and outer surfaces (Guan and Kaback, 2004), and a single substrate-binding site was also observed in its 3D structures (Abramson et al., 2003; Huang et al., 2003; Guan and Kaback, 2006). Therefore, a single-binding site in the middle of the protein has been the current canonical model. Actually, one of the major challenges in measuring the substrate binding in membrane transporters is the intrinsic dynamic feature of the transport proteins, which makes it difficult to attribute the outcome to a specific state. Therefore, the substrate binding affinity measured in a bulk sample is an average of binding affinities from all of the individual molecules in a distribution. It is also challenging to establish a correlation between measured binding affinities and experimentally determined structures. We approached this problem by using the conformation-specific binding protein Nb725_4 to decouple the binding and conformational dynamics shifting conformers to a single state or a narrowed cluster, that is, through experimental modulation of the distribution. The results of the structural determination and binding analysis in the combination of the conformation-selective Nb permitted the extension of the current single substrate-binding site (and single affinity) model to a more refined model of one site possessing conformation-dependent higher-affinity and lower-affinity states. Identification of this critical lower-affinity inward-open state of the sugar-binding site is significant for understanding the molecular basis of higher intracellular substrate accumulation.
The shared cation site for Na+, Li+, or H+, which is hosted only by the N-terminal two helices (II and IV), is in close proximity but not part of the mobile barrier at either outward- and inward-facing state. The inward-facing state retains the Na+ binding affinity (Figure 1—figure supplement 3d, Table 2) and the bound Na+ ion was observed (Figure 3a and b). Consistently, the simulated Na+-binding site at both outward- and inward-facing conformations is virtually identical (Figure 3—figure supplement 1), which is in stark contrast to the conformation-dependent sugar binding in MelBSt. No conformational dependency of Na+ binding might suggest the mechanism for the reversible reaction. It is likely that this Na+-binding site co-evolved with the E. coli intracellular Na+ homeostasis maintained to 3–5 mM (Hunte et al., 2005) to achieve optimal activity.
To interrogate the structural dynamics of MelBSt and conformational switch mechanisms, an HDX-MS comparability study in the absence or presence of the conformational Nb725_4 was performed. HDX-MS is a powerful approach for characterizing protein conformational flexibility and dynamics (Masson et al., 2019; Zheng et al., 2019) and has recently been applied to membrane transport proteins (Zheng et al., 2017; Jia et al., 2020; Zmyslowski et al., 2022). Under experimental conditions, two major parameters (solvent accessibility and hydrogen protonation) contribute to the H/D exchange rate. The residual plot clearly indicates that the major effects of Nb725_4 binding are the reduction of the conformational dynamics of MelBSt (Figure 5b). Stronger protections are observed at both transmembrane helix bundles, especially both ends of the cavity-lining helices (Figure 6, cartoon in blue), which marks the most conformationally dynamic regions that correlate to the conformational transition in MelBSt. Notably, the Nb-free Na+-bound WT MelBSt exists in both outward- and inward-facing conformations with a bias toward the outward-facing state (Ethayathulla et al., 2014; Guan and Hariharan, 2021), and the MelBSt in the Nb725_4 complex exists only as an inward-facing conformational ensemble. Therefore, the differences observed by HDX-MS between the two samples in the absence and presence of Nb725_4 likely disclosed, to some extent, the MelBSt global conformational transitions, in addition to the atomic motions.
While the resolution of HDX is at a peptide level, the data showed marked effects on two well-conserved cytoplasmic helices of MFS transporters (on the middle loop and the C-terminal tail). Those peripheral amphiphilic helices are likely to play important roles in the stability of the inner barrier. Consistently, a recent atomic force microscopy study has shown that the middle loop becomes loosely packed in the presence of both sugar and cation (Blaimschein et al., 2023).
As described, the peptide 137–150 of helix V contains a sugar-binding residue Arg149 and three conformation-critical residues Lys138, Arg141, and Glu142 in the inner barrier-specific charged network (Figure 4). Extensive site-directed mutagenesis and second-site suppression showed that this charged network play important role in the conformational changes and the mutations at all three Arg at positions 141, 295, and 363 (Figure 4b) can be substantially rescued by a single mutation D35E (helix I) located at the other side of the membrane (Amin et al., 2014). Arg141 and Glu142 of E. coli MelB have been shown to participate in the conformational transition (Meyer-Lipp et al., 2006). Pro148 has been identified by second-site mutation to reduce the transport Vmax of a Vmax elevated mutant by modulating the rate of conformational change (Jakkula and Guan, 2012). Thus, the helix-V dynamic region (peptide 137–150) could link the sugar-binding pocket with the inner barrier-specific salt-bridge network. Together with the interactions with the dynamic regions (residues 284–289) of helix VIII, the substrate binding occupancy could be propagated, via an allosteric mechanism, from the sugar-binding pocket to the cytoplasmic peripheral helices as a pre-transition for the opening of the transporter to the cytoplasm (Blaimschein et al., 2023).
All of the conformation-selective Nbs (Nb725, Nb733, and the hybrid Nb725_4) are expected to bind MelBSt similarly and trap it at the high-energy state, which is consistent with the observation of very low-frequency occurrence during Nbs selection (Katsube et al., 2023). The effects of the Nbs binding mimic the regulatory effect imposed by EIIAGlc binding (Hariharan and Guan, 2014; Hariharan et al., 2015), that is, reduction of sugar-binding affinity with no change in the Na+ binding. Interestingly, either Nbs or EIIAGlc can bind to MelBSt in the absence or presence of the other (Table 2, Figure 1—figure supplements 2 and 3); the complex composed of all three proteins can be isolated (Figure 2—figure supplement 8; Katsube et al., 2023). The extensive comparisons strongly support the notion that the Nbs and EIIAGlc can concurrently recognize two spatially distinct epitopes of MelBSt, and the inward-facing conformation trapped by the Nb mimics the conformation of MelBSt under the EIIAGlc regulation. Available data showed that three positions (Asp438, Arg441, and Ile445) on one face of the C-terminal tail helix of MelBSt might be involved in EIIAGlc binding (Kuroda et al., 1992), and this region is conserved with the later known EIIAGlc binding site in MalK (Chen et al., 2013). Thus, the cytoplasmic surface of the C-terminal domain could potentially support the EIIAGlc binding at the inward-facing state; unfortunately, the C-terminal tail helix is disordered in this Nb-bound inward-facing structure.
In this study, we demonstrated that the mobile barrier mechanism plays a central role in substate translocation, the barrier dynamics are modulated by the cooperative binding of substrates, and the inner/outer barrier shifting directly regulates the sugar-binding affinity, with no effect on the Na+ binding. Furthermore, we proposed that the functional inhibition of this MFS transporter by the central regulatory protein EIIAGlc is achieved by trapping MelBSt in a low-sugar affinity inward-facing state. Overall, our studies provide substantial information to explain the obligatorily coupled transport of Na+ and sugar substrate in structural and dynamic terms collectively.
