A recurrent regulatory change underlying altered expression and Wnt response of the stickleback armor plates gene EDA
Abstract
Armor plate changes in sticklebacks are a classic example of repeated adaptive evolution. Previous studies identified ectodysplasin (EDA) gene as the major locus controlling recurrent plate loss in freshwater fish, though the causative DNA alterations were not known. Here we show that freshwater EDA alleles have cis-acting regulatory changes that reduce expression in developing plates and spines. An identical T → G base pair change is found in EDA enhancers of divergent low-plated fish. Recreation of the T → G change in a marine enhancer strongly reduces expression in posterior armor plates. Bead implantation and cell culture experiments show that Wnt signaling strongly activates the marine EDA enhancer, and the freshwater T → G change reduces Wnt responsiveness. Thus parallel evolution of low-plated sticklebacks has occurred through a shared DNA regulatory change, which reduces the sensitivity of an EDA enhancer to Wnt signaling, and alters expression in developing armor plates while preserving expression in other tissues.
https://doi.org/10.7554/eLife.05290.001eLife digest
Stickleback fish develop bony plates on their surface to protect themselves from predators. The extent and pattern of their bony armor depends on their habitat: marine sticklebacks are typically covered from head to tail with bony plates, but freshwater sticklebacks retain only a few plates on their sides.
One gene that promotes the formation of the bony plates is called ectodysplasin (EDA). This encodes a signaling protein that is important for the development of the skeleton, skin and many other tissues. Variations in the sequence of this gene are shared among different stickleback populations worldwide. However, it has not been clear which genetic changes can explain how lightly armored freshwater sticklebacks could have evolved from their well-armored marine ancestors on several separate occasions.
Here, O'Brown et al. studied EDA in marine and groups of freshwater sticklebacks that have evolved in different locations around the world. The experiments show that the level of expression of EDA in the developing plates and spines is lower in the freshwater fish. O'Brown et al. thought this could be due to genetic changes in regions of EDA that lie outside the region that encodes the protein, so called ‘regulatory elements’.
Indeed, further experiments found that all freshwater fish have a small change in the DNA of a regulatory element that switches on the gene in plate-forming regions of the body. When this change was introduced into marine sticklebacks, the fish had lower levels of gene expression in these plate-forming regions.
These findings demonstrate that lightly armored sticklebacks have evolved multiple times from their well-armored marine ancestors through the same small change in their DNA that alters the expression of the EDA gene. The next challenge will be to understand why this particular small change in DNA appears to be favored over all the other changes that could occur in the regulatory element, and to see if factors that act through this regulatory switch also modify armor structures in natural populations.
https://doi.org/10.7554/eLife.05290.002Introduction
The repeated evolution of similar adaptive phenotypic traits in multiple populations is a fascinating evolutionary phenomenon observed in many organisms (Wood et al., 2005; Elmer and Meyer, 2011; Conte et al., 2012; Martin and Orgogozo, 2013; Stern, 2013). The threespine stickleback (Gasterosteus aculeatus) is a particularly favorable species to characterize the molecular mechanisms underlying repeated evolution of adaptive phenotypic traits in nature, because many populations have evolved similar morphological and skeletal traits following widespread colonization of new freshwater environments by migratory marine ancestors at the end of the last ice age (Bell and Foster, 1994).
One of the most striking and ubiquitous morphological changes seen in sticklebacks is repeated alteration in bony armor seen along the sides of fish. Marine sticklebacks are typically covered from head to tail with 32 (or more) bony lateral plates. In contrast, freshwater fish characteristically lack most plates, typically retaining only 0–7 plates in the anterior flank region. This dramatic difference in anterior-posterior patterning of armor plates was used to assign different species names to marine and freshwater sticklebacks in the 1800s (Cuvier and Valenciennes, 1829). Subsequent studies have shown that different armor patterns are highly heritable, and are likely controlled by a relatively simple genetic system (Münzing, 1959; Hagen and Gilbertson, 1973; Avise, 1976; Ziuganov, 1983; Banbura, 1994). More recently, genome-wide linkage mapping in crosses between divergent sticklebacks identified a major locus on stickleback chromosome IV that controls over 75% of the variance in armor plate number in F2 offspring (Colosimo et al., 2004; Cresko et al., 2004), as well as several unlinked modifier genes that each control 5–10% of the variance in plate numbers (Colosimo et al., 2004).
High-resolution mapping, chromosome walking, and transgenic rescue experiments showed that the major armor plate locus corresponds to the ectodysplasin (EDA) gene on stickleback chromosome IV (Colosimo et al., 2005). The EDA gene encodes a secreted protein in the tumor necrosis factor (TNF) family that plays a key role in cell signaling during the development of multiple neural crest and ectodermal tissues, including skin, hair, and teeth (Mikkola and Thesleff, 2003; Cui and Schlessinger, 2006). Humans with null mutations in EDA have defects in multiple ectoderm and neural crest derived tissues, including sparse hair, absent sweat glands, dental abnormalities, and dermal bone changes in the skull (Mikkola and Thesleff, 2003; Cui and Schlessinger, 2006; Yavuz et al., 2006; Clauss et al., 2008; Lesot et al., 2009). Zebrafish and medaka mutants with perturbations in the EDA pathway display severe skeletal abnormalities, such as loss of fins and scales, missing and abnormally shaped teeth, and abnormal craniofacial morphology (Harris et al., 2008; Iida et al., 2014).
While both high-resolution mapping and transgenic rescue experiments confirm that EDA is the major locus controlling armor plates in sticklebacks, the molecular difference between marine and freshwater fish is still unclear. Most freshwater populations share four amino acid differences in the EDA gene, as well as numerous non-coding changes that together make up a characteristic freshwater haplotype (Colosimo et al., 2005). However, the four amino acid changes occur at positions that also vary among other species, so these coding changes are unlikely to be the basis of major changes in EDA function. In addition, there exists at least one low-plated stickleback population that has the identical EDA protein-coding sequence as marine fish (Colosimo et al., 2005). This key population from Nakagawa Creek in Gifu, Japan (NAKA) is a low-plated stream population with a predominately marine-like sequence in both coding and non-coding regions. NAKA fails to complement armor plate changes when crossed with a typical Canadian low-plated population (Schluter et al., 2004), suggesting that NAKA and other low-plated fish share a modification in the same major locus. Based on the absence of amino acid changes in NAKA, and the deleterious nature of coding region changes in human patients, Colosimo et al. (2005) proposed that an unknown regulatory change at the stickleback EDA locus is the most likely basis of the common EDA variants found in freshwater fish.
Here we further investigate the EDA locus in order to study the causative base pair changes that underlie repeated evolution of low-plated sticklebacks.
