CatSperζ regulates the structural continuity of sperm Ca2+ signaling domains and is required for normal fertility
Abstract
We report that the Gm7068 (CatSpere) and Tex40 (CatSperz) genes encode novel subunits of a 9-subunit CatSper ion channel complex. Targeted disruption of CatSperz reduces CatSper current and sperm rheotactic efficiency in mice, resulting in severe male subfertility. Normally distributed in linear quadrilateral nanodomains along the flagellum, the complex lacking CatSperζ is disrupted at ~0.8 μm intervals along the flagellum. This disruption renders the proximal flagellum inflexible and alters the 3D flagellar envelope, thus preventing sperm from reorienting against fluid flow in vitro and efficiently migrating in vivo. Ejaculated CatSperz-null sperm cells retrieved from the mated female uterus partially rescue in vitro fertilization (IVF) that failed with epididymal spermatozoa alone. Human CatSperε is quadrilaterally arranged along the flagella, similar to the CatSper complex in mouse sperm. We speculate that the newly identified CatSperζ subunit is a late evolutionary adaptation to maximize fertilization inside the mammalian female reproductive tract.
https://doi.org/10.7554/eLife.23082.001eLife digest
Male mammals ejaculate millions of sperm cells each time they mate with a female. Only a few of these cells manage to travel up the female’s reproductive tract to reach the egg, and usually only one sperm fertilizes it. Freshly ejaculated sperm are incapable of fertilizing eggs and have to undergo several changes within the female to become able to do so. One crucial change occurs in the sperm tail, which starts to beat vigorously in a whip-like motion. This type of movement – known as hyperactivated motility – enables the sperm to swim towards the egg, push through a sticky coating that surrounds it, and then burrow into it.
Hyperactivated motility is triggered when calcium ions enter the sperm cell via a specific channel protein known as CatSper, which is found in the membrane that surrounds the cell. CatSper channels form groups (known as complexes) with several other proteins that are arranged in a unique pattern of four straight ‘stripes’ running down the tail of the sperm. This arrangement is necessary for hyperactivated motility and mutations in the genes that encode these proteins can lead to infertility in males. The CatSper channel complex is known to contain seven proteins: four that form a pore through which calcium ions can enter, and three accessory proteins whose roles in hyperactivated motility are less clear.
Chung et al. identified two genes in mice that encode new accessory proteins in the CatSper channel complex named CatSper epsilon and CatSper zeta. Further experiments analyzed the role of CatSper zeta in more detail. Mutant males that lack CatSper zeta have fragmented patterns of CatSper stripes in the tails of their sperm. Moreover, fewer calcium ions were able to pass through the channels to enter the cell. Together, this made the sperm tail more rigid, which prevented it from moving efficiently within the female, resulting in reduced fertility. Chung et al. also found that the mutant sperm were less able to penetrate the egg than normal sperm.
During evolution, the gene that encodes CatSper zeta appeared first in mammals and may represent an adaptation that improved the chances of a sperm fertilizing the egg inside the reproductive tract of female mammals. Future challenges will be to explore how the CatSper channel assembles on the membrane of sperm and find out exactly how calcium ions trigger hyperactivated motility.
https://doi.org/10.7554/eLife.23082.002Introduction
Sperm hyperactivation, characterized by a large asymmetric lateral displacement of the flagellum (Ishijima et al., 2002), is required for normal mammalian sperm navigation (Demott and Suarez, 1992), rheotaxis (Miki and Clapham, 2013), and zona pellucida (ZP) penetration (Stauss et al., 1995). Calcium influx through the flagellar Ca2+ ion channel, CatSper, triggers hyperactivation (Carlson et al., 2003; Kirichok et al., 2006; Ren et al., 2001) and leads to changes in the flagellar envelope during capacitation (Chung et al., 2011; Quill et al., 2003). In hyperactivated spermatozoa, the transverse flagellar force is larger than the propulsive flagellar force due to the increase in mid-piece curvature (α angle), which enables a larger range of motion and typical figure-of-eight swimming trajectories compared to the nearly straight paths of non-hyperactivated spermatozoa (Ishijima, 2011). Transverse force facilitates sperm penetration through the cumulus and ZP (Ishijima, 2011; Yanagimachi, 1966). Spermatozoa from all CatSper-null (1–4 or d) males have smaller α angles than wild-type (wt spermatozoa upon capacitation (Chung et al., 2011; Qi et al., 2007). Consistently, CatSper-null mutant spermatozoa migrate inefficiently in vivo (Chung et al., 2014; Ho et al., 2009) and fail to penetrate the ZP (Ren et al., 2001).
Sperm rheotax against Fallopian tubular and isthmus fluid flow (Miki and Clapham, 2013). Rheotactic turning to reorient to directional flow depends on flagellar rolling, not the sperm head or its geometry, as demonstrated by the rheotaxis of headless mouse sperm (Miki and Clapham, 2013). CatSper channels form unique Ca2+ signaling domains in linearly quadrilateral arrays along the principal piece of sperm flagella. The integrity of these domains is necessary to time and/or maintain hyperactivated motility (Chung et al., 2014). Thus, CatSper1-null sperm cannot rheotax due to defects in rolling (Miki and Clapham, 2013), and presumably exert less lateral force in escaping from epithelial walls (Ho et al., 2009) or in pushing cumulus cells aside. In general, however, there is a lack of understanding of the steps between CatSper-mediated calcium entry, Ca2+-modified phosphorylation cascades, and the resulting structural changes underlying orchestrated flagellar movement.
Here, we reveal that the murine Gm7068 (C1orf101-like) and Tex40 genes encode two new subunits of the CatSper ion channel complex, CatSper epsilon (ε) and zeta (ζ), respectively. In this study, we focus primarily on CatSperζ’s function. Genetic disruption of mammalian-specific CatSperζ reduces the CatSper current in the sperm flagellum and hyperactivated motility, resulting in severe subfertility. We use high speed video microscopy and digital image analysis to determine swimming trajectory and the flagellar waveform in detail. Surprisingly, abrogation of CatSperζ renders the proximal flagellum inflexible but preserves overall motility, thus resulting in restriction of the 3D flagellar envelope, inefficient sperm rheotaxis in vitro, and delayed sperm migration in vivo. Using super-resolution microscopy, we demonstrated that the structurally distinct CatSper Ca2+ signaling domains along the flagellum (Chung et al., 2014) becomes fragmented in the absence of CatSperz. We demonstrate that IVF failure of CatSperz-null spermatozoa is partially rescued by using ejaculated sperm recovered from the uterus of mated females, explaining the discrepancy between in vitro and in vivo fertilizing ability. Finally, we show that mouse and human spermatozoa have a similar macroscopic organization of the CatSper complex.
Results
CatSper ε and ζ: Two new accessory proteins in the CatSper channel complex
We previously identified seven protein components of the CatSper channel complex (CatSper1-4, β, γ, and δ) from mouse testis using tandem affinity purification (Chung et al., 2011). As the most biochemically complex ion channel known to date, it has not been possible to express functional CatSper channels in heterologous systems. This includes many attempts in many cell types, including simultaneous injection of all 7 CatSper mRNAs into Xenopus oocytes (data not shown). Therefore, we continued to seek potential additional components to more thoroughly understand CatSper channel assembly and trafficking. We identified a mouse homolog of human C1orf101 (C1orf101-like, currently Gm7068) (Figure 1A) based on its sequence homology to the C-terminal extracellular domain of CatSperδ (Figure 1—figure supplement 1A). This testis-specific gene (Figure 1—figure supplement 2A) is predicted to encode a single transmembrane (TM) protein (Figure 1C and Figure 1—figure supplement 1B,C). In addition, a small soluble protein encoded by another testis-specific gene, Tex40 (Figure 1—figure supplement 2A), was found to be associated with the CatSper channel complex (Figure 1B and C, and Figure 1—figure supplement 1D). In this study, we refer to the C1orf101-like and Tex40 genes as CatSpere and CatSperz, respectively (see Molecular Cloning, Materials and methods). Like the other CatSper accessory subunits (Chung et al., 2011), both CatSpere and CatSperz mRNAs express specifically in germ cells and are detected before CatSper1 expression during postnatal development (Figure 1—figure supplement 2B,C). Moreover, mouse CatSper ε and ζ proteins partition into the testis microsome fraction (P) (Figure 1—figure supplement 2D), complex with CatSper1, and exhibit interdependence with the expression of the other CatSper subunits (Figure 1D–1F). In both human and mouse sperm cells, CatSper ε and ζ proteins are localized to the principal piece of the tails (Figure 1G and H and Figure 1—figure supplement 2E–G).
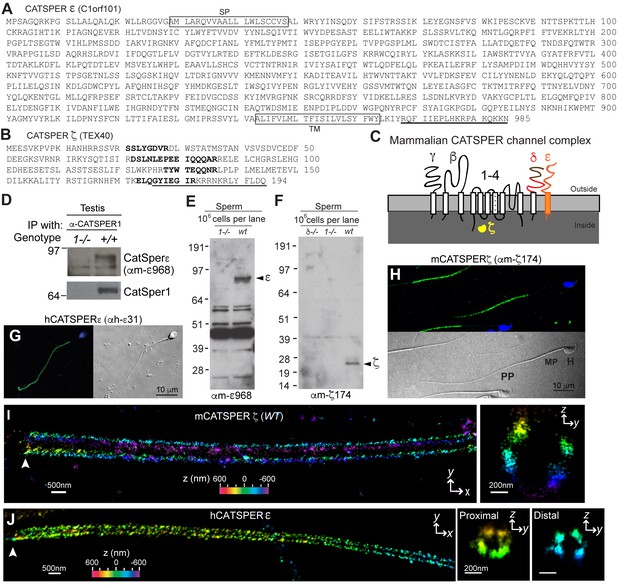
CatSper ε and ζ, two new accessory proteins of CatSper channel complex.
(A and B) Mouse protein sequences of CatSper ε (A) and ζ (B). (C) Cartoon of the predicted topology of 9 CatSper subunits. (D) Association of CatSperε with CatSper1 in testis. (E and F) Dependence of CatSper ε (E) and ζ (F) proteins on CatSper1 in mouse sperm cells. (G and H) Confocal fluorescence and the corresponding phase-contrast images of immunostained human CatSperε (G) and mouse CatSperζ (H). (I) 3D STORM images of mouse CatSperζ in capacitated wt sperm. x-y projection (left) and a y-z cross-section (right) at 0.5 um from the annulus. The color encodes the relative distance from the focal plane along the z axis (color scale bar in x-y projection). (J) 3D STORM images of human CatSperε in x-y projection (left), in y-z cross-sections (right). Colors indicate the z positions (see color scale bar). See also Figure 1—figure supplements 1–2.