Materials and methods
Reagent type (species) or resource | Designation | Source or reference | Identifiers | Additional information |
---|---|---|---|---|
Strain, strain background melB (Salmonella typhimurium) | S. typhimurium strain LT2/SGSC1412/ATCC | PMID:1495487 | STM4299 | Used for melB cloning |
Strain, strain background (Escherichia coli) | DW2 | PMID:3047112 | melA+ ΔmelB ΔlacZY | MelB expression and transport analysis |
Strain, strain background (E. coli) | XL1 Blue | Agilent Technologies | recA1 endA1 gyrA96 thi-1 hsdR17 supE44 relA1 lac [F′ proAB lacIqZΔM15 Tn10 (Tetr)] | Plasmid amplification |
Strain, strain background (E. coli) | ArcticExpress (DE3) | Agilent Technologies | F– ompT hsdS(rB – mB –) dcm+ Tetr gal λ(DE3) endA Hte [cpn10 cpn60 Gentr] | Protein expression |
Strain, strain background (E. coli) | DH5α cyaA- | PMID:37380079 | ΔcyaA | Two-hybrid assay |
Strain, strain background (E. coli) | BL21(DE3) T7 express | New England Biolabs | fhuA2 lacZ::T7 gene1 [lon] ompT gal sulA11 R(mcr-73::miniTn10--TetS)2 [dcm] R(zgb-210::Tn10--TetS) endA1 Δ(mcrC-mrr)114::IS10 | Protein expression |
Strain, strain background (E. coli) | BL21(DE3) C43 | PMID:8757792 | F– ompT hsdSB (rB- mB-) gal dcm (DE3) | Protein expression |
Strain, strain background (E. coli) | BL21(DE3) pRIL | Agilent Technologies | F– ompT hsdS(rB – mB –) dcm+ Tetr gal endA Hte [argU ileY leuW], Camr | Protein expression |
Recombinant DNA reagent (plasmid) | pCS19 | PMID:10319814 | pQE60 derivative inserted with gene lacIq; ampr | Cloning/expressing vector |
Recombinant DNA reagent (plasmid) | pCS19/FX | PMID:25627011 | Expression vector derived from pCS19 with two SapI sites and ccdB gene for FX cloning; ampr | |
Recombinant DNA reagent (plasmid) | pACYC | PMID:2190220 | Expression vector; no ccdB gene: camr | |
Recombinant DNA reagent (plasmid) | pACYC/FX | PMID:25627011 | Expression vector derived from pACYC; with ccdB gene, camr | |
Recombinant DNA reagent (plasmid) | pACYC/MelBSt | PMID:25627011 | Expression plasmid for MelBSt derived from pACYC/FX; no ccdB gene, camr | |
Recombinant DNA reagent (plasmid) | pCS19/X:T18/FX | PMID:37380079 | FX cloning vector; two SapI sites and ccdB gene for FX cloning; ampr | Two-hybrid assay vector; expressing a target protein ‘X’ with a C-terminal T18 fusion |
Recombinant DNA reagent (plasmid) | pCS19/T18 | PMID:37380079 | Expression plasmid from pCS19/X:T18/FX for expressing T18 fragment only; no ccdB gene. | Two-hybrid assay plasmid; control vector |
Recombinant DNA reagent (plasmid) | pACYC/T25 | PMID:37380079 | Expression plasmid from pACYC/T25:X/FX for expressing T25 fragment; no ccdB gene, camr | Two-hybrid assay plasmid; control vector |
Recombinant DNA reagent (plasmid) | pACYC/T25:MelBSt | PMID:37380079 | Expression plasmid derived from pACYC/T25:X/FX; no ccdB gene, camr | Two-hybrid assay plasmid; expressing T25:MelBSt hybrid |
Recombinant DNA reagent (plasmid) | pCS19/Nb725:T18 | PMID:37380079 | Expression plasmid hybrid derived from pCS19/X:T18/FX; no ccdB gene, ampr | Two-hybrid assay plasmid; expressing Nb725:T18 hybrid |
Recombinant DNA reagent (plasmid) | pCS19/Nb725_4:T18 | This study | Expression plasmid derived from pCS19/X:T18/FX; no ccdB gene, ampr | Two-hybrid assay plasmid; expressing Nb725_4:T18 hybrid |
Recombinant DNA reagent (plasmid) | pK95ΔAH/MelBSt/CHis10 | PMC3057838 | Constitutive expression plasmid | MelBSt protein expression |
Recombinant DNA reagent (plasmid) | pCS19/Nb725 | PMID:37380079 | Expression plasmid for Nb725 derived from pCS19/FX; no ccdB gene, ampr | Nb725 protein expression |
Recombinant DNA reagent (plasmid) | pCS19/Nb725_4 | This study | Expression plasmid for Nb725_4 derived from pCS19/FX; no ccdB gene, ampr | Nb725_4 protein expression |
Recombinant DNA reagent (plasmid) | pET26b(+) | Novagen (EMD Millipore) | Periplasmic expression plasmid with a N-terminal pelB signal sequence; Kanr | For expression Nb725_4 and anti-Fab Nb |
Recombinant DNA reagent (plasmid) | pET26/Nb725_4 | This study | Periplasmic expression plasmid derived from pET26b(+); Kanr | Nb725_4 protein production |
Recombinant DNA reagent (plasmid) | pET26/Anti-Fab Nb | This study | Anti-Fab Nb periplasmic expression plasmid; Kanr | Anti-Fab Nb protein expression |
Recombinant DNA reagent (plasmid) | p7XC3H/Nb725 | PMID:37380079 | Cytoplasmic expression plasmid; Kanr | Nb725 protein production |
Recombinant DNA reagent (plasmid) | p7XNH3/EIIAGlc | PMID:25296751 | Cytoplasmic expression plasmid; Kanr | EIIAGlc protein production |
Recombinant DNA reagent (plasmid) | pR2.2/NabFab | PMID:34782475 | Periplasmic expression of NabFab; Ampr | NabFab protein production |
Recombinant DNA reagent (plasmid) | pMSP1E3D1 | Addgene/20066 | Expressing SP1E3D1; Kanr | MSP1E3D1 production |
Recombinant DNA reagent (plasmid) | pRK792 | Addgene/8830 | Expressing TEV protease; Ampr | TEV protease production |
Sequence-based reagent (primers) | MelB_Nb725_4_T18 | This study | Fwd: 5′- ATATATGCTCTTCTAGTCAACGTCAATTGGTAG -3′ Rev: 5′- TATATAGCTCTTCATGCGCTGCTCACGGTCAC -3′ | FX cloning primers to contrast pCS19/Nb725_4:T18; addition of a C-terminal ‘A’ of Nb725_4 enabling the C-terminal T18 in-frame fusion |
Sequence-based reagent (primer) | MelB_Nb725_4 | This study | Fwd: 5′-ATATATGCTCTTCTAGTATGCAACGTCAATTGGTAG-3′ Rev: 5′-TATATAGCTCTTCATGCTTAGTGGTGATGATGGTGGTGGCT GCTCACGGTCAC-3′ | FX cloning primers to construct pCS19:Nb725_4-CTH with a C-terminal 6x His-tag |
Sequence-based reagent (primer) | Nb-PelB-NdeI | This study | Fwd: 5′-TTTAAGAAGGAGATATACATATG-3′ | Insert NdeI restriction site for Nb725_4 and anti-Fab Nb construction |
Sequence-based reagent (primer) | Nb-STRP-XhoI | This study | Rev: 5′-TTTGTTCTAGACTCGAGTTATTTCTC-3′ | Add XhoI restriction site for Nb725_4 and anti-Fab Nb construction |
Chemical compound, drug | [3H]Melibiose | PerkinElmer | (5.32 Ci/mmol) | Transport assay S |
Chemical compound, drug | UDM | Anatrace | Cat# D300HA | MelBSt purification |
Chemical compound, drug | DDM | Anatrace | Cat# D310 | MelBSt purification |
Chemical compound, drug | Melibiose | Acros Organics | Cat# 125375000 | Fermentation and Binding assay |
Chemical compound, drug | α-NPG | Acros Organics | Cat# 33733500 | Binding assay |
Chemical compound, drug | E. coli lipids | Avanti Polar Lipids, Inc | Extract Polar, 100600 | Nanodiscs |
Software, algorithm | CryoSPARC | CryoSPARC | 2-4.01 | CryoEM data processing |