Results
A cis-acting regulatory change reduces expression of freshwater EDA gene
In order to test if EDA is differentially expressed in marine and freshwater fish due to cis-regulatory differences, we performed allele-specific expression in F1 hybrid fish made by crossing marine and freshwater sticklebacks. The F1 hybrids are heterozygous for both the marine and freshwater haplotypes at the EDA locus, and therefore express both alleles in an identical trans-acting environment. We then isolated RNA from 10 different developing tissues, and determined whether the freshwater and marine EDA transcripts were expressed at the same or different levels using pyrosequencing (Figure 1, see ‘Materials and methods’). No significant expression differences between marine and freshwater EDA alleles were observed in the fins or the lower jaw. However, the freshwater EDA allele was expressed almost fourfold lower than the marine allele in the developing anterior and posterior flanks (corresponding to sites where armor plates had already appeared, or were not forming yet; respectively), and in the dorsal and pelvic spines (p < 0.01, Student's t test), as well as twofold lower in the premaxilla (p < 0.05, Student's t test). These data suggest that the marine and freshwater haplotypes at the EDA locus have cis-acting regulatory changes that reduce expression of the freshwater allele in particular tissues, including the flank regions where armor plates normally form.
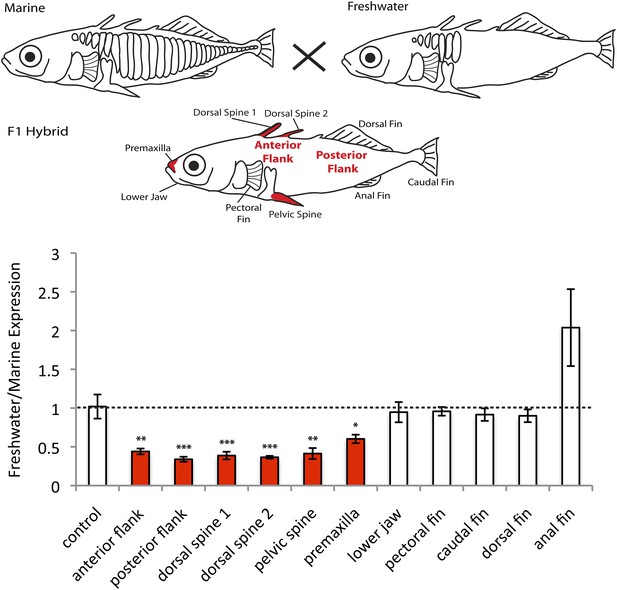
EDA shows allele-specific expression differences in several tissues, indicating cis-regulatory divergence.
Allele-specific expression in F1 freshwater-marine heterozygous larvae reveals significant differential expression of the marine and freshwater alleles in dorsal spines 1 and 2, the pelvic spine, the premaxilla, and the presumptive armor plates (anterior and posterior flanks). In all of these bony tissues the marine allele of EDA is expressed more highly than the freshwater allele, suggesting that there are differences in the cis-regulatory sequences controlling EDA expression. Several other tissues, however, do not show significant allelic imbalance in EDA expression; their allelic ratios are close to 1 (dashed line). The control shows results from a 1:1 mixture of plasmids containing the freshwater and marine alleles. Red-shaded structures and bars indicate tissues with significant allelic-imbalance compared to control (***p < 0.001, **p < 0.01, *p < 0.05 by two-tailed t-test).
Identification of a single base pair change shared by all low-plated fish
Previous studies narrowed the minimal candidate interval controlling armor plates to a 16 kb interval containing EDA and flanking regions (Colosimo et al., 2005). To look for possible shared molecular changes that might account for the regulatory difference between marine and freshwater sticklebacks, we amplified and sequenced the EDA candidate interval from low-plated Japanese NAKA fish, and compared it to other high- and low-plated stickleback populations (Figure 2 and ‘Materials and methods’). This analysis identified a single T → G nucleotide change, located at position chrIV:12811481 (gasAcu1 assembly, Jones et al., 2012) in the intergenic region downstream of EDA, that was shared between NAKA and all other low-plated sticklebacks examined.
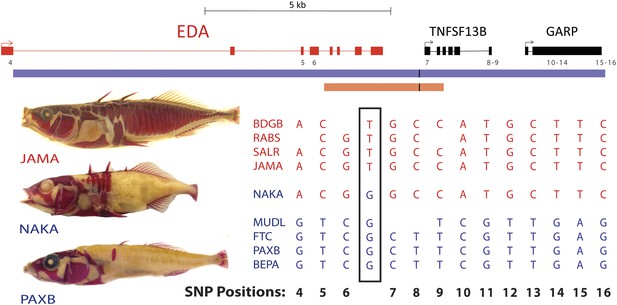
All low-plated populations share a single base pair change in the genetic region controlling armor plates.
Genome-wide comparisons of low- and high-plated fish reveal a T → G base pair change (black box) that is shared between all low-plated populations tested, including the low-plated Japanese NAKA fish that otherwise shows a primarily marine-like haplotype in the EDA region. Geographic population codes and DNA sequences from marine high-plated populations and freshwater low-plated populations are shown in red and blue, respectively, along with representative Alizarin Red stained fish showing typical armor plate patterns in different fish. The 16 kb candidate interval controlling armor plate number (blue bar, Colosimo et al., 2005) is shown beneath predicated genes in the region. Also shown are the numbered positions (4–16) of previously identified SNPs that differentiate most low- and high-plated sticklebacks other than NAKA (Colosimo et al., 2005). These numbered SNPs correspond to positions chrIV: 12800508, 12808303, 12808630, 12811933, 12813328, 12813394, 12815024, 12815027, 12816201, 12816202, 12816360, 12816402, and 12816464 in the stickleback genome assembly (Jones et al., 2012). Blank positions represent occasional gaps in sequence coverage for individual fish from large population surveys (Colosimo et al., 2005; Jones et al., 2012). The position of the shared T → G change (chrIV:12811481) is indicated with a short black vertical line in the overall genomic interval, and in a 3.2 kb region that was used to test for possible regulatory enhancers in the EDA region (orange bar, chrIV:12808949–12812120).
The low-plated base pair change alters the activity of an EDA enhancer
Given the known role of EDA in plate formation, we hypothesized that this intergenic base pair change (Figure 2) lies in a developmental enhancer that modulates EDA gene expression during armor plate development. Therefore, we cloned a 3.2 kb region surrounding the SNP (orange bar, Figure 2) from high-plated marine fish and tested for enhancer activity using a GFP reporter construct (p3.2mar-GFP, see ‘Materials and methods’). In two-month-old transgenic fish, this 3.2 kb region drives consistent GFP expression at multiple sites, including the anterior and posterior armor plates, the junction between the pelvic spine and girdle, the upper edge of the pelvic girdle, the base of the pectoral fin, the cranial ganglia surrounding the eyes and lips, and the premaxilla and jaw (Figure 3, Figure 4A,B). Comparison to the endogenous pattern of EDA expression using in situ hybridization suggests that the GFP construct recapitulates typical EDA patterns in cranial ganglia, premaxilla, jaw, pectoral fin base, armor plates, and pelvic girdle base (Figure 3). However, some domains of endogenous EDA expression are not accounted for by the enhancer region, including the dorsal and pelvic spines, suggesting that this construct contains some but not all of the regulatory information controlling EDA expression during normal development.