CatSper ε and ζ localize at quadrilateral Ca2+signaling domains in sperm flagella
Mouse CatSper proteins form a unique pattern of four linear (‘racing stripes’) Ca2+ signaling domains running down the four quadrants of the principal piece of the flagellum (Chung et al., 2014). We examined whether ε and ζ share this distinctive compartmentalization. The antibodies, anti-hε31, recognizing the N-terminal extracellular region of human CatSperε, and anti-mζ174, against the very C-terminus of mouse CatSperζ, were suitable for 3D stochastic optical reconstruction microscopy (STORM) (Figure 1F–1H and Figure 1—figure supplement 2E). CatSperζ and CatSperε show the apparent four-fold arrangement of CatSper1, β and δ subunits in mouse (Figure 1I) and human (Figure 1J) spermatozoa.
CatSperz-null male mice have severely impaired fertility
The lack of functional expression of CatSper channels in heterologous systems requires that genetic manipulation be used to study the function of each component. CatSpere has the same ancient origin at the root of early eukaryotic evolution as those of CatSpers1-4, b, and g and the same pattern of extensive lineage-specific gene loss as CatSperb and g through metazoan evolution (Figure 2—figure supplement 1A) (Cai et al., 2014). While CatSper δ and ε share high C-terminal sequence homology (Figure 1—figure supplement 1A), CatSperδ appears later in evolution (Figure 2—figure supplement 1A). In contrast, CatSperζ has no conserved domains and, like hyperactivated motility, is only present in mammals (Figure 2—figure supplement 1A), leading us to speculate that CatSperζ is a required evolutionary adaptation to mammalian fertilization. Based on sequence homology and conservation, we anticipated that deletion of CatSperε would likely be the same as the existing knockout of other CatSper subunits, but deletion of CatSperζ might provide new insights into spermatozoan adaptations to changes concomitant with the evolution of mammalian fertilization. To test this idea, we began by generating a CatSperz-null mouse line from Tex40 gene targeted ES cell clones. Tex40 is a small gene composed of 5 exons that spans only ~3 kb on chromosome 11 (Figure 2—figure supplement 1B). Deletion of exons 2–4 was confirmed in the homozygous null mouse (Figure 2—figure supplement 1C). No CatSperζ protein was detected in Tex40-null spermatozoa by immunoblotting and immunocytochemistry (Figure 2—figure supplement 1D,E).
CatSperz-null mutant mice are indistinguishable from their wt or heterozygous (het) littermates in appearance, gross behavior, or survival. In addition, no morphological differences were observed by histological examination of testis and epididymis (data not shown). Sperm morphology and epididymal sperm number from CatSperz-null mice were not significantly different from those of 2–3 month old paired heterozygous littermates (Figure 2—figure supplement 1E and Figure 2—figure supplement 2A). CatSperz-null female mice exhibited normal mating behavior and gave birth to litters comparable to those of het females when mated with wt or het males (Figure 2—figure supplement 2B). However, when CatSperz-null male mice were mated with wt or het females, they were severely subfertile: 20% (5/25) CatSperz-null males were completely infertile over six months (Figure 2A), and progeny of the fertile paternal CatSperz-null mice were significantly fewer in number (Figure 2B and Figure 2—figure supplement 2B). The latency from pair formation to the birth of these offspring from CatSperz-null males was ≥10 days compared to those from wt or het males (data not shown).
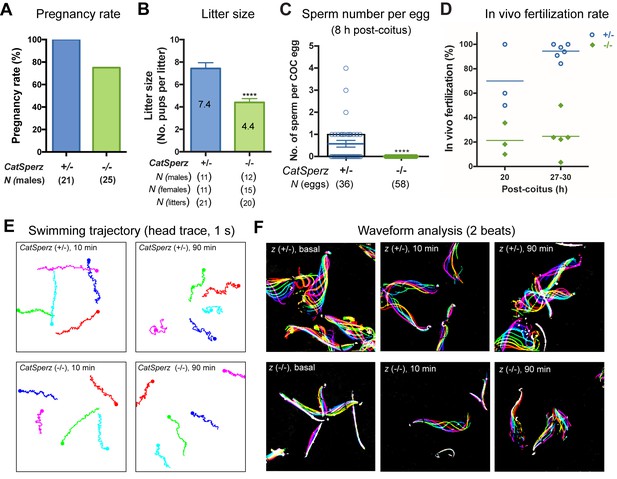
Deletion of the mouse CatSperζ subunit severely impairs male fertility.
(A) Percent pregnancy rate over three months. (B) Average litter size resulting from CatSperz+/- (7.4 ± 0.5) and CatSperz-/- (4.4 ± 0.3) males. (C) Sperm number per egg at the fertilization site 8 hr after 1 hr window-timed coitus with CatSperz+/- (0.58 ± 0.15) and CatSperz-/- (0, none) males, quantified from eggs collected from ampullae. (B) and (C) Data are mean ± SEM. ****p<0.0001. (D) In vivo fertilization rate: Scatter plot with mean % of 2 cell fertilized eggs from CatSperz+/- (70% and 94.4%) and CatSperz-/- (21.3% and 24.6%) mated females at 20 and 27–30 hr after coitus, respectively. (E) Head trace of free swimming CatSperz+/- (top) and CatSperz-/- (bottom) sperm cells at 10 min (left) and 90 min (right) after capacitation. Traces are from 1 s movies taken at 37°C. (F) Flagellar waveform traces. Movies recorded at 200 fps: CatSperz+/- (top) and CatSperz-/- (bottom) sperm cells attached on glass coverslips before capacitation (left), and 10 min (middle), and 90 min (right) after capacitation. Overlays of flagellar traces from two beat cycles are generated by hyperstacking binary images; time coded in color. See also Figure 2—figure supplements 1–2 and Figure 6—figure supplement 1.
-
Figure 2—source data 1
Impaired male fertility in CatSperz-/- mice: pregnancy rate, litter size, sperm number per egg, and in vivo fertilization rate.
- https://doi.org/10.7554/eLife.23082.008
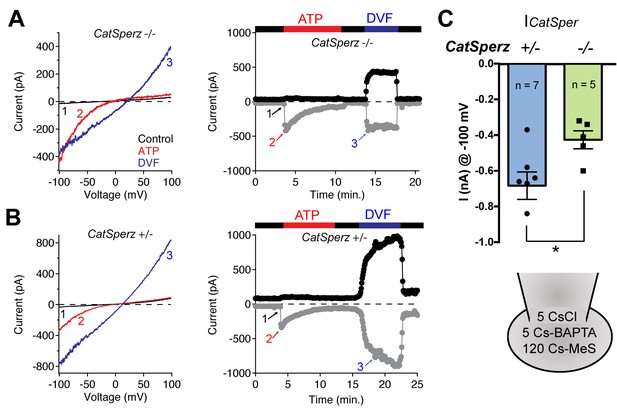
ICatSper, but not ATP-activated P2X2 current, is reduced in CatSperz-null spermatozoa.
(A) CatSperz-/- and (B) CatSperz+/- ICatSper. Left panels show the current-voltage relations of monovalent ICatSper in response to voltage ramps at the time points indicated. Right traces are representative time courses of ICatSper measured in the standard bath solution (1, HS), ATP-activated P2X2 current (2, ATP), and nominally divalent-free solution (3, DVF) at −100 mV (gray circles) and +100 mV (black circles). ICatSper in CatSperz-null sperm cells is ~60% of that recorded from wt. Inward IATP current induced by 100 µM ATP is similar in both phenotypes and indistinguishable from previously published wt IATP (Navarro et al., 2011). (C) Average ICatSper measured from CatSperz+/- (−683 ± 77 pA) and CatSperz-/- (−426 ± 50 pA) sperm cells at −100 mV. Data are mean ± SEM. p=0.0297. Cartoon shows the standard pipette solution (mM); internal Cs used to block K+ currents.
-
Figure 3—source data 1
Inward CatSper current at −100 mV.
- https://doi.org/10.7554/eLife.23082.013
We examined the number of sperm within cumulus oocyte complexes (COCs) after copulation and checked in vivo fertilization rates by isolating the COCs and/or embryos from the female ampullae. At 8 hr after coitus, no sperm was found in the COCs when mated with CatSperz-null male mice (Figure 2C). In contrast, the majority of the COCs from CatSperz-het mated females had one or more sperm cells within the complex. When mated with CatSperz-het males, more 2 cell eggs were observed over time after coitus, while the fertilization rate by CatSperz-null males did not change significantly (Figure 2D and Figure 2—figure supplement 2C). These data suggest that CatSperz-null sperm migration is delayed in the female reproductive tract.
CatSperz-null sperm cells have rigid proximal flagella
To understand why CatSperz-null spermatozoa did not efficiently migrate in the female reproductive tract, we first investigated sperm motility using computer assisted sperm analysis (CASA) (Figure 2—figure supplement 2D). The percentage of motile spermatozoa was not significantly different and most motility parameters of z-null spermatozoa were comparable to those of z-het sperm cells. However, the characteristic increase of lateral head displacement upon capacitation was not observed in z-null spermatozoa (Figure 2—figure supplement 2D), suggesting that hyperactivated motility was reduced. Ninety minutes after capacitation, there was a less marked difference in swimming trajectories of z-null spermatozoa compared to z-het spermatozoa, supporting this notion (Figure 2E and Video 1). Further analysis of flagellar amplitude and waveforms of tethered spermatozoa revealed a striking rigidity of z-null spermatozoa from their midpiece to midway down the principal piece (Figure 2F and Video 2). This phenotype was also observed from hyperactivation-deficient CatSper2-null patients (Smith et al., 2013). After incubation under capacitating conditions for 90 min, we observed that z-null spermatozoa beat only at the very distal end of a flagellum (Video 3). Moreover, CatSperz-null spermatozoa remain bent in the anti-hook direction (Ishijima et al., 2002) (Figure 2F, Videos 2 and 3). The anti-hook bend predominates as the pro-hook bend (initiated by the CatSper-mediated Ca2+ signaling pathway (Chang and Suarez, 2011) is dysregulated in CatSperz-null spermatozoa.
Movement of free swimming CatSperz +/- and -/- spermatozoa; related to Figure 2.