Software, algorithm | USF ChimeraX | USF ChimeraX | 1.6 | Mask preparation |
Software, algorithm | Phenix | Phenix | 1.20-4459 | Map sharpen and model refinement |
Software, algorithm | Coot | Coot | 0.9 | Model building |
Software, algorithm | Pymol | Pymol | 2.5 | Model visualization |
Software, algorithm | Qscore | Qscore | Q score calculation | |
Software, algorithm | NanoAnalyze | TA Instruments | 3.7.5 | Data fitting |
Software, algorithm | HDExaminer | Trajan Scientific and Medical | 3.3 | HDX data analysis |
Software, algorithm | BioPharma Finder | Thermo | 5.1 | HDX data analysis and peptide mapping |
Reagents
MacConkey agar media (lactose-free) was purchased from Difco. Unlabeled melibiose and 4-nitrophenyl-α-d-galactopyranoside (α-NPG) were purchased from Sigma-Aldrich. Maltose was purchased from (Acros Organics, Fisher Scientific). [1-3H]Melibiose (5.32 Ci/mmol) was custom synthesized by PerkinElmer. Detergents undecyl-β-d-maltopyranoside (UDM) and n-dodecyl-β-d-maltopyranoside (DDM) were purchased from Anatrace. E. coli lipids (Extract Polar, 100600) were purchased from Avanti Polar Lipids, Inc,. Oligodeoxynucleotides were synthesized by Integrated DNA Technologies. LC-MS grade water, LC-MS 0.1% formic, LC-MS grade acetonitrile with 0.1% formic acid in water were purchased from Fisher Scientific (Hampton, NH). Guanidine hydrochloride, citric acid, and zirconium (IV) oxide 5 μm powder were purchased from Sigma-Aldrich (St. Louis, MO). Deuterium oxide (99+% D) was purchased from Cambridge Isotope Laboratories (Tewksbury, MA). All other materials were reagent grade and obtained from commercial sources.
Strains, plasmids, and primers
Request a detailed protocolThe genotype and source of E. coli strains used in this study are described in Key Resources Table unless otherwise described specifically. The commercial E. coli Stellar or XL1 Blue were used for plasmid construction. The E. coli DB 3.1 strain was used to construct the ccdB-containing universal FX Cloning vectors (Geertsma and Dutzler, 2011). E. coli DW2 (melB-lacY-) (Botfield and Wilson, 1988) was used for functional studies, and DH5α cyaA- strain was used for in vivo protein–protein interaction assay (Katsube et al., 2023). E. coli BL21 (DE3) strain and E. coli ArcticExpress (DE3) strain were used for overexpression Nbs; E. coli BL21 (DE3) C43 strain (Miroux and Walker, 1996) was used for overexpression NabFab; E. coli BL21(DE3) T7 express strain was used to express EIIAGlc (Hariharan and Guan, 2014). The plasmids used or created and primers in this study are also listed in the Key Resources Table.
CDR grafting
Request a detailed protocolCDR grafting technique (Riechmann et al., 1988) to transfer the binding specificity of MelBSt Nbs to the TC-Nb4, which can be recognized by the NabFab, was performed (Bloch et al., 2021; Figure 1—figure supplement 1). The DNA fragment, which carried the hybrid Nb725_4 along with N-terminal pelB signal peptide (MKYLLPTAAAGLLLLAAQPAMA) and C-terminal HRV-3C protease site (LEVLFQGP) and 8xHis-tag, was synthesized, digested using restriction enzymes NdeI and XhaI, and ligated with the corresponding sites on expression plasmid pET26b+, resulting in the pET26/Nb725_4.
MelBSt protein expression and purification
Request a detailed protocolThe MelBSt expression plasmid pK95ΔAH/MelBSt/CHis10 (Guan et al., 2011) was constructed by cloning melB from Salmonella typhimurium LT2 strain (Mizushima et al., 1992) for constitutive expression in E. coli (Pourcher et al., 1995). A 10 L fermenter was used for the constitutive expression of MelBSt in DW2 cells in Luria-Bertani (LB) broth supplemented with 50 mM KPi, pH 7.0, 45 mM (NH4)SO4, 0.5% glycerol, and 100 mg/L ampicillin (Ethayathulla et al., 2014). The cultures were grown at 30°C until A280 ~5. The procedures for membrane preparation, extraction with 1.5% UDM or 2% DDM, and cobalt-affinity purifications were performed as previously described (Ethayathulla et al., 2014; Hariharan and Guan, 2017; Katsube et al., 2023). The eluted MelBSt proteins were dialyzed overnight against a buffer of 20 mM Tris–HCl, pH 7.5, 100 mM NaCl, 10% glycerol, and 0.35% UDM or 0.01% DDM, and concentrated with Vivaspin 50 kDa MWCO membrane to approximately 50 mg/mL, stored at –80°C after flash-frozen with liquid nitrogen.
Overexpression and purification of membrane scaffold protein 1E3D1 (MSP1E3D1)
Request a detailed protocolAn expression plasmid pMSP1E3D1 (Addgene; plasmid 20066) was used for the overexpression of the MSP1D1E3 with a 7-His-tag and a TEV protease site in the E. coli BL21 (DE3) (Denisov et al., 2007; Denisov and Sligar, 2017). The culture was grown in LB media containing 0.5% glucose at 37°C, induced by adding 1 mM IPTG at A600 of about 0.6, and incubated for another 2.5 hr. The MSP proteins from the cell lysate were purified with INDIGO Ni-Agarose (Cube Biotech) using a protocol as described (Hariharan and Guan, 2021; Zoghbi et al., 2016). The eluted proteins were dialyzed against 20 mM Tris–HCl, pH 7.5, and 100 mM NaCl and concentrated to ~8 mg/mL. The His-tag of MSP1E3D1 was removed by a His-tagged TEV protease (at 1:20 mole/mole ratio of TEV:MSP1E3D1) in the same buffer. The His-tagged TEV protease, unprocessed His-tagged MSP1E3D1, and free His tag fragments were trapped within the Ni-Agarose column. The processed MSP1E3D1 proteins without a His tag in the flow-through were concentrated to about 6–8 mg/ml, and stored at –80°C after flash-frozen with liquid nitrogen.
TEV protease expression and purification
Request a detailed protocolThe tobacco etch virus (TEV) encoded by the plasmid pRK792 (Kapust et al., 2001) was overexpressed in a tRNA-supplemented BL21-CodonPlus (DE3)-RIPL strain. The cells were grown in LB broth containing 100 µg/mL ampicillin and 30 µg/mL chloramphenicol at 30°C until A600 of 0.8~1.0. At this point, the temperature was decreased to 18°C, and protein expression was induced by 0.5 mM IPTG overnight. The cells in 50 mM KPi, pH 8.0, 500 mM NaCl, and 10% glycerol were lysed by a microfluidizer and the supernatants after removing the cell debris were subjected to affinity chromatography using Talon resin. The eluted proteins were concentrated to ~3 mg/mL and quickly dialyzed against 20 mM Tris–HCl, pH 7.5, 200 mM NaCl, and 10% glycerol for 3–4 hr, and stored at –80°C after flash-frozen with liquid nitrogen. A typical yield can reach >30 mg/L.