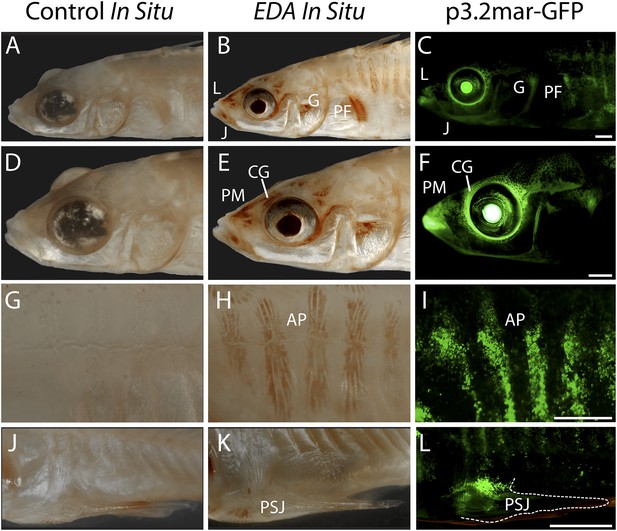
Reporter expression driven by an EDA enhancer matches several regions of endogenous EDA expression.
(A, D, G, J) Negative control DapB RNAscope in situ staining shows no positive brown signal appearing around the face (A and D), the plates (G), or the pelvic junction (J). The slight brown color in the pelvic spine is due to natural pigmentation at this site. (B, E, H, K) Endogenous EDA expression is localized to the premaxilla, lips, lower jaw, cranial ganglia, gill and pectoral fin base (B and E); armor plates (H); and the junction between the pelvic spine and the pelvic girdle (K). (C, F, I, L) The p3.2mar-GFP construct drives reporter expression at several corresponding sites, including the lips, premaxilla, lower jaw and cranial ganglia surrounding the eyes (C and F); in the armor plates; (I) and at the pelvic junction (L). Anatomical abbreviations as in other figures, including: lips (L), premaxilla (PM), lower jaw (J), cranial ganglia (CG), gills (G), pectoral fin base (PF), anterior plates (AP), and pelvic spine junction (PSJ). Scale bars are 1 mm long.
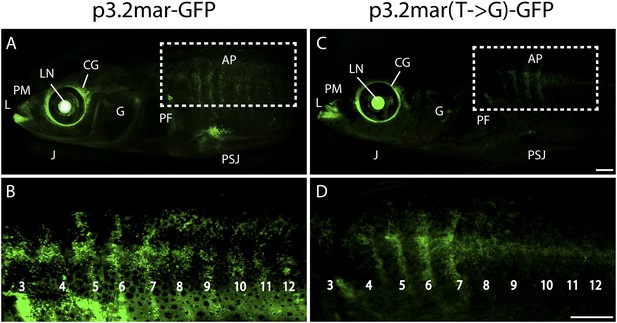
Enhancer expression in plates and other structures is reduced by a single base pair change.
(A, B) A 3.2 kb enhancer region from high-plated fish drives GFP expression in all armor plates (AP) of 2-month-old (20 mm long) marine stickleback larvae, with expression preceding plate ossification, and stronger expression in the first 7 armor plates. The p3.2mar-GFP construct also drives expression in the lips (L), premaxilla (PM), lower jaw (J), cranial ganglia (CG), the base of the pectoral fins (PF), and the pelvic spine-girdle junction (PSJ). Panel B is a higher magnification view of the area boxed in panel A. (C, D) The single base pair change in the p3.2mar(T → G)-GFP construct results in greatly reduced enhancer activity in the posterior plates, and reduced but detectable expression in plates 4–7 (D). This stable line also retains expression in the cranial ganglia and lips, reduced expression in the pelvic junction and the pectoral fin base, and novel strong expression in the spinal cord. Panel D is a higher magnification view of the area boxed in panel B. The hsp70 promoter in the GFP vector drives strong expression in the lens (LN) of all transgenic fish, helping to identify carriers following microinjection experiments (Chan et al., 2010). Scale bars are 1 mm long.
We next performed site-directed mutagenesis to change the T found in high-plated fish to the G found in all sequenced low-plated fish, while maintaining the sequence of the high-plated marine haplotype throughout the rest of the enhancer construct. The p3.2mar(T → G)-GFP plasmid still drove detectable expression in the anterior plates, cranial ganglia, jaws, and pectoral fin base, but showed greatly reduced GFP expression in the posterior armor plates and pelvic girdle junction (Figure 4C,D, Figure 4—figure supplement 1). Thus, the single base pair change shared by all low-plated sticklebacks produces striking but localized differences in gene expression, with prominent reduction occurring in the flank region where plates normally develop in marine fish.
Altered Wnt responsiveness of the marine and freshwater EDA enhancer
Previous studies have shown that Wnt signaling acts upstream of EDA in the early proliferation and specification of tissues in many vertebrates (Laurikkala et al., 2002; Cui and Schlessinger, 2006; Häärä et al., 2011; Arte et al., 2013). To test whether Wnt also acts upstream of plate development in sticklebacks, we tested whether implants of either Wnt-3a or Dkk-1 (an inhibitor of Wnt signaling, Glinka et al., 1998) altered normal patterns of armor plate formation. Beads soaked in PBS, Wnt-3a, or Dkk-1 proteins were surgically implanted into the mid-flank of 2-month-old marine fish, and fish were then aged to 6 months to test for effects on plate size and number. Control bead implantation had no significant effect on overall plate morphology (Figure 5A,B). In contrast, exposure to ectopic Wnt signaling at the juvenile stage induced hypermorphic plate development, characterized by adult fish with larger plates and plate fusions surrounding the sites of Wnt-3a bead implantation (Figure 5C). Conversely, the addition of the Wnt inhibitor Dkk-1 resulted in a hypomorphic phenotype marked by the absence of plates surrounding the bead implantation site (Figure 5D), suggesting that Wnt signaling plays an important role in normal plate development.