Uncapacitated (left) and 90 min capacitated (right) spermatozoa were allowed to disperse for 10 min pre-incubation in a 37°C chamber containing HEPES-HTF; free swimming sperm cells recorded within the next 5 min; video rate 20 fps (1/5 speed), 1 s movies; head trace to track swimming trajectory. (A) CatSperz+/- and (B) CatSperz-/- spermatozoa.
Motility of tethered CatSperz +/- and -/- spermatozoa; uncapacitated, related to Figure 2.
Uncapacitated epididymal spermatozoa in non-capacitating M2 media were tethered to the fibronectin-coated glass bottom dish; sperm motility was recorded at 37°C; video rate 200 fps, 2 s movies. (A) CatSperz+/- and (B) CatSperz-/- spermatozoa.
Motility of tethered CatSperz +/- and -/- spermatozoa; 90 min capacitated, related to Figure 2.
After 90 min incubation in HTF, capacitated epididymal spermatozoa were tethered to a fibronectin-coated glass bottom dish; sperm motility was recorded at 37°C; video rate 100 fps (1/2 speed), 1 s movies. (A) CatSperz+/- and (B) CatSperz-/- spermatozoa.
Reduced CatSper current in CatSperz-null spermatozoa
To examine how Ca2+ signaling in CatSperz-null spermatozoa is impaired, we first examined ICatSper, the sperm-specific Ca2+-selective ion current. Since Ca2+ has high affinity to calcium-selective pores (Almers et al., 1984), CatSper permeation of monovalents increases when external calcium is removed (Kirichok et al., 2006; Navarro et al., 2007). In divalent-free (DVF) solutions, wt spermatozoan ICatSper conducts a large Na+ current, which is completely absent in mice lacking CatSpers1, 2, 3, 4, or d (Chung et al., 2011; Kirichok et al., 2006; Qi et al., 2007). However, in CatSperz-null spermatozoa, monovalent CatSper current is present but reduced to ~60% of normal (−426 ± 50 pA at −100 mV; Figure 3A) compared to control CatSperz-het spermatozoa (−683 ± 77 pA at −100 mV; Figure 3B). Thus, in the absence of CatSperζ, the CatSper channel complex is still targeted to the flagellar membrane and forms functional channels. We hypothesize that the reduction in CatSper current reflected decreased protein expression levels.
P2X receptors are nonselective ion channels gated by purines such as ATP. The ATP-activated cation-nonselective current in the midpiece of murine sperm is mediated by the P2X2 purinergic receptor (Navarro et al., 2011). In CatSperz-null spermatozoa, IATP did not differ substantially from heterozygous spermatozoa (Figure 3A and B), supporting the assumption that there is selective down regulation of CatSper channels. Smaller ICatSper explains, in part, the attenuated hyperactivated motility, delayed sperm migration, and male subfertility (Figure 2A–2E). Protein tyrosine phosphorylation (P-Tyr), a hallmark of sperm capacitation, is potentiated and delocalized in CatSper knockout mice (Chung et al., 2014) or when Ca2+ influx is pharmacologically blocked (Navarrete et al., 2015). Upon capacitation, P-Tyr was more prominent in CatSperz-null spermatozoa than wt, but to a lesser extent than CatSper1-null spermatozoa (Figure 2—figure supplement 2E), consistent with the reduced calcium current. It is, however, also possible that an altered arrangement of the CatSper complex and/or its interaction with target proteins in the linear domains could have contributed to these functional deficits.
Abrogation of CatSperz retards targeting of the CatSper complex to flagella
To better understand why ICatSper is reduced in CatSperz-null spermatozoa, we examined levels of protein expression in CatSperz-null spermatozoa (Figure 4A). Expression of other CatSper subunits was detected in CatSperz -null spermatozoa, albeit at 30–60% lower levels than that of wt (Figure 4B), consistent with reduced ICatSper (Figure 3). This contrasts with the complete absence of other CatSper subunits in CatSper1- and CatSperd-null spermatozoa. mRNA and protein levels of other CatSper subunits were not reduced in the testis of CatSperz-null mice (Figure 4C and D), suggesting that the defect occurs during or after assembly of the protein complex.
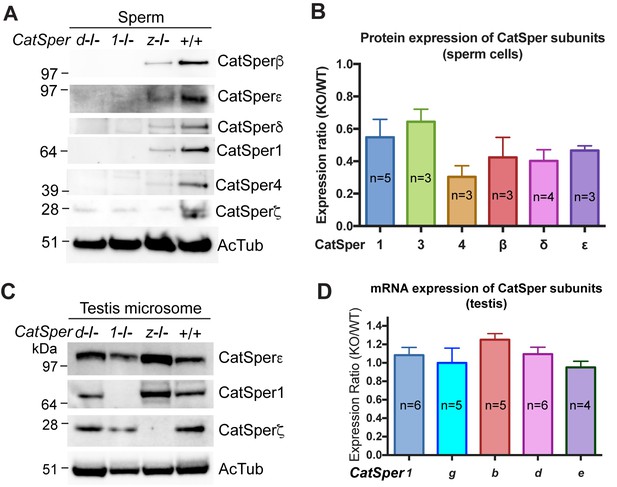
CatSper proteins are reduced in sperm from CatSperz-null mice despite protein expression during spermatogenesis.
(A and B) Reduced expression of CatSper subunits in sperm cells of CatSperz homozygous null mice compared with their complete absence in CatSper1 and d-null mice. Immunoblotting of (A) total mouse sperm extracts and (B) protein expression ratio (z-KO/wt) of CatSper 1 (0.5 ± 0.1), 3 (0.6 ± 0.08), 4 (0.3 ± 0.07), β (0.4 ± 0.1), δ (0.4 ± 0.07), and ε (0.5 ± 0.03). Data are mean ± SEM. (C) Increased expression of CatSper1 and ε in mouse testis in CatSperz-null mutants. (D) Quantitative gene expression analysis (qRT-PCR) from adult CatSperz-het and null testes: expression ratio (2-ddCT) and mean ddCt (null-het); TATA binding protein (TBP) is the internal control. The expression ratio of CatSper1 (1.1 ± 0.1) and all accessory g (1.0 ± 0.2), b (1.3 ± 0.07), d (1.1 ± 0.08), and e (0.95 ± 0.07) subunits are mean ± SEM.
-
Figure 4—source data 1
Protein and mRNA expression of CatSper subunits.
- https://doi.org/10.7554/eLife.23082.018
CatSperz is essential in maintaining the continuity of linear flagellar CatSper Ca2+domains
Loss of CatSperz resulted in fragmentation of CatSper1 staining on the flagellar membrane and these defects are large enough to be resolved by confocal imaging (Figure 5A). These gaps were not observed in wt/het (Figure 1H and Figure 2—figure supplement 1E) or previous wt and CatSper knockout studies (Chung et al., 2011; 2014; Liu et al., 2007; Ren et al., 2001). 3D STED and 3D STORM super-resolution microscopies clearly demonstrate that structural continuity is interrupted in CatSperz-null spermatozoa - each ‘stripe’ of the CatSper domains is fragmented, while the overall quadrilateral structure is maintained (Figure 5B and C). Cross-sections of the 3D STORM image of wt flagellum show the normal four tight clusters (Figure 5C, lower), represented as four lines in the 2D angular profiles of surface localizations (Figure 5—figure supplement 1A,E; inset) as previously observed (Chung et al., 2014). In CatSperz-null spermatozoa, however, the four lines in the 2D angular profiles were interrupted (Figure 5—figure supplement 1B). To examine whether the interruptions were periodic, we performed autocorrelation analysis and Fourier transform of STORM images of CatSperz-null sperm flagella (Figure 5—figure supplement 1C–F). Autocorrelation analysis of the CatSperz-null sperm flagella exhibited enhanced periodicity compared to the wt flagellum, with the first peak at ∼850 nm (Figure 5—figure supplement 1C,D). The Fourier transform shows a fundamental frequency of (800 nm)−1 (Figure 5—figure supplement 1F). We assume this thinning of one or more linear domains reflects an underlying structural periodicity that regulates CatSper complex trafficking or membrane insertion.
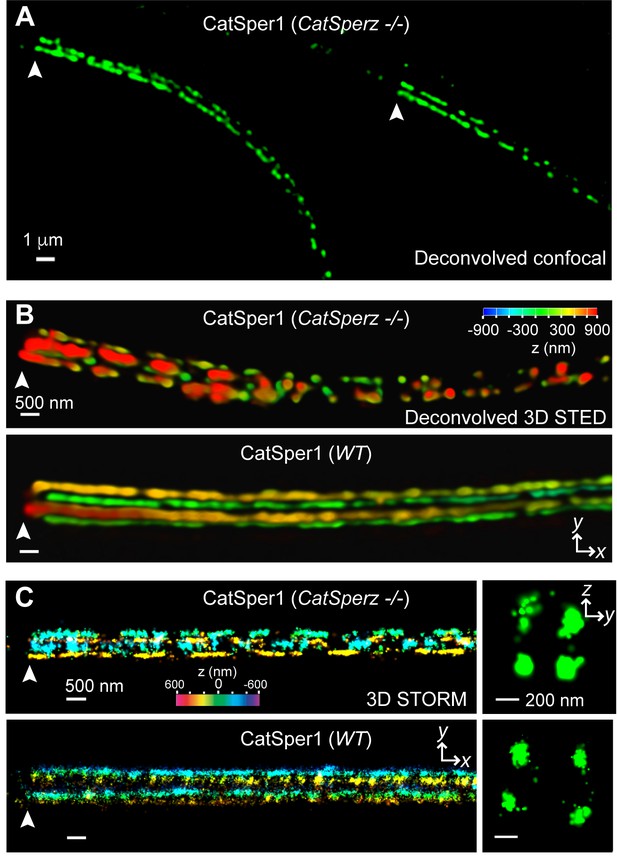
CatSperz deletion disrupts the continuity of the CatSper linear domains.
Application of different modes of fluorescence microscopy to observe CatSper localization. (A) Deconvolved confocal image of α-CatSper1 immunostained CatSperz -null spermatozoa. Scale bar, 1 μm. (B and C) 3D super-resolution images of CatSper1. 3D STED (B) and 3D STORM (C) images of CatSperz -null (top) and wt (bottom) sperm flagella, respectively. x-y projection colors encode the relative distance from the focal plane along the z axis. Scale bar, 500 nm. Arrowheads indicate the junction between the mid-piece and the principal piece (annulus) of the tail. 3D STORM, y-z cross-section images are shown on the right. Scale bar, 200 nm. See also Figure 5—figure supplement 1.