MelBSt reconstitution into lipids nanodiscs
View detailed protocolThe MelBSt reconstitution was performed as described previously (Hariharan and Guan, 2021). Briefly, 5.6 mg of E. coli polar lipids extract at a concentration of 40 mg/mL solubilized in 7.5% DDM was added to 1 mL of 1 mg of MelBSt in 20 mM Tris–HCl, pH 7.5, 100 mM NaCl, 10% glycerol, and 0.35% UDM (~1:350 mole/mole), and incubated for 10 min on ice. MSP1E3D1 proteins were then added into the MelBSt/lipid mixture at a 5:1 mole ratio (MSP1E3D1:MelBSt), and incubated at room temperature with mild stirring for 30 min before being shifted to 4°C with mild stirring. The detergents were removed and subsequent MelBSt lipids nanodiscs were formed by stepwise additions of Bio-beads SM-2 resin (500 mg and then an additional 300 mg after 2 hr) and further incubated overnight. After separating the Bio-beads SM-2 resins, the MelBSt lipids nanodiscs were isolated by absorbing onto Ni-NTA beads and the MelBSt-free empty nanodiscs were in the void due to His-tag being removed. The MelBSt lipids nanodiscs were eluted by adding 250 mM imidazole and the elutes were dialyzed against 20 mM Tris–HCl, pH 7.5, and 150 mM NaCl, and concentrated to ~3–4 mg/mL. After ultracentrifugation at ~352,000 × g (90,000 rpm using a TLA-120 rotor) in a Beckman Optima MAX Ultracentrifuge for 30 min at 4°C, the supernatant containing MelBSt lipids nanodiscs were stored at –80°C after flash-frozen with liquid nitrogen.
Nbs expression and purification
Request a detailed protocolE. coli BL21(DE3) Arctic express cells were used to produce Nbs. The Nb725_4 and anti-Fab Nb were expressed by the expression plasmid pET26/Nb725_4 and pET26/anti-Fab Nb, respectively, containing the pelB leader sequence. Nb725 was expressed by the plasmid p7xC3H/Nb725 as described (Katsube et al., 2023). Cells were grown in Terrific Broth media supplemented with 0.4% (vol/vol) glycerol at 37°C. Protein expression was induced by adding 1 mM IPTG at the mid-log phase after decreasing the temperature to 25°C, and the cultures continued growth for 18–20 hr. The periplasmic extraction of Nbs using sucrose-mediated osmolysis was as described (Kariuki and Magez, 2021), and Nb purification was performed by cobalt-affinity chromatography, which yielded ~5 mg/L with >95% purity. The cytoplasmic expression for the Nb725 also provided a similar yield and purity, and all were stable. The purified Nbs were concentrated to ~5–10 mg/mL, dialyzed against 20 mM Tris–HCl, pH 7.5, and 150 mM NaCl, and frozen in liquid nitrogen and stored at –80°C.
NabFab expression and purification
Request a detailed protocolThe NabFab expressing plasmid pRH2.2/NabFab and the expression protocol were kindly gifted from Dr. Kasper P. Locher (Bloch et al., 2021; Hornsby et al., 2015). Briefly, the NabFab was expressed in E. coli C43 (DE3) cells in an autoinduction medium at 25°C for ~18 hr. The cells were resuspended in 20 mM Tris–HCl, pH 7.5, 500 mM NaCl, and 1 mM EDTA, and lysed by a microfluidizer. The lysates were subjected to heat treatment at 65°C for 30 min, followed by centrifugation at 33,175 × g (15,000 rpm on the rotor JA18) for 30 min at 4°C to remove insoluble materials. The supernatants were loaded onto the 5 mL Cytivia Protein L column for affinity purification of NabFab proteins. The eluted NabFab in 0.1 M acetic acid, pH 2.0, was neutralized with 200 mM Tris–HCl, pH 8.5, dialyzed against 20 mM Tris–HCl, pH 7.5, 150 mM NaCl, and concentrated up to 5 mg/mg prior to being frozen in liquid nitrogen and stored at –80°C. The yield for NabFab protein is about 2–3 mg/L.
EIIAGlc expression and purification
Request a detailed protocolThe overexpression of unphosphorylated EIIAGlc was performed in the E. coli T7 Express strain (New England Biolabs) as described (Hariharan and Guan, 2014).
A bacterial two-hybrid assay
Request a detailed protocolA bacterial two-hybrid assay based on reconstituting adenylate cyclase activities from the T18/T25 fragments of Bordetella pertussis adenylate cyclase toxin as described (Battesti and Bouveret, 2012; Ladant and Ullmann, 1999) was used to examine in vivo interaction of Nb and MelBSt in E. coli DH5α cyaA strain (Katsube et al., 2023). The competent cells were transformed by two compatible plasmids (pACYC [Bibi and Kaback, 1990] and pCS19 [Spiess et al., 1999]) with different replication origins (e.g., pACYC/T25:MelBSt vs. pCS19/Nb725:T18 [Katsube et al., 2023] or pCS19/Nb725_4:T18), and plated onto the lactose-free MacConkey agar plate containing 30 mM maltose as the sole carbohydrate source, 100 mg/L ampicillin, 25 mg/L chloramphenicol, and 0.2 mM IPTG, and the plates were incubated in 30℃ for 7 d before photography. Red colonies grown on the MacConkey agar indicated positive fermentation due to the cAMP production derived from the interaction of two hybrids. Yellow colonies denoted no fermentation and no cAMP production.
Sugar fermentation assay
Request a detailed protocolE. coli DW2 cells (ΔmelBΔlacYZ) were transformed with two compatible plasmids with different replication origins (pACYC/MelBSt and pCS19/Nbs) (Tikhonova et al., 2015) and plated onto the lactose-free MacConkey agar plate containing 30 mM maltose or melibiose as the sole carbohydrate source, 100 mg/L ampicillin, 25 mg/L chloramphenicol, and 0.2 mM IPTG. The plates were incubated at 37℃ for 18 hr before photography. Magenta colonies, a normal sugar fermentation; yellow colonies, no sugar fermentation. The cells carrying two empty plasmids or with MelBSt but no Nb were used as the control. Maltose fermentation was another control for the specificity of Nb inhibition.
[3H]Melibiose transport assay and western blot
Request a detailed protocolE. coli DW2 cells (melA+, melB-, lacZ-Y-) (Pourcher et al., 1995) were transformed with the two compatible plasmids pACYC/MelBSt and pCS19/Nb725 or pCS19/Nb725_4, respectively. The cells transformed with two empty vectors (pACYC and pCS19) or pACYC/MelBSt with pCS19 without an Nb were used as the negative or positive control, respectively. The cells were grown in LB broth with 100 mg/L ampicillin and 25 mg/L chloramphenicol in a 37°C shaker overnight, and inoculated by 5% to fresh LB broth with 0.5% glycerol, 100 mg/L ampicillin, 25 mg/L chloramphenicol, and 0.2 mM IPTG. The cultures were shaken at 30°C for 5 hr. The transport assay was performed at 20 mM Na+ and 0.4 mM [3H]melibiose (specific activity of 10 mCi/mmol) in 100 mM KPi, pH 7.5, 10 mM MgSO4 at A420 of 10 (~0.7 mg proteins/mL) as described (Guan et al., 2011; Jakkula and Guan, 2012). The transport time courses were carried out at 0, 5 s, 10 s, 30 s, 1 min, 2 min, 5 min, 10 min, and 30 min by dilution and fast filtration. The filters with trapped cells were subjected to radioactivity measurements using a Liquid Scintillation Counter. The MelBSt membrane expression was analyzed by western blot using HisProbe-HRP Conjugate as described (Katsube et al., 2023). The western blot result was imaged by the ChemiDoc MP Imaging System (Bio-Rad).