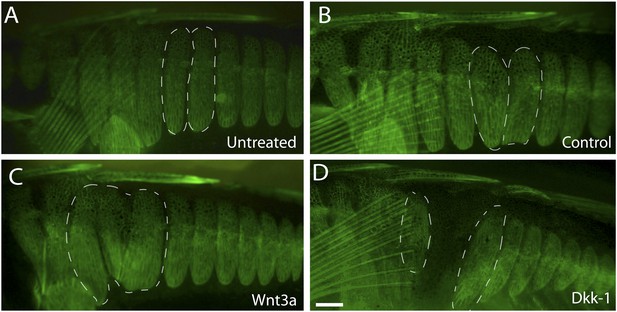
Wnt signaling regulates armor plate development.
Live Calcein staining of 6-month-old fish marks newly ossified bones in green. (A) Armor plates in an untreated high-plated adult marine fish. The normal morphologies of two individual plates are outlined with dashed lines. (B) Control beads soaked in PBS were implanted between the two outlined plates at two months of age. After bead implantation, fish continued to develop a full set of armor plates, with minimal changes in plate morphology (n = 8). (C) Implantation of Wnt-3a beads results in hypermorphic growth and armor plate fusion in the regions surrounding the exogenous Wnt-3a signal (n = 11). (D) Conversely, beads soaked in the Wnt inhibitor Dkk-1 inhibit plate formation surrounding the site of bead implantation (n = 10). Scale bar in D is 2 mm long.
To examine whether ectopic Wnt signaling also causes changes in EDA expression, we placed Wnt-3a protein beads into marine fish and used in situ hybridization to visualize EDA expression 48 hr later (Figure 6A,B). These experiments revealed a strong ring of induced EDA expression surrounding the site of Wnt-3a bead implantation (Figure 6B). We then implanted Wnt-3a beads into a stable transgenic line carrying the p3.2mar-GFP reporter construct described above. Implanted Wnt-3a beads, but not control beads, induced a strong ring of GFP expression directly around the site of bead implantation (Figure 6C,D). In contrast, implantation of Wnt-3a protein beads failed to produce a similar strong ring of GFP expression in transgenic fish carrying the mutated p3.2mar(T → G)-GFP construct (Figure 6E,F). Unexpectedly, the p3.2mar(T → G)-GFP construct did show a novel GFP response to the cyanoacrylate glue used in the implantation procedure, which was not seen in fish carrying p3.2mar-GFP. This expression was also observed in control manipulations with PBS beads (Figure 6E) or cyanoacrylate glue alone (data not shown), and was therefore distinct from the strong Wnt-3a response observed only with the fish carrying the p3.2mar-GFP construct.
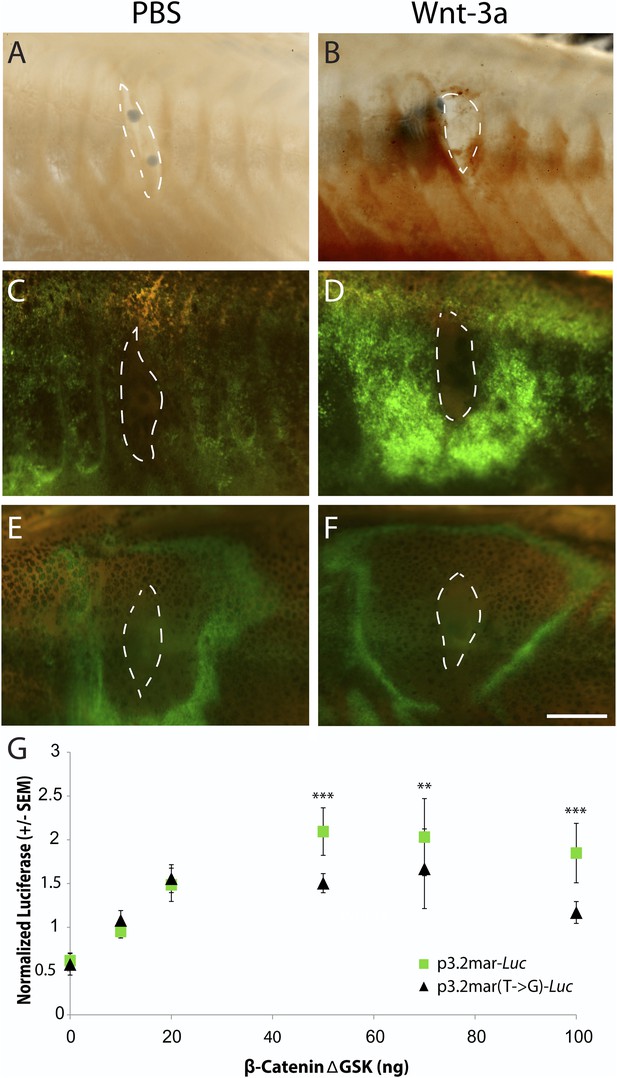
Single point mutation alters Wnt responsiveness of the EDA plate enhancer.
Beads soaked in either PBS or Wnt-3a protein were implanted in the flanks of 2-month-old (24 mm long) marine fish. All images were taken at 48 hr post bead implantation. (A, B) RNAscope in situ hybridization for EDA expression induced by control bead placement (A) or Wnt-3a protein (B). The addition of Wnt-3a beads induces a ring of EDA expression (brown color in B) directly surrounding the implantation site. (C, D) Bead implantation into the stable p3.2mar-GFP transgenic fish line. Control beads fail to induce GFP activity (C), whereas Wnt-3a beads induce a strong GFP response, seen in a ring surrounding the bead implantation site (D). (E, F) Bead implantation into the stable p3.2mar(T → G)-GFP line of transgenic fish. A ring of GFP expression is only seen at a distance from the implantation site of either control (E) or Wnt-3a (F) beads, corresponding to the location where cyanoacrylate glue was placed following implantation. Strong expression immediately surrounding the bead is not seen with Wnt-3a beads, in contrast to the result seen with p3.2mar-GFP transgenic fish (compare panels F and D). Scale bar in F is 1 mm long. (G) In vitro analysis of enhancer response to Wnt signaling via β-catenin co-transfection shows a strong induction of p3.2mar-Luc (green squares) with 50 ng or more of β-catenin in human HaCaT keratinocyte cells. The β-catenin-responsiveness of the p3.2mar(T → G)-Luc is significantly lower (black triangles). Combined p-values were calculated using Meta-P (***p < 0.001, **p < 0.01).
Canonical Wnt signaling normally activates gene expression through changes in the activity of β-catenin (Logan and Nusse, 2004). Cotransfection of a β-catenin expression construct (pRK5-sk-βcatΔGSK) with the marine EDA enhancer driving a luciferase reporter (p3.2mar-Luc) produced a significant, dose-dependent increase in luciferase expression in cultured human keratinocytes in vitro (Figure 6G). Engineering the single SNP change in the marine enhancer (p3.2mar(T → G)-Luc) reduced but did not eliminate response to β-catenin in the heterologous system (28% lower expression with 50 ng of β-catenin, p < 0.001, n = 4).