CatSperz-null spermatozoa rheotax inefficiently with reduced torque
Thus far, our results show that CatSperz-null sperm have reduced ICatSper, dysregulated structural continuity of the CatSper Ca2+ signaling domains, beat in an atypical pattern, and are delayed in migrating in the female reproductive tract, resulting in reduced male fertility. Rheotactic guidance for sperm over long distances requires rotational motion during CatSper-mediated hyperactivated motility (Miki and Clapham, 2013). We thus measured rheotactic parameters and the rotation rate of CatSperz-null spermatozoa with a particular focus on whether their proximal tail rigidity and subsequent low amplitude lateral movement (Figure 2) affects sperm movement. In flow-directed capillary tubes (Miki and Clapham, 2013), we observed that the rheotactic ability of CatSperz-null spermatozoa was significantly reduced (Figure 6A and B and Figure 6—figure supplement 1A). At all flow rates tested, most motile CatSperz-null spermatozoa were unable reorient to swim against the flow and were swept out of the tube (Video 4). In contrast, 85% of motile heterozygous spermatozoa displayed rheotactic behaviors by maintaining their position or swimming upstream for more than 2 s of the 9 s period of recording (Figure 6B and Video 4).
In-capillary rheotaxis of CatSperz +/- and -/- spermatozoa; capacitated, related to Figure 6.
Capacitated epididymal spermatozoa in HTF for 90 min were loaded into the capillary and transferred to a 37°C chamber; sperm cells swimming against the flow and down were recorded; video rate 33 fps, 9 s movies. (A) CatSperz+/- and (B) CatSperz-/- spermatozoa.
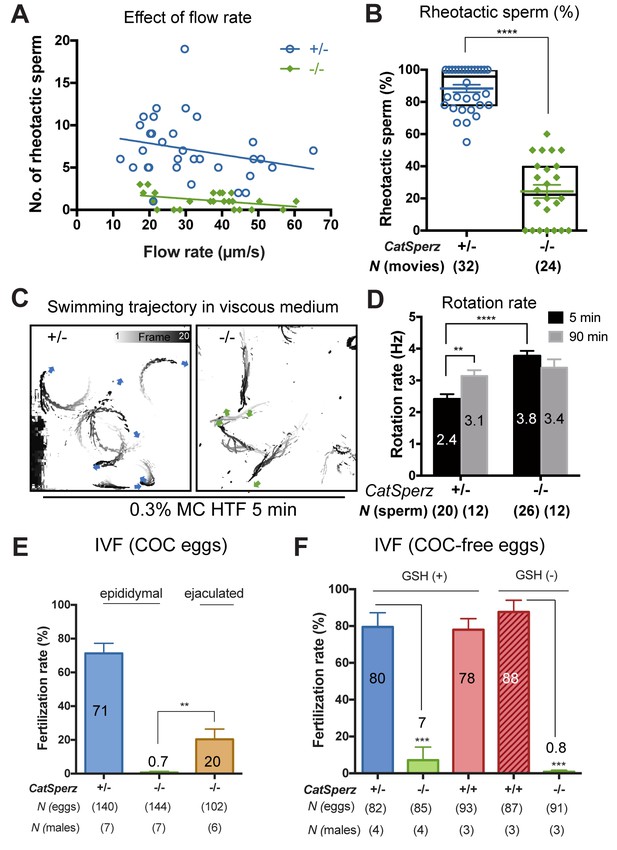
CatSperz-null sperm rheotax poorly due to low torque.
(A) In-capillary sperm rheotaxis. Rheotactic ability is reduced in sperm lacking CatSperz at all flow rates tested (12–65 µm/s). (B) Rheotactic sperm cells are expressed as the % of total motile spermatozoa counted from 9 s-movies (CatSperz+/-, n = 32; CatSperz-/-, n = 24). Data are expressed in scatter plots; mean ± SEM (colored bars) of CatSperz+/- (88 ± 2) and CatSperz-/- (24 ± 4) as well as median with interquartile ranges (black boxes) of CatSperz+/- (96, IQR 78–100) and CatSperz-/- (22.5, IQR 0–40). ****p<0.0001. (C) Trajectory of free-swimming sperm in 0.3% methyl cellulose. Movies were taken at 50 fps to compare CatSperz+/- (left) and CatSperz-/- (right) sperm cells; bottom of glass dish, 37°C, 5 min after incubation in capacitation medium (HTF). Overlays of flagellar traces (20 frames, 2 s movie) are generated by hyperstacking binary images with gray intensity scale; end frame in black. Arrows indicate sperm heads in each trace. (D) Sperm rotation rate from CatSperz+/- (5 min, 2.4 ± 0.2; 90 min, 3.1 ± 0.2, p=0.0064) and CatSperz-/- (5 min, 3.8 ± 0.2; 90 min, 3.4 ± 0.3) males after incubation in HTF. The sperm rotation rate is calculated as previously reported (Miki and Clapham, 2013). Data are mean ± SEM. ****p<0.0001. (E and F) IVF with epididymal and/or ejaculated CatSperz+/- and CatSperz-/- spermatozoa. 2 cell stage eggs were counted 24 hr after insemination. (E) IVF rate with cumulus-intact oocytes from CatSperz+/- (epididymal, 71 ± 6) and CatSperz-/- (epididymal, 0.7 ± 0.7; ejaculate, 20 ± 6, p=0.0051). (F) IVF rate of cumulus-free/ZP-intact eggs with (CatSperz+/-, 80 ± 8; CatSperz-/-, 7 ± 7, p=0.0005; wt, 78 ± 6) or without (CatSperz+/-, 88 ± 6; CatSperz-/-, 0.8 ± 0.8, p=0.0002) glutathione-containing (GSH; 2 mM) media. Data are mean ± SEM. See also Figure 6—figure supplement 1.
-
Figure 6—source data 1
In-capillary sperm rheotaxis and in vitro fertilization.
- https://doi.org/10.7554/eLife.23082.023
We next examined the rotational motion of CatSperz-null spermatozoa. At high viscosities (0.3% methyl cellulose (MC), cP = 6.7), uncapacitated z-het spermatozoa swim in circles (Figure 6C, left and Video 5), while capacitated z-het spermatozoa swim in a more linear path as they rotate around a longitudinal axis, like wt spermatozoa (Video 6) (Miki and Clapham, 2013). Interestingly, z-null spermatozoa exhibit linear migration as they can rotate along the tail axis regardless of capacitating conditions, even at higher viscosities (Figure 6C, right and Videos 5 and 6). Indeed, uncapacitated CatSperz-null spermatozoa rotate ~50% faster than z-het spermatozoa (Figure 6D). This indicates that CatSperz-null spermatozoa have less lateral motion and are subject to less torque by the moving stream. Spatially, the spermatozoa trace out a less conical 3D envelope (Figure 2F and Figure 6—figure supplement 1B). In short, the rigidity of the CatSperz-null sperm proximal tail constrains its motion to that of a propeller-driven rod.
Movement of CatSperz +/- and -/- sperm in viscous medium; uncapacitated, related to Figure 6.
Uncapacitated spermatozoa were allowed to disperse for 10 min pre-incubation in a 37°C chamber containing HEPES-HTF supplemented with 0.3% methylcellulose; swimming sperm cells were recorded within the next 5 min; video rate 50 fps, 2 s movies. (A) CatSperz+/- and (B) CatSperz-/- spermatozoa.
Movement of CatSperz +/- and -/- sperm in viscous medium; 90 min capacitated, related to Figure 6.
Spermatozoa capacitated in HTF were allowed to disperse for 10 min pre-incubation in a 37°C chamber containing HEPES-HTF supplemented with 0.3% (left), 0.4% (middle), or 0.5% (right) methylcellulose; swimming sperm cells were recorded within the next 5 min; video rate 50 fps, 2 s movies. (A) CatSperz +/- and (B) CatSperz-/- spermatozoa.
Compromised Ca2+signaling alters the sperm’s 3D flagellar envelope and movement
We next examined the relation between external calcium entry and sperm function. First, we tested whether increasing extracellular [Ca2+] could rescue z-null sperm motility. After incubation for 90 min with a two-fold greater [Ca2+], most CatSperz-null sperm remain bent in the anti-hook orientation with a rigid proximal tail (Figure 6—figure supplement 1C, z(-/-) middle, and Video 7). A few z-null spermatozoa partially recover, bending occasionally in the pro-hook direction with hyperactivated motility (Figure 6—figure supplement 1C, z(-/-) right, and Video 7). Conversely, a 20-fold reduction of extracellular [Ca2+] alone did not significantly alter the flagellar waveforms of z-het spermatozoa within 90 min (Figure 6—figure supplement 1C and Video 8).
Motility of tethered CatSperz-/- sperm in high external calcium; 90 min capacitated, related to Figure 6—figure supplement 1.
After 90 min incubation in Ca2+-HTF under capacitating conditions, CatSperz-/- spermatozoa were tethered to a fibronectin-coated glass bottom dish; sperm motility was recorded within the next 5 min at 37°C; video rate 100 fps (1/2 speed), 1 s movies. (A) 2 Ca2+-HTF and (B) 4 Ca2+-HTF (in mM).
Motility of tethered CatSperz+/- sperm in low external calcium; 90 min capacitated, related to Figure 6—figure supplement 1.
After 90 min incubation in Ca2+-HTF under capacitating conditions, CatSperz+/- spermatozoa were tethered to a fibronectin-coated glass bottom dish; sperm motility was recorded within the next 5 min at 37°C; video rate 100 fps (1/2 speed), 1 s movies. (A) 2 Ca2+-HTF, (B) 0.5 Ca2+-HTF and (C) 0.1 Ca2+-HTF (in mM).
A transient Ca2+ pulse induced by Ca2+ ionophore, A23187, significantly reduces the time required for wt sperm to develop hyperactivated motility (Tateno et al., 2013). Moreover, a short (10 min) exposure to A23187 can rescue defects in hyperactivated motility and the fertilizing capability of CatSper1-null sperm in vitro (Navarrete et al., 2016). We tested the relation of calcium transients to hyperactivated motility in CatSperz-het and null sperm. In z-het spermatozoa, an A23187-induced Ca2+ pulse followed by washout, enables full hyperactivation, characterized by wide lateral displacement with large midpiece α angle within 30 min (Figure 6—figure supplement 1D and Videos 9 and 10). However, in z-null sperm, the same treatment improved the flexibility of the proximal flagella, particularly in the principal piece, but the midpiece remained largely inflexible (Figure 6—figure supplement 1D and Videos 9 and 10). Building on our previous work, the present study suggests that calcium entry through CatSper channels has time-dependent, complex effects on the coordination of motility and that loss of CatSperz results in reduced ICatSper, changes in calcium signaling, and structural alterations of the flagellum.