Isothermal titration calorimetry
Request a detailed protocolAll ITC ligand-binding assays were performed with the Nano-ITC device (TA Instruments) (Hariharan and Guan, 2017) and the heat releases are plotted as positive peaks. All samples were buffer-matched by simultaneously dialyzed in the same buffer or diluting the concentrated small molecule ligands to corresponding dialysis buffers as described (Hariharan and Guan, 2017; Hariharan and Guan, 2014). The titrand (MelBSt) placed in the ITC Sample Cell was titrated with the specified titrant (placed in the Syringe) by an incremental injection of 1.5–2 μL aliquots at an interval of 300 s at a constant stirring rate of 250 rpm. All samples were degassed by a TA Instruments Degassing Station (model 6326) for 15 min prior to the titration. Heat changes were collected at 25°C, and data processing was performed with the NanoAnalyze (v. 3.7.5 software) provided with the instrument. The normalized heat changes were subtracted from the heat of dilution elicited by the last few injections, where no further binding occurred, and the corrected heat changes were plotted against the mole ratio of titrant versus titrand. The values for the binding association constant (Ka) were obtained by fitting the data using the one-site independent-binding model included in the NanoAnalyze software (version 3.7.5), and the dissociation constant (Kd) = 1/Ka. In most cases, the binding stoichiometry (N) number was fixed to 1.
Protein concentration assay
Request a detailed protocolThe Micro BCA Protein Assay (Pierce Biotechnology, Inc) was used to determine the protein concentration assay. The A280 was used for concentration estimation.
Protein complex preparation for grid vitrification
Request a detailed protocolProteins NabFab, Nb725_4, and anti-Fab Nb were mixed at a mole ratio of 1:1.1:1.15. The final complex was obtained by adding WT MelBSt in nanodiscs based on a mole ratio of 1:1.6 of MelBSt:NabFab complex. The sample was expected to contain 6 μM MelBSt, 12 μM MPS1E3D1, 9.5 μM NabFab complex in 20 mM Tris–HCl, pH 7.5, 150 mM NaCl (~1.5 mg/mL). The complex was also analyzed by gel-filtration chromatography using GE Superdex 200 column on a Bio-Rad NGC Chromatography System. Peak fractions were examined by SDS-15% PAGE stained by silver nitrate.
Grids vitrification and single-particle cryoEM data acquisition
Request a detailed protocolGrids preparation and data collection were carried out in S2C2 (Menlo Park, CA). The WT MelBSt/Nb725_4/NabFab/anti-NabFab Nb complex was thawed out from –80°C storage. Aliquots of 3 μL of samples at 0.75 mg/mL or 1.5 mg/mL protein concentration were placed on 300-mesh Cu holey carbon grids (Quantifoil, R1.2/1.3) after glow-discharge (PELCO easiGlow) at 15 mA for 30 s and blotted on two sides for 3 s at 4°C and 100% humidity before vitrified in liquid ethane using a Vitrobot Mark IV (Thermo Fisher). The grids were subsequently transferred to a Titan Krios (G3i) electron microscope (Thermo Fisher) operating at 300 kV and equipped with K3 direct electron detector (Gatan) and BioQuantum energy filter. The best grids were found from the sample at 1.5 mg/mL concentration.
A total of 14,094 and 8715 movies were automatically collected using the EPU Data Acquisition Software (Thermo Fisher) for non-tilted and 30° tilted collections, respectively. Both datasets were collected at a 0.86 Å pixel size and a dose of 50 e−/Å−2 across 40 frames (0.0535 or 0.07025 s per frame for the non-tilted and tilted data collection, respectively) at a dose rate of approximately 23.36 or 17.8 e−/Å2/s, respectively. A set of defocus values was applied ranging from −0.8, to 1.0, −1.2,–1.4, or –1.8 μm with an energy filter slit width of 20 eV and a 100 μm objective aperture.
Single-particle data processing
Request a detailed protocolAll cryoEM single-particle data processing was performed using CryoSPARC program (v. 2–4.01) (Figure 2—figure supplement 1). All imported movies were aligned using defaulted Patch Motion Correction and followed with defaulted Patch contrast transfer function (CTF) Estimation. Micrographs were then curated based on CFT Estimation, astigmatism, and ice thickness, which resulted in 13,649 and 7129 micrographs for the non-tilted and 30° tilted datasets, respectively.
Initially, particle picking from the 2000 micrographs was performed using Blob Picker using 120 and 160 Å for the minimal and maximal particle diameters, respectively, in the CryoSPARC program (Figure 2—figure supplement 2). A total of 1,087,124 particles were selected and extracted with a box size of 256 pixels. After two runs of 2D Classification, five classes were selected as imputing templates in Template Picker. A total of resultant 9,103,229 particles from 13,649 micrographs were extracted with a box size of 384 pixels bin 92 pixels and subjected to iterative rounds of 2D Classification. The yielded 1,245,734 particles were re-extracted with a box size of 416 pixels. After further sorting using 2D Classification, the highly selected 338,883 particles, which clearly contained nanodisc-surrounding MelBSt bound with Nb725_4/NabFab/anti-NabFab Nb, were used for Ab Initio 3D Reconstruction. One out of five Ab Initio classes contained the expected super-complex from a total of 93,693 particles, which allowed for a 3.80 Å volume to be reconstructed using Non-uniform Refinement. The 93,693 particles were then further sorted using heterogeneous refinement with input volumes created by multiclass ab initio to a subset of 41,264 particles, which allowed a 3.49 Å volume to be reconstructed using Non-uniform Refinement with a static mask prepared from one of the volumes. This map was successfully used to build the initial model of all four proteins; however, this map was anisotropic and lacked some orientations in angular space. To improve the map quality, two strategies were applied including reprocessing this dataset and performing tilted data collection. The hand of the 3.49 Å volume was changed and used to generate 50 templates.
Template Pick using the 50 templated was applied to the 13,649 micrographs, which generated 7,632,727 particles (Figure 2—figure supplement 3). After extraction with a box size of 384 pixels bin 92 pixels and iterative rounds of 2D Classification and Heterogeneous Refinement, 370,941 particles were selected and re-extracted with a box size of 384 pixels. This 370,941 particle set was combined with the previously identified 41,264 particle set after extraction with a box size of 384 pixels and removed duplicate, the subset of 188,566 particles exhibited slightly improved directional distribution. To further explore the first data collection, the previously rejected 2D classes were analyzed and 214,522 particles were selected based on orientation. After combined and iterative rounds of 2D Classification, Heterogeneous Refinement, and Local Refinement, a subset of the 196,254 particle set supported a 3.39 Å volume to be reconstructed using local refinement, and the directional distribution of the map is further improved.