Our combined experiments show that Wnt signaling can alter armor plate development and EDA expression in sticklebacks. The EDA enhancer region from high-plated sticklebacks also responds to Wnt signaling, while the single base pair mutation shared between NAKA and other low-plated sticklebacks significantly reduces Wnt responsiveness both in vivo and in vitro.
Discussion
Previous work has shown that repeated armor plate reduction in sticklebacks is due in large part to genetic changes in the EDA region, though the causative molecular lesion(s) remained unknown (Colosimo et al., 2005; Jones et al., 2012). Our allele-specific expression experiments show that the freshwater allele of EDA is expressed at lower levels than the marine allele in F1 hybrids, confirming prior suggestions that there were likely to be cis-acting regulatory differences between marine and freshwater EDA variants (Colosimo et al., 2005). In addition, we have now identified a specific enhancer region in the key armor plates region, shown that the marine version of this enhancer normally drives expression in developing armor plates, and identified a specific T → G base pair change within the enhancer that is shared by all sequenced low-plated freshwater fish. Experimental recreation of the T → G base pair change reduces both armor plate expression and Wnt responsiveness of the enhancer, suggesting that this specific DNA change is the likely causative regulatory lesion in the EDA locus that leads to low-plated phenotypes in sticklebacks.
Like other genes found to underlie major morphological differences between marine and freshwater fish (Shapiro et al., 2006; Miller et al., 2007; Chan et al., 2010), the EDA gene is a key developmental control gene that is essential for formation of multiple tissues. Mutations in the coding region of EDA in both zebrafish and medaka cause deleterious phenotypes at multiple body sites, including complete loss of scales, partial loss of fins and teeth, and multiple craniofacial abnormalities (Harris et al., 2008; Iida et al., 2014). In contrast, the T → G regulatory change we have identified in an EDA enhancer leads to partial loss of EDA expression, particularly in the posterior flank region (Figure 4). This regulatory change thus alters EDA expression at the same body site where freshwater fish lack body armor, while preserving important functions of EDA in other tissues. These results provide a new example of a specific regulatory change linked to morphological evolution in natural populations (Martin and Orgogozo, 2013), and add to growing evidence that regulatory changes are a predominant mechanism underlying adaptive evolution in sticklebacks (Jones et al., 2012) and other organisms (Wray, 2007; Carroll, 2008).
Our results also provide new insight into genomic mechanisms contributing to repeated evolution. Previous analyses identified a shared low-plated EDA haplotype that has been fixed in most low-plated Pacific and Atlantic freshwater populations, and that is also present at very low frequency in the heterozygous state in marine populations (Colosimo et al., 2005; Barrett et al., 2008; Bell et al., 2010). Thus, EDA has become a classic example of rapid parallel evolution based on a preexisting genetic variant that increases in frequency when marine populations colonize new freshwater environments (Stern, 2013). The current results suggest that repeated evolution of low-plated phenotypes might also result from independent mutations occurring in the EDA locus in different populations. In previous surveys, Japanese NAKA fish were the only low-plated freshwater population that did not share the same EDA haplotype as other freshwater populations (Colosimo et al., 2005). Our experiments show that NAKA and other freshwater sticklebacks share an identical T → G non-coding regulatory mutation that reduces expression of EDA specifically in developing posterior armor plates. Characteristic flanking SNPs are not shared between NAKA and other low-plated populations, suggesting that the same T → G mutation has likely occurred independently on two very different haplotypes.
Recurrent mutations can be due to a particular DNA sequence that has a high intrinsic mutation rate. For example, previous studies of pelvic reduction in sticklebacks suggest that a key pelvic enhancer repeatedly deleted in freshwater populations has sequence features shared with fragile sites in human chromosomes (Chan et al., 2010). Individual base pairs can also be prone to particular mutations. For example, C → T transition mutations are particularly common at CpG dinucleotides in mammalian genomes, due to a high rate of spontaneous deamination of methylated C residues (Mancini et al., 1997; Xia et al., 2012). In contrast, the recurrent regulatory mutation we have identified at the stickleback EDA locus is a T → G transversion substitution, one of the least common types of changes seen in large scale studies of spontaneous germ-line mutations in humans (Kong et al., 2012; Genome of the Netherlands Consortium, 2014) as well as in flies, worms, and yeast (Lynch, 2010).
It is possible that the shared T → G change arose not by independent mutation, but by extensive recombination or gene conversion from the typical freshwater EDA haplotype. Migratory marine populations include rare individuals that are heterozygous for both marine and freshwater haplotypes, which likely arise by repeated rounds of introgression of freshwater alleles into marine populations (Colosimo et al., 2005; Schluter and Conte, 2009). Sequence studies suggest that recombination can occur between typical marine and freshwater haplotypes, producing smaller and smaller blocks of sequence shared among most low-plated populations (Colosimo et al., 2005). In previous studies, the minimal shared freshwater region was approximately 16 kb, consisting of regions of both the EDA gene and two flanking genes involved in immune functions (Colosimo et al., 2005). However further recombination between marine and freshwater haplotypes could narrow this region further, conceivably approaching the size of a single base pair. For example, we have recently surveyed 263 migratory marine sticklebacks from Alaska and identified 12 completely plated individuals that are heterozygous for the T → G change in the EDA enhancer (minor allele frequency 2.3%). Analysis of flanking SNPs suggests one of these carriers is heterozygous for a larger characteristic freshwater haplotype, three are heterozygous for a much shorter freshwater haplotype, and eight are heterozygous at the T → G position but are marine-like at other characteristic flanking SNPs tested (Supplementary file 1). These data show that migratory marine populations can carry freshwater haplotypes of different sizes, including much smaller regions surrounding the key T → G regulatory change. Although most low-plated populations have clearly fixed a multi-kilobase haplotype surrounding EDA, the large size of this haplotype may reflect co-selection for additional phenotypes controlled by the closely linked genes (Colosimo et al., 2005). The geographically distant NAKA population is low-plated but shares only the T → G change, either because of an independent mutation, or because of fixation of a tiny fragment of the typical EDA haplotype. The NAKA population may be useful in the future for distinguishing the phenotypic effects of the isolated T → G regulatory change versus the larger EDA haplotype typically found in most low-plated sticklebacks.