Motility of tethered CatSperz +/- and -/- sperm after A23187 treatment; 5 min after wash, related to Figure 6—figure supplement 1.
Spermatozoa treated with 20 µM A23187 in H-HTF for 10 min were washed and incubated in HTF under capacitating conditions for 5 min; sperm were tethered to a fibronectin-coated glass bottom dish and the motility was recorded in H-HTF within the next 5 min at 37°C; video rate 100 fps (1/2 speed), 1 s movies. (A) CatSperz+/- and (B) CatSperz-/- spermatozoa.
Motility of tethered CatSperz +/- and -/- sperm after A23187 treatment; 30 min after wash, related to Figure 6—figure supplement 1.
Spermatozoa treated with 20 µM A23187 in H-HTF for 10 min were washed and incubated in HTF under capacitating conditions for 30 min; sperm were tethered to a fibronectin-coated glass bottom dish and the motility was recorded in H-HTF within the next 5 min at 37°C; video rate 100 fps (1/2 speed), 1 s movies. (A) CatSperz+/- and (B) CatSperz-/- spermatozoa.
CatSperz-null sperm cells inefficiently penetrate the egg cumulus
We performed in vitro fertilization (IVF) to determine how the low rotational torque generated by CatSperz-null spermatozoa affects sperm-egg interactions. We found that these spermatozoa cannot fertilize cumulus-intact oocytes (Figure 6E), but could dissociate the cumulus cell layers and bind to the ZP (data not shown). Cumulus removal did not change the fertilization rate of ZP intact oocytes by CatSperz-null spermatozoa. Furthermore, this rate was only marginally enhanced by destabilization of the ZP by 2 mM glutathione (Figure 6F) (Miyata et al., 2015). This indicates that reduced hyperactivated motility alone does not explain the failure of CatSperz-null spermatozoa in IVF. One possibility is that the kinetics of capacitation in vitro is different from that in vivo, resulting from fluctuations in timing or amplitude of known factors (e.g., HCO3, pH) or from unknown factors present in seminal and/or female fluids. We then compared IVF rates with ejaculated and epididymal spermatozoa of CatSperz-null mice. When ejaculated spermatozoa flushed from the uterus of the mated females were used in IVF trials, 20% of oocytes incubated with z-null spermatozoa developed into two-cell embryos (Figure 6E). This compares to 50% of oocytes incubated with z-het ejaculated (data not shown), or epididymal sperm. Thus, additional factors may be functionally relevant in in vivo fertilization.
Discussion
Complex protein composition and conservation of compartmentalization in mammals
Sperm hyperactivation and normal fertility in mammals requires the unique CatSper channel complex. With four distinct pore-forming gene products (CatSper 1–4) and, now, five accessory subunits (β, γ, δ, ε, and ζ), the CatSper channel is the most complex of known ion channels. This may reflect the relatively high evolutionary pressure on spermatozoan evolution (Swanson and Vacquier, 2002; Torgerson et al., 2002), and various adaptations to different modes of fertilization. Like many gamete-specific proteins and the other CatSper proteins reported so far (Cai and Clapham, 2008; Chung et al., 2011), mouse and human CatSper ε and ζ show signs of rapid evolutionary change with only 50% and 45% amino acid sequence identity, respectively. In particular, the sequence regions outside TM segments and the pore loop of CatSper proteins are poorly conserved across species, indicating these regions possibly convey species-specific modulation of flagellar motility (Miller et al., 2015). This is illustrated by striking differences in progesterone-elicited ICatSper responses in mouse and human (Lishko et al., 2011). Here we have shown that CatSper ε and ζ are components of the highly organized CatSper complex, that CatSperζ is required for proper continuity of this complex along the flagellum, and that loss of ζ alters hyperactivation waveforms and reduces fertilizing capacity.
The conservation pattern of the lineage-specific gain and loss of the CatSpere gene is identical to those of b and g, suggesting that they likely belonged to an ancient CatSper channel Ca2+ signaling network before the divergence of unikonts and bikonts. Since their protein expression is strictly interdependent, we speculate that CatSpere-null mice will have the phenotype of CatSper1-4, or d-null mice. In contrast, CatSperz is conserved only in mammals, suggesting that this protein imparts some adaptation, perhaps as a method enabling rheotaxis in the mammalian female reproductive tract.
Interestingly, although CatSperζ has no putative transmembrane domains, it is localized in the same quadrilateral pattern as other CatSpers, but is not present elsewhere in sperm. An intriguing aspect of our observations is that, unlike CatSper1-4 and d-null mice, which produce complete infertility, CatSperz-null males exhibit an incomplete loss of fertility. The CatSper current is reduced in CatSperz-null spermatozoa, and may have similar permeation properties (likely dominated by the CatSper1-4 pore subunits), but the effects of CatSperz on channel gating remain to be determined in future studies. This is reminiscent of the non-spermatozoan, voltage-gated Cav channel auxiliary subunits, which are not required for expression but modulate expression levels and gating (Catterall et al., 2005). Most tantalizing is the thinning and disruption of the linear CatSper signaling domains at repeat intervals in the absence of ζ. Further detailed examination via mutagenesis experiments has been stymied by our inability to heterologously express functional CatSper channels. New rapid genome editing techniques should enable more mice to be generated that will further the study of CatSper trafficking, subunit interactions, and localized signaling pathways.
Traffic into the linear domains of sperm flagella
Functional Ca2+ signaling domains are common adaptations in many biological systems, such as synapses and muscle. They enable specific and fast triggering of downstream events (Clapham, 2007). CatSper channels are compartmentalized into a unique multilinear arrangement and form Ca2+ signaling nanodomains with other Ca2+ signaling molecules along the sperm flagellum (Chung et al., 2014). The mechanisms involved in the delivery of the CatSper channels to these specific domains are currently unknown, and we suspect will be as interesting and complex as those in primary and motile cilia (Sung and Leroux, 2013). We found that abrogation of CatSperz not only retards targeting of the CatSper complex to flagella, but also disrupts continuity of the linear domains, resulting in repeated fragmented domains with ~800 nm periodicity. In order for CatSper domains to form and function properly, interactions are needed between the CatSper channel complex in the flagellar membrane and the underlying cytoskeletal proteins. One speculation is that CatSperζ might adapt to cytoskeletal structures that traffic, distribute, and enable membrane insertion of CatSper.
The fibrous sheath (FS), a cytoskeletal structure unique to the mammalian sperm flagellum, defines the extent of the tail’s principal piece, in which all the CatSper proteins are specifically localized. The FS closely lies under the plasma membrane and its two longitudinal columns are connected by circumferential ribs. Immunogold electron microscopy demonstrated that the CatSper channels are distributed on the end of ribs, where they merge with the column (Chung et al., 2014). It seems likely that the timing of occurrence and localization of CatSper Ca2+ signaling domains is coordinated with the assembly of FS proteins along the axoneme. The column appears early in spermiogenesis, forming from the distal tip of the tail along the axoneme, followed by subsequent rib formation in the opposite direction (Oko, 1998; Oko and Clermont, 1989). Based on scanning electron micrographs (Danshina et al., 2010; Miki et al., 2004), we find that the distance between ribs is about 800 nm in mouse spermatozoa. Thus, it seems likely that the repeated disruption in the absence of CatSperz is related to rib spacing of the FS.
Ca2+regulation of the flagellar envelope in sperm rheotaxis and egg penetration
Genetic abrogation of CatSper disrupts hyperactivated motility as manifested by changes in movement symmetry, amplitude, and rolling (Carlson et al., 2003; Chung et al., 2011; Miki and Clapham, 2013; Qi et al., 2007). Here we report that the flagellar envelope is significantly altered in the absence of CatSperz, in part due to the inflexibility of the proximal tail. We previously reported that the catalytic subunit of calcineurin, PP2B-Aγ, expresses throughout the tail but localized to the CatSper quadrilateral structures and axoneme (Chung et al., 2014). In CatSper1-null spermatozoa, PP2B-Aγ remains localized primarily to the axoneme but disappears from the quadrilateral structures. Recently, a similar but not identical phenotype (inflexible midpiece, reduced hyperactivated motility, and impaired ZP penetration) was reported in testis-specific calcineurin Ppp3cc-null and Ppp3r2-null spermatozoa (Miyata et al., 2015). Note that the principal piece of both CatSper1-null and Ppp3cc-null spermatozoa are not rigid. The integrity and distribution of CatSper channels in Ppp3cc-null spermatozoa remain to be examined and may clarify midpiece/principal piece disparities. In any case, inflexibility in the proximal regions of flagellum results in a flagellar envelope approximated as a rod with a distal propeller. The sperm can rotate faster but the smaller lateral deviation reduces torque. This limits the sperm’s ability to orient into the flow, as well as penetrate the cumulus and ZP.
Physiological modulation of CatSper in sperm function and fertility
Gene-manipulated mice highlight the importance of in vivo observations and have reshaped the landscape of fertilization science (Okabe, 2015). In vitro capacitation and fertilization systems underpin much of the study of sperm motility and fertilization potential. While ejaculated sperm are preferred for fertilization studies in larger animals and humans, epididymal sperm are commonly used in genetically tractable mouse studies. Notably, these sperm are not exposed to accessory sex gland secretions and female fluids. This may explain why CatSperz-null spermatozoa are completely infertile in an IVF setting (COCs), but in vivo are merely subfertile. Perhaps natural modulators, absent in epididymal sperm IVF studies, partially rescue the fertilizing potential of CatSperz-null spermatozoa by activating Ca2+ signaling activity.
Interestingly, a transient pulse of Ca2+ can greatly reduce the capacitation time required for wt sperm to develop hyperactivated motility (Tateno et al., 2013). Moreover, Navarrete et al recently demonstrated that a short exposure to A23187 rescued the defects in motility and fertilizing capability of CatSper1-null sperm in vitro (Navarrete et al., 2016). These independent studies were interpreted to mean that the initial priming by Ca2+ influx, perhaps above a certain threshold, is essential for sperm function. However, the linear quadrilateral CatSper complexes are not present in CatSper1-null spermatozoa and in CatSperz-null spermatozoa are disrupted by gaps. We hypothesize that the linear quadrilateral structure in vivo likely maintains, regulates, and distributes CatSper Ca2+ signaling during hyperactivated motility. But it is important to point out that alterations in the structure should also result in changes in mechanical properties, movement of the flagellum, distribution of entering calcium, and downstream kinase activity and the motor elements they regulate. This complexity is illustrated in vivo sperm swimming trajectories, which are modulated by switching between pro- and anti-hook beating patterns. In the absence of CatSperζ, anti-hook beating predominates. Pro-hook motions are associated with intact CatSper-mediated Ca2+ signaling pathways (Chang and Suarez, 2011). Finally, ejaculated sperm display more pro-hook hyperactivation than epididymal sperm (Li et al., 2015).