A tilted data collection on the same microscopy and parameter settings was performed with a 30° tilt (Figure 2—figure supplement 3). A total number of 7129 micrographs were obtained. The 50 templates were used for particle pick, which yielded 2,887,147 particles after curation. The particles were extracted using a box size of 384 bin 96 pixels. After three-run 2D Classification followed by three-run Heterogeneous Refinement using the 3.39 Å volumes and the other two ab initio volumes, a subset of 544,531 particles were re-extracted using a box size of 384 pixels. After further iterative rounds of 2D Classification, Heterogeneous Refinement, and Local Refinement, 182,101 particles were obtained for the tilted collection, which could support a 4.01 Å volume using Local Refinement and directional distribution focused on the missing angles in the first dataset.
A total number of 378,355 polished particles from both non-tilted and 30° tilted data acquisition were used to reconstruct a 3.40 Å volume using Local Refinement, and the 30° tilted data filled the missing particle orientations but the resolution was not improved. The particles were subjected to 3D Classifications using a target resolution of 3.3 Å and 10 classes and the highest resolution classes were collected. The final collected 296,925 particle set supported a 3.37 Å volume to be reconstructed using Local Refinement and a static mask covering MelBSt, Nb725_4, and NabFab. The reporting GSFSC resolution of 3.29 Å was calculated by Validation (FSC) using the two half maps, and the particle distribution was calculated by ThereDFSC (Figure 2—figure supplement 4).
The initial mask for Non-uniformed Refinement was created in UCSF ChimeraX using the volume generated from Ab Initio 3D Reconstruction which covered the full complex. The refinement masks for final-stage Local Refinement only covered MelBSt, Nb725_4, and NabFab and filtered at a low pass to 10 Å and soft padding width of 10. The 3D Classification used the full-covered mask as the solvent mask and the refinement masks in the Local Refinement as the focus mask.
Local Refinement set the initial low pass resolution of 8 Å, use of pose/shift Gaussian prior during alignment, rotation search extent of 9°, and shift search extent of 6 Å. The final 3.29 Å volume was sharpened in Phenex auto_sharpen program (Terwilliger et al., 2018) default setting for model refinement.
Model building and structure refinement
Request a detailed protocolThe initial model building was carried out based on the 3.45 Å reconstruction map obtained from the first dataset. The coordinates of MelBSt D59C (PDB ID 7L17), NabFab, and anti-NabFab Nb (PDB ID 7PHP), and a sequence-based 3-D model generated by AlphaFold 2 were fitted into the map in UCSF ChimeraX (Goddard et al., 2018). The N-terminal helices of MelBSt fit well and the C-terminal helices were manually fitted into the initial map. The sequence of MelBSt D59C was mutated back to wild type in COOT (Emsley and Cowtan, 2004). All four chains were changed to poly-Ala peptides and the side chains of each residue were rebuilt. Structure refinement was performed using phenix.real_space_refine application in PHENIX (Afonine et al., 2013) in real space with secondary structure and geometry restraints.
The model was refined against the final map at a resolution of 3.29 Å after sharpening using PHENIX auto_sharpen program default setting (Terwilliger et al., 2018). A cation Na+ was modeled in the density between helices II and IV in COOT. After runs of refinement and remodeling, in total, 417 residues of MelBSt (positions 2–210, 219–355, and 364–432), with 6 unassigned side-chains at the C-terminal domain (Leu293, Tyr355, Arg363, Tyr369, Tyr396, and Met410) due to the map disorder, 122 residues of Nb725_4 (2–123), 229 residues of NabFab H-chain (1–214), and 210 residues of NabFab L-chain (4–213), were modeled, respectively. There are three MelBSt regions with missing densities including the middle loop righter after the Nb binding site (positions 211–218), loop10-11 (356–361), and the C-terminal tail after residue Tyr432. In addition, the densities between positions 219–230 and the regions close to the missing densities at loop10-11 are very weak. There is no unexplained density that can be clearly modeled by lipids. Statistics of the map reconstruction and model refinement can be found in Table 3. The model-map fitting quality was evaluated by Q score program (Pintilie et al., 2020). Pymol (Schrodinger, 2013) and UCSF chimera or ChimeraX (Goddard et al., 2018) were used to prepare the figures.
All-atom molecular dynamics (MD) simulations
Request a detailed protocolAll-atom MD simulations were performed taking the inward-facing cryoEM structure as the starting model. Atom coordinates in the loops missing from the cryoEM structure were filled in using homology modeling with the Modeller software package (Webb and Sali, 2016). Subsequently, the protein was embedded into a lipid bilayer composed of 1-palmitoyl-2-oleoyl phosphatidylethanolamine (POPE), 1-palmitoyl-2-oleoyl phosphatidylglycerol (POPG), and cardiolipin (CDL), in a molar ratio of 7:2:1 for POPE:POPG:CDL, mimicking the membrane composition of E. coli. Each side of the lipid bilayer was then enclosed by a box of water molecules with 25 Å thickness. Sodium chloride ions were introduced among these water molecules to yield a solution with an approximate concentration of 0.16 M. The system setup was performed via the CHARMMGUI (Jo et al., 2008) web interface. The all-atom MD simulations for the outward-facing MelBSt under the same setup have been reported by constructing through an in silico mutation, converting the outward-facing crystal structure of the D59C MelBSt mutant (PDB ID 7L16) back to the WT MelBSt (Katsube et al., 2022). In both the inward- and outward-facing configurations, a sodium ion was initially situated in the binding site, while melibiose was notably absent from the system.
Each system underwent initial optimization where the heavy atoms of proteins and lipids were harmonically restrained, employing a force constant of 1000 kJ/mol/Å2 . This was followed by 100 ps of dynamics at a temperature of 300 K in the constant NVT ensemble. Subsequently, the restraints were gradually reduced during 10 ns of dynamics in the constant NPT ensemble, maintained at a temperature of 300 K and pressure of 1 atm. For equilibration, we carried out an additional 30 ns of dynamics in the NPT ensemble under the same conditions, but without any restraints. After the equilibration stage, 1 μs of dynamics was performed in the constant NPT ensemble, under the same temperature and pressure, to generate the production trajectories. We maintained the temperature using a Langevin thermostat with a friction coefficient of 1 ps–1. The trajectory was propagated with a timestep of 2 fs, and all bonds involving a hydrogen atom were constrained. The CHARMM36 force field (Klauda et al., 2010; Best et al., 2012; Guvench et al., 2009) was utilized to simulate the protein, lipid, and ions throughout all simulations. Electrostatic interactions were calculated using the particle mesh Ewald method, with a tolerance of 5 × 10–4. We set the cutoff for van der Waals interactions at 12 Å. The TIP3P model (Jorgensen et al., 1983) was used to treat water molecules and ions. All classical MD simulations were performed using the OPENMM (Eastman et al., 2013) software package.
Hydrogen-deuterium exchange coupled to mass spectrometry (HDX-MS)
Request a detailed protocolIn-solution pairwise HDX-MS experiment was performed to study the structural dynamics of MelBSt. Labeling, quenching, lipids removal, and online digestion were achieved using a fully automated manner using HDx3 extended parallel system (LEAP Technologies, Morrisville, NC) (Hamuro et al., 2003; Hamuro and Coales, 2018).
Working samples of MelBSt and MelBSt complexed with Nb725_4 were prepared to a concentration of 50.0 μM for MelBSt and 100 μM for Nb725_4 in a buffer of 25 mM Tris–HCl, pH 7.5, 150 mM NaCl, 10% glycerol, 0.01% DDM in H2O. Aliquots of 4 µl of each working sample were diluted by tenfold into the labeling buffer of 25 mM Tris–HCl, pD 7.5, 150 mM NaCl, 10% glycerol, 0.01% DDM in D2O. The reactions were incubated in D2O buffer at 20°C for multiple time points (30 s, 300 s, and 3000 s) in triplicates and non-deuterated controls were prepared in a similar manner except H2O buffer was used in the labeling step. The pH of the labeling buffer was measured with a pH meter mounted with a glass electrode and then corrected to pD (pD = pH + 0.4).