The absence of a greater range of armor plate mutations at the EDA locus could be due to the relatively high frequency of a preexisting freshwater haplotype, whose frequency in migratory populations exceeds the rate of many spontaneous mutations. Alternatively, the T → G change could represent one of very few possible ways of producing a major change in armor plate patterns while still preserving other functions of the EDA gene. A constrained spectrum of mutations has been observed in other contexts involving very specific phenotypes. For example, nearly all patients with classic achondroplasia contain the same Gly380Arg (G → C) substitution in FGFR3 (Horton et al., 2007). This Gly380Arg substitution leads to a constitutively active FGF receptor that is thought to confer a selective advantage to spermatogonial cells (Tiemann-Boege et al., 2002; Choi et al., 2008). Identical amino acid substitutions in particular genes also underlie several examples of repeated evolution including insecticide resistance in insects (GABA), tetrodotoxin resistance in snakes (NaK-ATPase), C4 photosynthesis in plants (PEPC), and dark pigmentation in mice and birds (MC1R) (Stern, 2013). These and other well-studied cases typically involve particular amino acid changes that alter protein activity in specific ways, rather than completely ablating protein function. In contrast, few examples are known of identical recurrent base pair mutations in non-coding regulatory sequences (Martin and Orgogozo, 2013), though multiple cases are now being uncovered in large-scale sequencing surveys of replicate microbial evolution (Tenaillon et al., 2012; Blank et al., 2014). In a recent large-scale study of parallel temperature adaptation over 2000 generations, recurrent use of particular genes was at least 10 times more common than recurrent use of the same base pair changes within those genes (Tenaillon et al., 2012). Of the relatively rare recurrent base pair changes, those affecting protein-coding sequence also outnumbered those affecting non-coding intergenic sequence by nearly threefold. The T → G base pair change we have identified near EDA provides a rare example in vertebrates of a particular non-coding base pair change contributing to repeated adaptive evolution.
Our experiments also show that Wnt signaling acts upstream of EDA control sequences in armor plate patterning, and that the low-plated SNP reduces Wnt responsiveness of the EDA enhancer (Figures 5, 6). Although canonical Wnt signaling typically acts through the β-catenin and Lef transcription factors, the particular T → G base pair change we have identified does not alter a canonical Lef binding sequence. However, Wnt signaling is known to interact with multiple additional signaling and transcription factor pathways, and the T → G change does alter a predicted binding site for c-Jun in the marine sequence (Newburger and Bulyk, 2009), which can act in collaboration with Wnt signaling in chondrocyte dedifferentiation (Hwang et al., 2005), osteopontin promoter activation in mammary cells (El-Tanani et al., 2004), and complexes with β-catenin to bind the promoters of Wnt target genes both in mammalian cells and in zebrafish (Gan et al., 2008). There are seven base pair positions in the predicted marine c-Jun binding site, and 21 corresponding single bp mutations that could alter one of these bases. 19 of these potential mutations are predicted to eliminate c-Jun binding (Messeguer et al., 2002). Of these 19 mutations, the T → G change found in low- plated sticklebacks is the only mutation that is also predicted to create a new overlapping binding site for AP-2α in the low-plated sequence. AP-2α has been shown to inhibit Wnt signaling by complexing with APC/β-catenin (Li and Dashwood, 2004; Li et al., 2009b). A new binding site for AP-2α could contribute to the reduced Wnt responsiveness of the freshwater EDA gene, or may contribute to other novel expression patterns that are not yet understood (such as the enhanced cyanoacrylate response we have observed with the T → G mutated enhancer). Future experiments are needed to test whether c-Jun, AP-2α or other factors interact directly with the EDA enhancer of either marine or freshwater sticklebacks. However, the simultaneous loss and gain of specific binding sites is a good example of the type of dual molecular constraints that could limit the range of possible base pair substitutions found underlying adaptive regulatory evolution at the EDA locus.
Our findings that connect Wnt signaling, plate development, and EDA signaling in sticklebacks also suggest new candidates for trans-acting genetic factors that may modify armor plate number in evolving populations. Previous genetic studies have shown that while the majority of the variance (>75%) in armor plate number in stickleback crosses maps to the EDA locus, the remainder of the variance can be explained by multiple plate modifier loci located on other chromosomes (Colosimo et al., 2004). Interestingly, two of the three previously mapped armor plate modifier regions contain genes for members of the Wnt pathway: WNT11 on chromosome VII and β-catenin (CTTNB1) on chromosome X. Given the dramatic effects of Wnt signaling on armor plate development and EDA regulation (Figures 5, 6), these or other components of the Wnt signaling pathway are strong candidates for additional loci that may contribute to the adaptive fine-tuning of armor plate numbers that is known to occur in many low-plated populations (Hagen and Gilbertson, 1972, 1973; Moodie, 1972; Moodie et al., 1973; Bell and Haglund, 1978).
Materials and methods
Allele-specific expression
Request a detailed protocolAllele-specific expression differences were detected using pyrosequencing analysis of F1 hybrid fish as previously described (Wang and Elbein, 2007). In brief, a marine female from Rabbit Slough, AK was crossed to a freshwater benthic male fish from Paxton Lake, British Columbia to generate F1 hybrids that were heterozygous for a SNP in the EDA gene. Hybrid fish were raised to 13 mm standard length, a stage where the first few armor plates are forming in anterior tissues, but posterior plates have not yet formed. Multiple tissues were dissected, including: first dorsal spine, second dorsal spine, pelvic spines, pectoral fins, caudal fin, dorsal fin, anal fin, premaxilla with oral teeth, lower jaw (approximately the articular and dentary with oral teeth), left anterior flank skin between the second dorsal spine and pelvic spine (where anterior plates are forming), and left posterior flank skin between the dorsal fin and anal fin (where posterior plates will later form). RNA was prepared from dissected tissues using the TRI Reagent Protocol (Life Technologies, Carlsbad, CA). cDNA was synthesized using the Superscript III Supermix (Life Technologies) with random hexamer primers. A 183 bp product from the EDA gene was amplified using a biotinylated forward primer 5′-TCCACCAGAAGCGGGATACA-3′ and the reverse primer 5′-TTATGCCCCGGTTATCCTGTG-3′. Amplified products were sequenced using the primer 5′-TCTCCTCATGACCCTCT-3′, and the percentage of the two SNP alleles was calculated by EpigenDx, Inc. (Hopkinton, MA).
DNA sequence comparisons
Request a detailed protocolThe 16 kb EDA candidate interval from NAKA fish was amplified as several long PCR products and assembled using Sanger sequencing (GenBank entry KP164994). Alignment of the NAKA sequence with the complete sequence of the EDA region from Salmon River (SALR) marine and Paxton Benthic (PAXB) freshwater BAC clones (Colosimo et al., 2005); and the Bear Paw Lake (BEPA) reference genome (Jones et al., 2012); identified 13 positions where low-plated NAKA, PAXB, and BEPA differed from high-plated SALR fish. Reexamination of these positions in sequence reads from 21 marine and freshwater genomes (Jones et al., 2012) placed with SAMtools (Li et al., 2009a) against the BEPA reference genome, and resequencing of additional fish, identified the chrIV:12811481 position as shared among all low-plated sticklebacks examined. Population codes and source locations are as previously described (Colosimo et al., 2005; Jones et al., 2012).