Future areas for investigation are the functional positioning of the remaining accessory subunits of the CatSper channel in assembly and domain organization, the testing of potential modifiers present in accessory sex gland secretions that may activate CatSper channels, and the determination of Ca2+ dependent molecules in the axoneme which eventually determine flagellar bending and its envelope. CatSperz-null mice, which are hypomorphic to the null-mutation of other CatSper genes with abrogated hyperactivation, and newly expanding animal models from recent advances in genome editing will serve as a foundation to this end. Advanced imaging techniques with higher time and spatial resolution will be necessary to carry this out. The present results also suggest that alterations of Ca2+ current and/or dysregulated downstream Ca2+ signaling affecting dynamic structures may be sufficient to compromise sperm function. CatSper’s unique composition and central role in hyperactivated motility make it an ideal target for contraception.
Materials and methods
Details of source and identifier are provided in the Key Resources Table as a supplementary file.
Animals
CatSper1 and d-null mice were previously described (Chung et al., 2011; Ren et al., 2001). Lines were backcrossed and maintained on a C57BL/6 background. WT C57BL/6 male, B6D2F1 female (Jackson laboratory, Bar Harbor, ME), and CD1 (Charles River Laboratories, Wilmington, MA) female mice were purchased.
Generation of CatSperz-deficient mice and genotyping of mutant mice
Request a detailed protocol[1700019N12Riktm(KOMP)Mbp]-targeted ES cells (two clones, 1700019N12Rik_D05 and 1700019N12Rik_C06) were purchased from the UC Davis KOMP repository. The parental ES cell line, JM8A1.N3, was derived from C57BL/6N (agouti) ES cells. Chimeras were born from injection of the C06 ES cells into host embryos. The male chimeras were bred to C57BL/6N females to establish germline transmission and obtain heterozygous animals. Initially, genotype analysis was performed by PCR on isolated genomic DNA (F/R1/R2, F (JJC575): 5’-ATAACCATCCGGGAGGAGAC-3’, R1 (YS_zWT-Rev): 5’-GCGATGGTTTGCGTGTTTG-3’, R2 (JJC562): 5’- CACAACGGGTTCTTCTGTTAGTCC-3’). From F2 mice, genotyping was done by Transnetyx. The mice used in this study were the offspring of crosses between F1 and/or F2 generations (100% C57BL/6N genetic background). Mice were treated in accordance with guidelines approved by the Boston Children’s Hospital and Yale Animal Care and Use Committees (IACUC).
Mouse sperm preparation and in vitro capacitation
Mouse caudal epididymal sperm were collected by swim-out in HEPES buffered saline (HS) containing (in mM): 135 NaCl, 5 KCl, 2 CaCl2, 1 MgSO4, 20 HEPES, 5 glucose, 10 lactic acid, 1 Na pyruvate, pH 7.4 (with NaOH) (Chung et al., 2011). To induce capacitation in vitro, sperm cells were incubated (2 × 106 cells ml−1) in human tubular fluid (HTF) media (in mM): 102 NaCl, 4.7 KCl, 2 CaCl2, 0.2 MgCl2, 0.37 KH2PO4, 2.78 glucose, 18.3 lactic acid, 0.33 Na pyruvate, 25 HCO3- and 4 mg ml−1 BSA) (Millipore) for 90 min at 37°C (5% CO2).
Capacitation in varying external [Ca2+]
Request a detailed protocolTo test the effect of external [Ca2+] on the development of hyperactivated motility, 2 mM CaCl2 in standard HTF was replaced with 4 mM, 0.5 mM, and 0.1 mM CaCl2 and sperm cells were incubated for 90 min under capacitating conditions (37°C, 5% CO2).
Motility rescue by Ca2+ ionophore, A23187 treatment
Request a detailed protocolCa2+ transient-inducible hyperactivated motility was tested by treating sperm with A23187 as described (Navarrete et al., 2016; Tateno et al., 2013) with slight modification. In short, caudal epididymal mouse sperm were collected by swim-out in HEPES-HTF medium (H-HTF: 92 mM NaCl, 2 mM CaCl2, 4.7 mM KCl, 0.2 mM MgCl2, 0.37 mM KH2PO4, 25 mM NaHCO3, 18.3 mM Na lactate, 2.78 mM glucose, 0.33 mM Na pyruvate, 0.4% [w/v] bovine serum albumin [BSA], and 10 mM HEPES [pH 7.4]), allowing motile sperm to disperse for 10 min at 37°C. Sperm concentration was ~4 × 107 cells/mL. An aliquot (150 μL) of the sperm suspension was exposed to 20 μM A23187 in H-HTF. After 10 min, sperm were washed by two centrifugation at 550 G for 5 min and 300 G for 5 min, resuspended in standard HTF, and incubated at 2 × 106 cells/ml under capacitating conditions (37°C, 5% CO2). At 5 min and 30 min post-incubation, spermatozoa were tethered to fibronectin-coated coverslips and recorded in H-HTF at 37°C as described in Flagellar Waveform Analysis.
Human sperm preparation
Request a detailed protocolAll experiments using human samples were approved by the Committee of Clinical Investigation, Boston Children’s Hospital CCI/IRB (IRB-P00000538). Human semen samples were obtained from fertile donors. Human spermatozoa were collected by the swim-up method with the use of modified human tubal fluid medium (HTF).
Cell line origin and authentication
Request a detailed protocolHEK293T cells were purchased from ATCC. In this study, they were used to overexpress recombinant human CatSperζ in order to test antibodies. The cells were tested negative for mycoplasma and validated as of human origin. The identity was authenticated by confirming their negative expression of testis-specific genes including CatSper. The cell line was cultured in DMEM/F12 containing 10% FBS.
Antibodies and reagents
Request a detailed protocolRabbit polyclonal CatSper1, CatSper4, β, and δ antibodies were previously described (Chung et al., 2011; Ren et al., 2001). To produce antibodies to new CatSper subunits, peptides were synthesized and conjugated to KLH carrier protein (Open Biosystems, Lafayette, CO) as follows: mouse CatSperε, 968–985 (αm-ε968: RQFIIEPLHKRPAKQKKN); mouse CatSperζ, 174–195 (αm-ε174: GYIEGIRKRRNKRLYFLDQ); human CatSperε, 31–50 (αh-ε31: RIFSTRSTIKLEYEGTLFTE); and human CatSperζ, 11–29 (αh-ζ11: KSSDRQGSDEESVHSDTRD). Antisera were affinity purified on the immobilized resin of the corresponding peptide (Amino Link Plus or Sulfo Link Plus) (Pierce, Waltham, MA). Anti-phosphotyrosine (clone 4G10), anti-Flag (clone M2), anti-calmodulin (05-173) and anti-acetylated tubulin (T7451) antibodies were from EMD Millipore (Germany). All chemical compounds were from Sigma-Aldrich (St. Louis, MO) unless indicated.
Genomic database search
Request a detailed protocolAnnotated orthologs in the NCBI gene database (http://www.ncbi.nlm.nih.gov/gene/) and/or homologous amino acid sequences of reported protein databases were screened in 17 eukaryotes for the presence of genes for CatSper auxiliary subunits. Non-annotated orthologs in the NCBI gene database were identified by comparing sequences of the annotated orthologs to those in the protein database of species by Phmmer implemented on HMMER 3.1 (default option, http://hmmer.org/). The longest amino acid sequences among all the isoforms of the orthologs annotated in each species and protein sequence databases from 15 eukaryotes, except human and mouse, were downloaded from the NCBI Genome database (http://www.ncbi.nlm.nih.gov/genome; Tinamus guttatus, GCA000705375.2; Anolis carolinensis, GCA000090745.2; Salmo salar, GCA000233375.4; Callorhinchus milii, GCA000165045.2; Branchiostoma floridae, GCA000003815.1; Caenorhabditis elegans, GCA000002985.3; Crassostrea gigas, GCA000297895.1; Exaiptasia pallida, GCA001417965.1; Trichoplax adhaerens; GCA000150275.1, Salpingoeca rosetta, GCA_000188695.1), Ensembl genome browser (http://ensembl.org; Strongylocentrotus purpuratus, GCA000002235.2; Drosophila melanogaster, GCA000001215.4; Thecamonas trahens, GCA000142905.1), and JGI genome portal (http://genome.jgi.doe.gov; Allomyces macrogynus; Aurantiochytrium limacinum). Aligned phmmer hits of expected values <10−10 were considered as candidate orthologs of the corresponding CatSper subunits in each species.
Multiple tissue RT–PCR
Request a detailed protocolPCR was performed according to standard protocols using a commercial multiple panel cDNA template (MTC), Clontech). PCR primers amplified Gm7068 (forward: 5′-CTATGGCTCAAGTGTAATGACC-3′, reverse: 5′-GCTCTTATTGAATCCTCGAACC-3′), Tex40 (forward: 5′-GAAACAGGATTCGCAAGTACAG-3′, reverse: 5′-TCGTGGACCTATATGTGATGAG-3′) using mouse GAPDH (forward: 5′-TGAAGGTCGGTGTGAACGGATTTGGC-3′, 5′-ATGTAGGCCATGAGGTCCACCAC-3′) as a control.