The reactions were quenched at given time points by adding the same volume of ice-cold 6 M urea, 100 mM citric acid, pH, 2.3 in water for 180 s at 0°C and immediately subjected to a lipid filtration module containing ZrO2 available on the LEAP PAL system. After incubation of 60 s, the LEAP X-Press then compressed the filter assembly to separate proteins from the ZrO2 particles-bound phospholipids and detergents. The filtered protein sample was injected into a cool box for online digestion and separation.
LC/MS bottom-up HDX was performed using a Thermo Scientific Ultimate 3000 UHPLC system and Thermo Scientific Orbitrap Eclipse Tribrid mass spectrometer. Samples were digested with a Nepenthesin-2 (Affipro, Czech Republic) column at 8°C for 180 s and then trapped in a 1.0 mm × 5.0 mm, 5.0 µm trap cartridge for desalting. Peptides were then separated on a Thermo Scientific Hypersil Gold, 50 × 1 mm, 1.9 µm, C18 column with a gradient of 10–40% gradient (A: water, 0.1% formic acid; B: acetonitrile, 0.1% formic acid) gradient for 15 min at a flow rate of 40 µL/min. To limit carry-over issues, a pepsin wash was added in between runs. To limit the back-exchange of hydrogen, all of the quenching, trapping, and separation steps were performed at near 0°C.
For data analysis, a nonspecific digested peptide database was created for MelBSt with separate tandem mass spectrometry measurements of non-deuterated samples. Digested peptides from undeuterated MelBSt protein were identified on the orbitrap mass spectrometer using the same LC gradient as the HDX-MS experiment with a combination of data-dependent and targeted HCD-MS2 acquisition. Using the Thermo BioPharma Finder software (v 5.1), MS2 spectra were matched to the MelBSt sequence with fixed modifications. Altogether 153 peptide assignments (confident HDX data cross all labeling times) were confirmed for MelBSt samples giving 86% sequence coverage. MS data files were processed using the Sierra Analytics HDExaminer software with the MelBSt peptide database. Following the automated HDX-MS analysis, manual curation was performed. Upon the completion of the data review, a single charge state with high-quality spectra for all replicates across all HDX labeling times was chosen to represent HDX for each peptide. Differential HDX data were tested for statistical significance using the hybrid significance testing criteria method with an in-house MATLAB script, where the HDX differences at different protein states were calculated (ΔD = DNb725_4-bound – DNb-free). D denotes deuteration, defined as the mass increase above the average mass of the undeuterated peptide. Mean HDX differences from the three replicates were assigned as significant according to the hybrid criteria based on the pooled standard deviation and Welch’s t-test with p<0.01. The statistically significant differences observed at each residue (i.e., ΔD ≥ 0.3184 for this study) were used to map HDX consensus effects based on overlapping peptides onto the structure models. Residue-level data analysis was performed using a built-in function in BioPharma Finder software. The parameters included the number of simulations of 200, recorded solutions of 20, Chi2 increase by the larger of smooth absolute of 0, smother relative of 2, differential absolute of 0, and differential relative of 2.
Data availability
CryoEM data have been deposited to wwPDB under the accession code 8T60 and EMDB ID EMD-41062. Source data has been provided for Figure 1, Figure 1—figure supplement 1, Figure 2—figure supplement 8, and Figures 5 and 6.
-
RCSB Protein Data BankID 8T60. Mobile barrier mechanisms for Na+-coupled symport in an MFS sugar transporter.
-
Electron Microscopy Data BankID EMD-41062. Mobile barrier mechanisms for Na+-coupled symport in an MFS sugar transporter.
References
-
Suppression of conformation-compromised mutants of Salmonella enterica serovar Typhimurium MelBJournal of Bacteriology 196:3134–3139.https://doi.org/10.1128/JB.01868-14
-
Optimization of the additive CHARMM all-atom protein force field targeting improved sampling of the backbone φ, ψ and side-chain χ(1) and χ(2) dihedral anglesJournal of Chemical Theory and Computation 8:3257–3273.https://doi.org/10.1021/ct300400x
-
Mutations that simultaneously alter both sugar and cation specificity in the melibiose carrier of Escherichia coliThe Journal of Biological Chemistry 263:12909–12915.
-
Improved prediction of protein-protein interactions using AlphaFold2Nature Communications 13:1265.https://doi.org/10.1038/s41467-022-28865-w
-
Cooperativity in cytochrome P450 3A4: linkages in substrate binding, spin state, uncoupling, and product formationThe Journal of Biological Chemistry 282:7066–7076.https://doi.org/10.1074/jbc.M609589200
-
Nanodiscs in membrane biochemistry and biophysicsChemical Reviews 117:4669–4713.https://doi.org/10.1021/acs.chemrev.6b00690
-
The bacterial phosphoenolpyruvate:carbohydrate phosphotransferase system: regulation by protein phosphorylation and phosphorylation-dependent protein-protein interactionsMicrobiology and Molecular Biology Reviews 78:231–256.https://doi.org/10.1128/MMBR.00001-14
-
The effect of modifications of the charged residues in the transmembrane helices on the transport activity of the melibiose carrier of Escherichia coliBiochemical and Biophysical Research Communications 285:348–354.https://doi.org/10.1006/bbrc.2001.5200
-
OpenMM 4: A Reusable, ExtensibleHardware Independent Library for High Performance Molecular Simulation. J. Chem. Theo. Comp 9:461–469.https://doi.org/10.1021/ct300857j
-
Coot: model-building tools for molecular graphicsActa Crystallographica. Section D, Biological Crystallography 60:2126–2132.https://doi.org/10.1107/S0907444904019158
-
Structure-based mechanism for Na(+)/melibiose symport by MelBNature Communications 5:3009.https://doi.org/10.1038/ncomms4009
-
Review: the molecular logic of sodium-coupled neurotransmitter transportersPhilosophical Transactions of the Royal Society of London. Series B, Biological Sciences 364:149–154.https://doi.org/10.1098/rstb.2008.0181
-
Lessons from lactose permeaseAnnual Review of Biophysics and Biomolecular Structure 35:67–91.https://doi.org/10.1146/annurev.biophys.35.040405.102005
-
Mechanism of melibiose/cation symport of the melibiose permease of Salmonella typhimuriumThe Journal of Biological Chemistry 286:6367–6374.https://doi.org/10.1074/jbc.M110.206227
-
Encyclopedia of Biophysics 2nd EdnNa(+)/Melibiose membrane transport protein, Melb, Encyclopedia of Biophysics 2nd Edn, Springer, 10.1007/978-3-642-35943-9.
-
BookGlucose/sugar transport in bacteriaIn: Jez J, editors. Encyclopedia of Biological Chemistry. Elsevier. pp. 192–202.https://doi.org/10.1016/B978-0-12-819460-7.00093-1
-
Structure and mechanism of membrane transportersScientific Reports 12:13248.https://doi.org/10.1038/s41598-022-17524-1
-
CHARMM Additive All-Atom Force Field for Glycosidic Linkages between HexopyranosesJournal of Chemical Theory and Computation 5:2353–2370.https://doi.org/10.1021/ct900242e
-
Rapid analysis of protein structure and dynamics by hydrogen/deuterium exchange mass spectrometryJournal of Biomolecular Techniques 14:171–182.