The region surrounding the T → G base pair change was subsequently amplified from 263 fully plated migratory sticklebacks collected from Rabbit Slough, AK (RABS), using 5′-TTGACAAGTGATGTTCTCTGTGGCC-3′ and 5′-ATGTTGGAGCTGGCAGGAGGAGG-3′. All heterozygous carrier fish were then tested for the characteristic flanking SNPs previously found to distinguish most high-plated and low-plated haplotypes in previous studies (Colosimo et al., 2005). SNPs 5 and 6 at positions 12808303 and 12808630 were determined by amplifying and sequencing a genomic region using 5′-CAGAGGAGGTGAAACCGCACTTACA-3′ and 5′-TGGGAACGCGTCGACATTTGGGA-3′. SNP 7 at position 12811933 was called from the same genomic amplification used to recover the T → G regulatory change. SNPs 8 and 9 at positions 12813328 and 12813394 were determined by amplifying and sequencing a genomic region using 5′-GTGCCCAGGAGCTCTAGACTTGGC-3′ and 5′-TCTCACATCCGGCAGCGACAAGC-3′.
Plasmids
The plate enhancer region was amplified from genomic DNA of a marine fish from Salmon River, British Columbia using 5′-ATGTGGCCAGATAGGCCACATGGTGTGGGAGAGCAGTGATCG-3′ and 5′-ATGTGGCCTATCTGGCCATGTTGGAGCTGGCAGGAGGAGG-3′ primers that each contain SfiI linkers. The 3.2 kb amplified fragment was cloned into the SfiI site of the pT2HE GFP reporter vector (modified from Kawakami, 2007) to generate p3.2mar-GFP.
Site directed mutagenesis was performed on the p3.2mar-GFP plasmid to induce a single freshwater base pair change using two 40 bp overlapping primers 5′-AATTAGTTCCATCTTGAGAGGCAGAGAGAAGATGGTTCCT-3′ and 5′-AGGAACCATCTTCTCTCTGCCTCTCAAGATGGAACTAATT-3′. A 15-cycle PCR amplification using 50 ng of plasmid, 125 ng of primers, and Phusion polymerase was performed to induce the base pair change (Zheng et al., 2004). The resulting plasmid, p3.2mar(T → G)-GFP, was verified by DNA sequencing.
For cell culture experiments, the enhancer inserts from p3.2mar-GFP and p3.2mar(T → G)-GFP were excised from the pT2He plasmid using SfiI and cloned into the XhoI site of the pTA-Luc vector (Clontech Laboratories, Inc., Mountain View, CA), to generate p3.2mar-Luc and p3.2mar(T → G)-Luc. The β-catenin expression plasmid pRK5-sk-βcatΔGSK was a gift from the Nusse Lab.
Transgenic enhancer assays
Request a detailed protocolTransgenic sticklebacks were generated by microinjection of freshly fertilized eggs as previously described (Chan et al., 2010). Plasmids were co-injected with Tol2 transposase mRNA as described (Fisher et al., 2006; Wada et al., 2010). Mature Tol2 mRNA was synthesized by in vitro transcription using the mMessage mMachine SP6 kit (Life Technologies). All enhancer assays were performed on high-plated fish derived from Little Campbell River (British Columbia), Bodega Bay (California), or Rabbit Slough (Alaska). Microscopic observation for GFP expression was conducted with a MZFLIII fluorescent microscope (Leica Microsystems, Bannockburn, IL) using GFP2 filters and a ProgResCF camera (Jenoptik AG, Jena, Germany) to distinguish GFP expression from autofluorescence in pigmented fish. We generated stable lines by making crosses from mosaic founder animals.
Whole-mount RNAscope in situ hybridization
Request a detailed protocolTwo-month-old fish (20–24 mm standard length) were fixed in 4% paraformaldehyde overnight at 4°C, washed, and stored in methanol at −20°C for up to 6 months prior to in situ hybridization. Fish were rehydrated through a series of methanol/water washes (90%, 75%, 50%, 25%, 0), bleached in 6% hydrogen peroxide rocking at room temperature for up to 3 hr, and treated with 10 μg/ml Proteinase K in water rocking for 7.5 min in order to detect EDA signal. From this point, the RNAscope Brown Protocol was followed with an EDA probe designed by Advanced Cell Diagnostics (Hayward, CA) with two procedural modifications: Pretreatment 2 was performed at 40°C and the hybridization step with EDA probe was allowed to proceed overnight (Wang et al., 2012; Gross-Thebing et al., 2014).
Bead experiments
Request a detailed protocolAffi-Gel Blue Gel beads (BioRad Laboratories, Inc., Hercules, CA) were soaked overnight in PBS, 1.2 μg of recombinant human Wnt-3a (R&D Systems, Minneapolis, MN), or 1.2 μg of recombinant mouse Dkk-1 (R&D Systems). Marine-derived fish were raised to 20–24 mm standard length (first four armor plates present), anesthetized with Tricaine (0.017% wt/vol), and an average of 12 beads were placed into the flank of each fish, posterior to the apparent plates. Cyanoacrylate glue (Loctite Super Glue) was used to close the skin surrounding the implantation site. Fish were allowed to recover for 48 hr before further experimentation or to continue developing into adulthood. The beads' effects on overall plate development were analyzed in live adult fish using 0.2% Calcein in aquarium water to mark newly ossified bones as previously described (Kimmel et al., 2003; Wada et al., 2010).
Cell culture experiments
Request a detailed protocolHaCaT human keratinocyte cells (Boukamp et al., 1988) were cultured in DMEM supplemented with 10% FBS, 2 mM L-glutamine and 1% penicillin-streptomycin. Cells were seeded into 24-well plates at a density of 1 × 105 cells/well and transfected after 24 hr. 300 ng of p3.2mar-Luc or p3.2mar(T → G)-Luc plasmids were cotransfected together with 0–100 ng of pRK5-sk-βcatΔGSK using Lipofectamine 2000 (Life Technologies) according to manufacturer's protocol. After 6 hr of transfection, cell culture medium was replaced with standard medium supplemented with 2.8 mM calcium chloride (Sigma, St. Louis, MO). Cell lysates were collected after 48 hr and assayed using the Dual-Luciferase Reporter Assay System (Promega, Madison, WI) according to the manufacturer's instructions.