Molecular cloning
Request a detailed protocolThe initial mouse Tex40 cDNA sequence (NM_001039494) was identified from database searches using novel peptide sequences from MS. The full-length human Tex40 cDNAs was obtained by PCR with primers (forward: 5′-GGGCAGAACCATGGAGGAAA-3′, reverse: 5′-AGGACTCAAATTCCACTCGGATG-3′) using the human testis cDNA library (Clontech). Sequencing the TOPO-cloned PCR products into pCR4-TA (Invitrogen) confirmed the full-length human Tex40 ORF, which was subcloned into pCMV-Tag2A (Stratagene) to express recombinant N-terminal Flag-tagged human CatSperζ in mammalian cells. Mouse Gm7068 was identified by homologous amino acid sequence to C-terminal CatSperd (Tmem146). There are six transcript variants (Almers et al., 1984); XM_006497083, 2; XM_006497084, 3; XM_017314031, 5: XM_006497085, 6; XM_017314033, and 8; XM_006497087). Variants 1, 3, 5, 6, and 8 are predicted to encode polypeptides with the same C-terminal sequence that can be detected by anti-mε−968. Among them, the predicted polypeptides from longer splicing variant 1 (isoform X1; XP_006497147, 985 aa) and variant 3 (isoform X3; XP_017169520, 914 aa) are consistent with the apparent molecular weight of the band observed in testes microsomes (Figure 4C and Figure 1—figure supplement 2D). The predicted polypeptides from shorter variant 5 (isoform 4; XP_006497148, 805 aa) and variant 6 (isoform 5; XP_017169522, 770 aa) are consistent with that of the band detected in CatSper1-IP from testis and total sperm lysate (Figures 1D,E and and 4A). It is likely that mouse Gm7068 expresses at least four potential splice variants that can encode protein isoforms and/or undergo cleavage during spermatogenesis.
RNA in situ hybridization
Request a detailed protocolIn situ hybridization experiments were carried out with an RNAscope (Advanced Cell Diagnostics, Newark, CA). Testes from three month old wild-type mice were fixed in 10% (vol/vol) neutral-buffered formalin at room temperature for 24 hr, dehydrated, and embedded in paraffin. Paraffin sections (10 μm thick) were processed according to the manufacturer’s instructions for in situ detection in the Rodent Histopathology Core Facility at Harvard Medical School. Sequences of the probes used in this study are: Gm7068 (XM_982472.3, 645–1072) and Tex40 (NM_001039494.2, 41–456). After the DAB (3,3, -diaminobenzidine) reaction, slides were counterstained using hematoxylin.
mRNA preparation and Real-time PCR
Request a detailed protocolReal-time PCR was carried out with first strand cDNAs (iScript cDNA Synthesis) (Bio-Rad, Hercules, CA) synthesized from 2 µg total mouse testis RNA using the SYBR Green (iTaq Universal SYBR Green Supermix) (Bio-Rad; CFX96). Quantitative analysis by the ddCt method employed c-Jun as an amplification control. Three independent sets of experiments were performed to calculate fold changes (2-ddCt) of CatSpers mRNA. The primers used for qRT-PCR were: CatSper1 (forward: 5′-CTGCCTCTTCCTCTTCTCTG-3′, reverse: 5′-TGTCTATGTAGATGAGGGACCA-3′), CatSperb (forward: 5′-CCTTA TTGACCAAGAAACAGAC-3′, reverse: 5′-TGAAACCCATATTTGACTGCC-3′), CatSperg (forward: 5′-TGAGCAATAGAGGTGTAGAC-3′, reverse: 5′-CAGGA TGTAGAAGACAACCAG-3′), CatSperd (forward: 5′-GCTGACATTTCTGTGTATCTAGG-3′, reverse: 5′-CTGATATACCTTCCAATTTACGCC-3′), CatSpere (forward: 5′-GTCTCATGCTTCTTCAGTTCC-3′, reverse: 5′- CAGAAGTTCCTTGTCCATCAC-3′), CatSperz (forward: 5′-GAGACCTCCTTAGCATCGTC-3′, reverse: 5′-TCGTGGACCTATATGTGATGAG-3′ and c-Jun (forward: 5′-CTCCAGACGGCAGTGCTT-3′, reverse: 5′-GAGTGCTAGCGGAGTCTTAACC-3′).
Preparation of mouse testis microsome
Request a detailed protocolTestes (200 mg, normally two testicles) from 8- to 12-wk-old male mice were homogenized on ice using a Dounce homogenizer in 2 mL 0.32 M sucrose solution with protease inhibitor cocktails (Roche). The tissue suspension was centrifuged at 300 g for 10 min at 4°C and the supernatant was then transferred to an ultra-speed centrifuge tube. The microsome faction was isolated by centrifuging the tube at 105,000 g for 60 min.
Protein preparation, immunoprecipitation, and western blotting
Request a detailed protocolMouse sperm total protein was prepared as described before (Chung et al., 2011; 2014). For total protein from human spermatozoa, purified swim-up sperm were then lysed (0.1% SDS, 0.5% sodium deoxycholate, 1 mM DTT, 1 mM EDTA in PBS with protease inhibitors) followed by sonication for 5 min and centrifuged at 15,000 g for 10 min. The supernatants were further denatured by adding DTT to 10 mM and heated at 75°C for 10 min before SDS-PAGE. For immunoprecipitation, the testis microsome pellet was resuspended in 10 mL 1% Triton X-100 in PBS with protease inhibitors (Roche). The suspension was rocked at 4°C for 1 hr and then centrifuged at 15,000 g for 30 min. 1.5 mL of the solubilized testis microsome were mixed with 1–2 μg antibody and 25 μL Protein A/G-bead slurry (Santa Cruz Biotechnology) at 4°C overnight. The IP products were finally eluted in 50 μL LDS loading buffer containing 50 mM DTT. Antibodies used for Western blotting were rabbit anti-mouse CatSperε (αm-ε968; 1.6 μg/mL), mouse CatSperζ (αm-ε174; 2.7 μg/mL), human CatSperε (αh-ε31; 2.7 μg/mL), human CatSperζ (αh-ζ11: 1 μg/mL). Monoclonal anti-phosphotyrosine (clone 4G10; 1 μg/mL), anti-Flag (clone M2; 1 μg/mL), anti-calmodulin (05–173, 1 μg/mL), and anti-acetylated tubulin (T7451,1: 20,000). Secondary antibodies were anti-rabbit IgG-HRP (1:10,000) and anti-mouse IgG-HRP (1: 10,000) from Jackson ImmunoResearch (West Grove, PA).
Sperm immunocytochemistry
Request a detailed protocolCaudal epididymal mouse sperm cells attached to glass coverslips were fixed in 4% paraformaldehyde (PFA) in PBS, permeabilized with 0.1% TrixonX-100 for 10 min. Human sperm cells from swim-up purification were fixed 4% PFA in PBS for 10 min followed by 100% MeOH. Fixed human sperm cells were permeabilized in 0.1% saponin for 10 min. Permeabilized sperm cells were washed in PBS and blocked with 10% goat serum for 1 hr. Mouse samples were stained overnight with primary antibody against CatSper1 (10 µg ml−1) and CatSperζ (mζ174, 20 µg ml−1) as were human samples with primary antibodies against CatSperε (hε31, 20 µg ml−1) and CatSperζ (hζ11, 10 µg ml−1), in 10% goat serum in PBS, 4°C. After PBS wash, goat-anti-rabbit Alexa488 conjugate (Invitrogen) served as the secondary antibody. Images were acquired on laser scanning confocal microscopes (Olympus Fluoview 1000; Figure 1G and H, Figure 1—figure supplement 2G, and Figure 2—figure supplement 1E and Leica TCS SP8; deconvolved image in Figure 5A).
Super-resolution imaging
3D STED imaging
Request a detailed protocolFor analysis of CatSper nanodomain organization, CatSper1 images were acquired with Leica TCS SP8 gated stimulated emission and depletion (STED 3×) microscopy using an HCX PL APO 100×/1.40 oil objective lens (Leica Microsystems, Germany). Samples were prepared as described in Sperm Immunocytochemistry with slight modifications. After incubation with primary antibody, cells were washed with PBS and incubated with goat anti-rabbit IgG coupled to Alexa Fluor 546 (Invitrogen, 1:100) for 1 hr at room temperature. Coverslips were mounted with Prolong Gold (Invitrogen) and cured for 24 hr before image acquisition. Within each experiment, identical settings for laser power, STED power, and gating were used to acquire images. The wavelength of the STED depletion laser was 660 nm and was adjusted to 50% of power. z-stacks of 17 optical sections with a step size of 0.1 μm were deconvolved using Huygens Software.
3D STORM imaging
Request a detailed protocol3D STORM experiments were performed as previously described (Chung et al., 2014). Imaging buffer was prepared in 60% (wt/wt) sucrose solution, increasing imaging depth to 1 µm (Chung et al., 2014). Imaging buffer was supplemented with 100 mM mercaptoethylamine (pH 8.5) as a switching agent as well as an O2 scavenger (5% glucose (wt/vol), 0.5 mg/ml glucose oxidase, and 40 mg/ml catalase) to reduce the rate of photobleaching. The sample was illuminated at 657 nm for imaging the photoswitchable reporter molecules (Alexa 647), and 405 or 532 nm for facilitating the activation of Alexa 647 from the dark state. For 3D localization, a cylindrical lens (focal length = 1 m) was inserted into the detection path to enable determination of z positions from the ellipticities of the molecular images and the x and y positions from the centroid positions (Huang et al., 2008). Image analysis and rendering was performed and angular profiles were constructed as previously described (Chung et al., 2014).
Fourier transform and autocorrelation
Request a detailed protocolFourier transform and autocorrelation analyses of 3D STORM images were performed as previously described (Xu et al., 2013; Zhong et al., 2014). A Fourier transform of the 1D projection localization distribution yielded a main peak that corresponds to a spatial period of ~800 nm for the CatSperz-/- spermatozoa. The autocorrelation curve for the CatSperz-/- spermatozoa showed a periodic modulation with the first peak at ∼850 nm.
Sperm migration assay and in vivo fertilization
Request a detailed protocolFor timed coitus, females were introduced to single-caged CatSperz-het or -null males for 1 hr and checked for the presence of a vaginal plug. To examine sperm migration to the fertilization site in vivo, ampullae were removed from the mated females at 8 hr after coitus and COCs were released. A series of z-stacked images (2 µm step size) of the COCs was taken and number of sperm within each COC was recorded according to the presence of a sperm head. To calculate in vivo fertilization rate, eggs were gently flushed from oviducts and ampullae from the mated females at 20 hr and 27–30 hr after coitus. The total number of eggs and the number of 2 cell eggs were counted.
Fertility test and in vitro fertilization
Request a detailed protocolTwo females were caged with each male for three months to track pregnancy and litter production. For IVF assays, oocytes were recovered from superovulated 5–6-week-old B6D2F1 female mice 13 hr after injection of 5 U human chorionic gonadotropin. For standard IVF, sperm were collected from the cauda epididymis. For ejaculate IVF, sperm were retrieved from the uterus of a 1 hr window-timed coitus. Both epididymal and ejaculated sperm were capacitated in vitro at 37°C for 1 hr, and coincubated with eggs at ∼105 sperm/mL. After about 4.5 hr, unbound sperm were washed away. After 24 hr incubation the embryos were observed under light microscopy (Olympus IX-70) to check for development of the two-cell stage.