-
Optimization of Feasibility Stage for Hydrogen/Deuterium Exchange Mass SpectrometryJournal of the American Society for Mass Spectrometry 29:623–629.https://doi.org/10.1007/s13361-017-1860-3
-
Insights into the inhibitory mechanisms of the regulatory protein IIA(Glc) on melibiose permease activityThe Journal of Biological Chemistry 289:33012–33019.https://doi.org/10.1074/jbc.M114.609255
-
Thermodynamic cooperativity of cosubstrate binding and cation selectivity of Salmonella typhimurium MelBThe Journal of General Physiology 149:1029–1039.https://doi.org/10.1085/jgp.201711788
-
Cooperative binding ensures the obligatory melibiose/Na+ cotransport in MelBThe Journal of General Physiology 153:e202012710.https://doi.org/10.1085/jgp.202012710
-
A High Through-put Platform for Recombinant Antibodies to Folded ProteinsMolecular & Cellular Proteomics 14:2833–2847.https://doi.org/10.1074/mcp.O115.052209
-
Reduced Na+ affinity increases turnover of Salmonella enterica serovar Typhimurium MelBJournal of Bacteriology 194:5538–5544.https://doi.org/10.1128/JB.01206-12
-
CHARMM-GUI: A web-based graphical user interface for CHARMMJournal of Computational Chemistry 29:1859–1865.https://doi.org/10.1002/jcc.20945
-
Comparison of simple potential functions for simulating liquid waterThe Journal of Chemical Physics 79:926–935.https://doi.org/10.1063/1.445869
-
Tobacco etch virus protease: mechanism of autolysis and rational design of stable mutants with wild-type catalytic proficiencyProtein Engineering, Design and Selection 14:993–1000.https://doi.org/10.1093/protein/14.12.993
-
Improving the yield of recalcitrant Nanobodies by simple modifications to the standard protocolProtein Expression and Purification 185:105906.https://doi.org/10.1016/j.pep.2021.105906
-
Molecular Basis for the Cation Selectivity of Salmonella typhimurium Melibiose PermeaseJournal of Molecular Biology 434:167598.https://doi.org/10.1016/j.jmb.2022.167598
-
In vivo and in vitro characterizations of melibiose permease (MelB) conformation-dependent nanobodies reveal sugar-binding mechanismsThe Journal of Biological Chemistry 299:104967.https://doi.org/10.1016/j.jbc.2023.104967
-
Update of the CHARMM all-atom additive force field for lipids: validation on six lipid typesThe Journal of Physical Chemistry. B 114:7830–7843.https://doi.org/10.1021/jp101759q
-
Resistance of the melibiose carrier to inhibition by the phosphotransferase system due to substitutions of amino acid residues in the carrier of Salmonella typhimuriumThe Journal of Biological Chemistry 267:18336–18341.
-
Bordatella pertussis adenylate cyclase: a toxin with multiple talentsTrends in Microbiology 7:172–176.https://doi.org/10.1016/s0966-842x(99)01468-7
-
SLC transporters as therapeutic targets: emerging opportunitiesNature Reviews. Drug Discovery 14:543–560.https://doi.org/10.1038/nrd4626
-
Complete cysteine-scanning mutagenesis of the Salmonella typhimurium melibiose permeaseThe Journal of Biological Chemistry 297:101090.https://doi.org/10.1016/j.jbc.2021.101090
-
The inner interhelix loop 4-5 of the melibiose permease from Escherichia coli takes part in conformational changes after sugar bindingThe Journal of Biological Chemistry 281:25882–25892.https://doi.org/10.1074/jbc.M601259200
-
Osmochemistry of solute translocationResearch in Microbiology 141:286–289.https://doi.org/10.1016/0923-2508(90)90002-8
-
Cloning and sequencing of the melB gene encoding the melibiose permease of Salmonella typhimurium LT2Molecular & General Genetics 234:74–80.https://doi.org/10.1007/BF00272347
-
Phosphoenolpyruvate:carbohydrate phosphotransferase systems of bacteriaMicrobiological Reviews 57:543–594.https://doi.org/10.1128/mr.57.3.543-594.1993
-
Mutagenesis of acidic residues in putative membrane-spanning segments of the melibiose permease of Escherichia coli. I. Effect on Na(+)-dependent transport and binding propertiesThe Journal of Biological Chemistry 268:3209–3215.
-
Automated map sharpening by maximization of detail and connectivityActa Crystallographica Section D Structural Biology 74:545–559.https://doi.org/10.1107/S2059798318004655
-
BookComparative protein structure modeling using ModellerIn: Webb B, editors. Current Protocols in Protein Science. Wiley. pp. 1–2.https://doi.org/10.1002/cpps.20
-
Structural Biology of the Major Facilitator Superfamily TransportersAnnual Review of Biophysics 44:257–283.https://doi.org/10.1146/annurev-biophys-060414-033901
-
Effect on cationic selectivity and coupling propertiesThe Journal of Biological Chemistry 268:3216–3221.https://doi.org/10.1016/S0021-9258(18)53680-2
-
Protein dynamics and conformational changes explored by hydrogen/deuterium exchange mass spectrometryCurrent Opinion in Structural Biology 58:305–313.https://doi.org/10.1016/j.sbi.2019.06.007
-
The Lipid Bilayer Modulates the Structure and Function of an ATP-binding Cassette ExporterThe Journal of Biological Chemistry 291:4453–4461.https://doi.org/10.1074/jbc.M115.698498
Article and author information
Author details
Funding
National Institute of General Medical Sciences (R01 GM122759)
- Lan Guan
Welch Foundation (D-2108-20220331)
- Ruibin Liang
The funders had no role in study design, data collection and interpretation, or the decision to submit the work for publication.
Acknowledgements
We thank the S2C2 cryoEM facility sponsored by NIH Common Fund Transformative High-Resolution Cryo-Electron Microscopy Program (U24 GM129541 to Wah Chiu). We thank Drs. Wah Chiu, Htet Khant, and Grigore Pintilie for support and critical discussions; Drs. Kaspar P Locher, Anthony A Kossiakoff, Joël S Bloch, and Somnath Mukherjee for kindly providing the NabFab plasmid and detailed instructions; Drs. Eric R Geertsma and Raimund Dutzler for the FX cloning tools; Dr. Gerard Leblanc for a MelB-expressing vector and DW2 strain; Drs. Bryan Sutton and Kei Nanatani for support; and Dr. Michael Wiener for critical reading and editing. This work was supported by the National Institutes of Health Grant R01 GM122759 to LG and the Welch Foundation D-2108-20220331 to RL.
Version history
- Sent for peer review:
- Preprint posted:
- Reviewed Preprint version 1:
- Reviewed Preprint version 2:
- Version of Record published:
Cite all versions
You can cite all versions using the DOI https://doi.org/10.7554/eLife.92462. This DOI represents all versions, and will always resolve to the latest one.
Copyright
© 2023, Hariharan et al.
This article is distributed under the terms of the Creative Commons Attribution License, which permits unrestricted use and redistribution provided that the original author and source are credited.
Metrics
-
- 1,085
- views
-
- 143
- downloads
-
- 10
- citations
Views, downloads and citations are aggregated across all versions of this paper published by eLife.
Citations by DOI
-
- 3
- citations for umbrella DOI https://doi.org/10.7554/eLife.92462
-
- 7
- citations for Version of Record https://doi.org/10.7554/eLife.92462.3