Data availability
References
-
Frequency of Ectodysplasin alleles and limited introgression between sympatric threespine stickleback populationsEnvironmental Biology of Fishes 89:189–198.https://doi.org/10.1007/s10641-010-9712-z
-
The predictability of molecular evolution during functional innovationProceedings of the National Academy of Sciences of USA 111:3044–3049.https://doi.org/10.1073/pnas.1318797111
-
Normal keratinization in a spontaneously immortalized aneuploid human keratinocyte cell lineThe Journal of Cell Biology 106:761–771.https://doi.org/10.1083/jcb.106.3.761
-
A germ-line-selective advantage rather than an increased mutation rate can explain some unexpectedly common human disease mutationsProceedings of the National Academy of Sciences of USA 105:10143–10148.https://doi.org/10.1073/pnas.0801267105
-
The probability of genetic parallelism and convergence in natural populationsProceedings Biological Sciences / The Royal Society 279:5039–5047.https://doi.org/10.1098/rspb.2012.2146
-
Parallel genetic basis for repeated evolution of armor loss in Alaskan threespine stickleback populationsProceedings of the National Academy of Sciences of USA 101:6050–6055.https://doi.org/10.1073/pnas.0308479101
-
EDA signaling and skin appendage developmentCell Cycle 5:2477–2483.https://doi.org/10.4161/cc.5.21.3403
-
Ets gene PEA3 cooperates with beta-catenin-Lef-1 and c-Jun in regulation of osteopontin transcriptionThe Journal of Biological Chemistry 279:20794–20806.https://doi.org/10.1074/jbc.M311131200
-
Adaptation in the age of ecological genomics: insights from parallelism and convergenceTrends in Ecology & Evolution 26:298–306.https://doi.org/10.1016/j.tree.2011.02.008
-
Nuclear Dvl, c-Jun, beta-catenin, and TCF form a complex leading to stabilization of beta-catenin-TCF interactionThe Journal of Cell Biology 180:1087–1100.https://doi.org/10.1083/jcb.200710050
-
Consequences of X-linked hypohidrotic ectodermal dysplasia for the human jaw boneFrontiers of Oral Biology 13:93–99.https://doi.org/10.1159/000242398
-
The sequence alignment/map format and SAMtoolsBioinformatics 25:2078–2079.https://doi.org/10.1093/bioinformatics/btp352
-
The Wnt signaling pathway in development and diseaseAnnual Review of Cell and Developmental Biology 20:781–810.https://doi.org/10.1146/annurev.cellbio.20.010403.113126
-
Rate, molecular spectrum, and consequences of human mutationProceedings of the National Academy of Sciences of USA 107:961–968.https://doi.org/10.1073/pnas.0912629107
-
Constitutively methylated CpG dinucleotides as mutation hot spots in the retinoblastoma gene (RB1)American Journal of Human Genetics 61:80–87.https://doi.org/10.1086/513898
-
Ectodysplasin signaling in developmentCytokine & Growth Factor Reviews 14:211–224.https://doi.org/10.1016/S1359-6101(03)00020-0
-
Biologie, Variabilität und Genetik von Gasterosteus Aculeatus L. (Pisces) Untersuchungen Im ElbegebietInternationale Revue Der Gesamten Hydrobiologie und Hydrographie 44:317–382.https://doi.org/10.1002/iroh.19590440119
-
UniPROBE: an online database of protein binding microarray data on protein-DNA interactionsNucleic Acids Research 37:D77–D82.https://doi.org/10.1093/nar/gkn660
-
Parallel evolution and inheritance of quantitative traitsThe American Naturalist 163:809–822.https://doi.org/10.1086/383621
-
Genetics and ecological speciationProceedings of the National Academy of Sciences of USA 106:9955–9962.https://doi.org/10.1073/pnas.0901264106
-
Parallel genetic origins of pelvic reduction in vertebratesProceedings of the National Academy of Sciences of USA 103:13753–13758.https://doi.org/10.1073/pnas.0604706103
-
The genetic causes of convergent evolutionNature Reviews Genetics 14:751–764.https://doi.org/10.1038/nrg3483
-
The observed human sperm mutation frequency cannot explain the achondroplasia paternal age effectProceedings of the National Academy of Sciences of USA 99:14952–14957.https://doi.org/10.1073/pnas.232568699
-
RNAscope: a novel in situ RNA analysis platform for formalin-fixed, paraffin-embedded tissuesThe Journal of Molecular Diagnostics 14:22–29.https://doi.org/10.1016/j.jmoldx.2011.08.002
-
Detection of allelic imbalance in gene expression using pyrosequencingMethods in Molecular Biology 373:157–176.https://doi.org/10.1385/1-59745-377-3:157
-
The evolutionary significance of cis-regulatory mutationsNature Reviews Genetics 8:206–216.https://doi.org/10.1038/nrg2063
-
Ectodermal dysplasia: Retrospective study of fifteen casesArchives of Medical Research 37:403–409.https://doi.org/10.1016/j.arcmed.2005.06.016
-
An efficient one-step site-directed and site-saturation mutagenesis protocolNucleic Acids Research 32:e115.https://doi.org/10.1093/nar/gnh110
Article and author information
Author details
Funding
National Science Foundation (NSF)
- Natasha M O'Brown
- Brian R Summers
Howard Hughes Medical Institute (HHMI)
- Shannon D Brady
- David M Kingsley
National Institute of General Medical Sciences (NIGMS) (T32 GM007790)
- Natasha M O'Brown
National Human Genome Research Institute (NHGRI) (3P50 HG002568)
- Felicity C Jones
- David M Kingsley
The funders had no role in study design, data collection and interpretation, or the decision to submit the work for publication.
Acknowledgements
We thank Seiichi Mori and Michael Bell for samples of NAKA and RABS fish; Tim Howes for the pT2HE plasmid; Roel Nusse's lab for the pRK5-sk-βcatΔGSK plasmid; Mike McLaughlin for fish care; and Roel Nusse, Will Talbot, Sarah McMenamin, Zach O'Brown and members of the Kingsley lab for useful discussions. This research was supported in part by a National Institutes of Health Training Grant (NIGMS T32 GM007790, NO'B), National Science Foundation Graduate Research Fellowships (NO'B, BS), and a NIH Center of Excellence in Genomic Science grant (NHGRI 3P50 HG002568, DMK). DMK is an investigator of the Howard Hughes Medical Institute.
Ethics
Animal experimentation: This study was performed in accordance with the recommendations in the Guide for the Care and Use of Laboratory Animals of the National Institutes of Health. All of the animals were handled according to approved institutional animal care and use committee (IACUC) protocols (#13834) of Stanford University, in animal facilities accredited by the Association for Assessment and Accreditation of Laboratory Animal Care International (AAALAC).
Copyright
© 2015, O'Brown et al.
This article is distributed under the terms of the Creative Commons Attribution License, which permits unrestricted use and redistribution provided that the original author and source are credited.
Metrics
-
- 5,286
- views
-
- 764
- downloads
-
- 106
- citations
Views, downloads and citations are aggregated across all versions of this paper published by eLife.
Citations by DOI
-
- 106
- citations for umbrella DOI https://doi.org/10.7554/eLife.05290