Flagellar waveform analysis
Request a detailed protocolSpermatozoa from the dissected cauda epididymis (swim up method) were collected in HEPES buffered saline (HS) media. Spermatozoa were plated on 35 mm fibronectin-coated coverslips for 15 min (22°C); unattached sperm were removed by the gentle pipette wash (time 0) and basal motility recorded. Activated motility was recorded within the first 10 min after adding pre-warmed human tubal fluid (HTF)-capacitating medium (Millipore). To induce hyperactivation, attached sperm cells were incubated in HTF media for 90 min at 37°C (5% CO2). All subsequent images were recorded at 37°C. The flagellar waveform was analyzed by stop-motion digital imaging collected at 200 fps (HC Image software, Hamamatsu Photonics or Zen Blue, Zeiss; 2 s movies). Overlay of flagellar traces from two complete flagellar beats were generated by hyperstacking binary images using open-source FIJI software (Schindelin et al., 2012) and time coded in color.
Sperm motility analysis
Request a detailed protocolCauda epididymal spermatozoa were suspended and incubated in non-capacitating M2 medium (Specialty Media, Millipore) or in HTF medium for capacitation. Sperm motility was then measured using the IVOS sperm analysis system (Hamilton Thorne Biosciences, Beverly, MA) in an 80 µm (depth) chamber to obtain various parameters (Figure 2—figure supplement 2D). Sperm motility was also analyzed with an Olympus IX-70 microscope equipped with a high-speed sCMOS camera (Orca-Flash4.0) and a 10x objective. 1–2 × 105 mouse sperm before and after capacitation were added to the 37°C chamber (Delta T culture dish controller; Bioptechs) containing 1 ml HEPES-HTF medium (H-HTF: 92 mM NaCl, 2 mM CaCl2, 4.7 mM KCl, 0.2 mM MgCl2, 0.37 mM KH2PO4, 25 mM NaHCO3, 18.3 mM Na lactate, 2.78 mM glucose, 0.33 mM Na pyruvate, 0.4% [w/v] bovine serum albumin [BSA], and 10 mM HEPES [pH 7.4]). In some experiments, the medium was supplemented with methylcellulose (MC) (M0512, 4000 cP in 2% solution; Sigma) at 0.3%, 0.4%, or 0.5% (w/v). Sperm swimming 3–5 mm from the rim were recorded after a 10 min preincubation period that allowed spontaneous dissociation of sperm clumps. To inhibit convective flow, 1 ml of medium was overlaid by 1 ml of mineral oil and covered by a heated glass lid (Bioptechs). Sperm motility at 37°C was videotaped at 100 fps. Images (HC Image software, Hamamatsu Photonics) were analyzed for swimming trajectory from a 1 s playback movie at 1/5 speed, by head tracing via Computer Assisted Sperm Analysis (CASA; http://rsbweb.nih.gov/ij/plugins/casa.html). To track swimming trajectory in viscous medium, the sperm motility was videotaped at 50 fps. The images were analyzed using Fiji software (Schindelin et al., 2012) by assembling overlays of the flagellar traces generated by hyperstacking binary images of 20 frames of 2 s movies coded in a gray intensity scale.
In-capillary sperm rheotaxis
Request a detailed protocolMouse sperm incubated in HTF medium for 90 min at 2 × 106/ml yielded capacitated sperm. Capacitated sperm were transferred and concentrated for capillary loading by centrifugation at 900 g for 3 min. The loose sperm pellet at the bottom of the microcentrifuge tube was resuspended in HEPES-HTF at 4 × 106/ml, and loaded into the capillary by suction via an air-pressure microinjector (IM-5B; Narishige; 22 C, ~200 µm/s). While applying gentle positive pressure, the sperm in the tip of the capillary were moved out of the sperm drop. The tip of the capillary is transferred to a 37°C chamber (Delta T culture dish controller; Bioptechs) and placed into a 50 µl drop of HEPES-HTF medium covered with mineral oil. Negative pressure was applied slowly and sperm cells swimming against the flow and down to the H-HTF drop was video-recorded at 33 fps.
Electrophysiological recording of mouse spermatozoa
Request a detailed protocolWhole-cell recording of corpus epididymal spermatozoa from 3–5 month-old CatSperz+/- or CatSperz-/- mice was performed blind as to genotype (Kirichok et al., 2006; Navarro et al., 2011). HS was the bath medium. The standard pipette solution was (mM): 120 Cs- Methanesulfonate (Cs-MeSO4), 5 CsCl, 5 Cs-BAPTA, 10 HEPES and 10 MES, pH 7.2 with H-MeSO3. To record IATP, we used a low Cl- bath solution (to reduce background Cl- conductance) in the following (mM): 150 Na- methanesulfonate (Na-MeSO3), 2 CaCl2, 10 Na-HEPES, and 10 MES (pH 7.4 or 6.0). To measure ICatSper, we used divalent-free (DVF) solution, in mM: 150 Na-MeSO3, 2 Na3HEDTA [(hydroxyethyl)ethylenediaminetriacetic acid], 2 EGTA, and 20 HEPES (pH 7.4) with NaOH. Solutions were applied to sperm cells (lifted from the coverslips) initially by bath perfusion. After break-in, the access resistance was 25–80 MΩ. All experiments were performed at 22–24°C. The whole-cell currents were recorded using an Axopatch 200B amplifier (Molecular Devices, Sunnyvale, CA), acquired with Clampex 9 (pClamp9 Software; Molecular Devices), and analyzed with Origin software (OriginLab). Signals were low-pass filtered at 2 kHz and sampled at 10 kHz. Data are given as mean ± SD.
Quantification and statistical analysis
Request a detailed protocolAll the experiments are repeated at least three times. Sample size and number of replicates are described in each figure and the figure legends. Statistical analyses were performed using Student’s t-test unless indicated; e.g. F-test in one-way ANOVA. Differences were considered significant at *p<0.05, **p<0.01, ***p<0.001, and ****p<0.0001. When ****p<0.0001, actual P value is not indicated.
References
-
A non-selective cation conductance in frog muscle membrane blocked by micromolar external calcium ionsThe Journal of Physiology 353:565–583.https://doi.org/10.1113/jphysiol.1984.sp015351
-
Early evolution of the eukaryotic Ca2+ signaling machinery: conservation of the CatSper channel complexMolecular Biology and Evolution 31:2735–2740.https://doi.org/10.1093/molbev/msu218
-
Two distinct Ca(2+) signaling pathways modulate sperm flagellar beating patterns in miceBiology of Reproduction 85:296–305.https://doi.org/10.1095/biolreprod.110.089789
-
Phosphoglycerate kinase 2 (PGK2) is essential for sperm function and male fertility in miceBiology of Reproduction 82:136–145.https://doi.org/10.1095/biolreprod.109.079699
-
Hyperactivated sperm progress in the mouse oviductBiology of Reproduction 46:779–785.https://doi.org/10.1095/biolreprod46.5.779
-
CatSper-null mutant spermatozoa are unable to ascend beyond the oviductal reservoirReproduction, Fertility and Development 21:345–350.https://doi.org/10.1071/RD08183
-
Quantitative analysis of flagellar movement in hyperactivated and acrosome-reacted golden hamster spermatozoaMolecular Reproduction and Development 61:376–384.https://doi.org/10.1002/mrd.10017
-
CatSperbeta, a novel transmembrane protein in the CatSper channel complexThe Journal of Biological Chemistry 282:18945–18952.https://doi.org/10.1074/jbc.M701083200
-
Rheotaxis guides mammalian spermCurrent Biology : CB 23:443–452.https://doi.org/10.1016/j.cub.2013.02.007
-
Biphasic role of calcium in mouse sperm capacitation signaling pathwaysJournal of Cellular Physiology 230:1758–1769.https://doi.org/10.1002/jcp.24873
-
Mechanisms of fertilization elucidated by gene-manipulated animalsAsian Journal of Andrology 17:646–652.https://doi.org/10.4103/1008-682X.153299
-
Fiji: an open-source platform for biological-image analysisNature Methods 9:676–682.https://doi.org/10.1038/nmeth.2019
-
Sperm motility hyperactivation facilitates penetration of the hamster zona pellucidaBiology of Reproduction 53:1280–1285.https://doi.org/10.1095/biolreprod53.6.1280
-
The roles of evolutionarily conserved functional modules in cilia-related traffickingNature Cell Biology 15:1387–1397.https://doi.org/10.1038/ncb2888
-
The rapid evolution of reproductive proteinsNature Reviews Genetics 3:137–144.https://doi.org/10.1038/nrg733
-
Mammalian sperm proteins are rapidly evolving: evidence of positive selection in functionally diverse genesMolecular Biology and Evolution 19:1973–1980.https://doi.org/10.1093/oxfordjournals.molbev.a004021
Article and author information
Author details
Funding
Howard Hughes Medical Institute
- Xiaowei Zhuang
- David E Clapham
Yale School of Medicine (Goodman-Gilman Yale Scholar Award 2015-08)
- Jean-Ju Chung
The funders had no role in study design, data collection and interpretation, or the decision to submit the work for publication.
Acknowledgements
We thank M. Sanguinetti for testing CatSper expression in Xenopus oocytes for ICatSper detection, P. DeCaen for help with sperm electrophysiology, and L. Ded for calculating rib length from the literature and critical reading of the manuscript-in-progress. This work was supported by Goodman-Gilman Yale Scholar Award 2015–08 (J-JC), HHMI (XZ), and HHMI (DEC).
Ethics
Animal experimentation: This study was performed in strict accordance with the recommendations in the Guide for the Care and Use of Laboratory Animals of the National Institutes of Health. All the mice were treated in accordance with guidelines approved by the Boston Children's Hospital (13-01-2341R) and Yale (2015-20079) Animal Care and Use Committees (IACUC).
Copyright
© 2017, Chung et al.
This article is distributed under the terms of the Creative Commons Attribution License, which permits unrestricted use and redistribution provided that the original author and source are credited.
Metrics
-
- 5,102
- views
-
- 997
- downloads
-
- 138
- citations
Views, downloads and citations are aggregated across all versions of this paper published by eLife.
Citations by DOI
-
- 138
- citations for umbrella DOI https://doi.org/10.7554/eLife.23082