Functional evidence implicating chromosome 7q22 haploinsufficiency in myelodysplastic syndrome pathogenesis
Abstract
Chromosome 7 deletions are highly prevalent in myelodysplastic syndrome (MDS) and likely contribute to aberrant growth through haploinsufficiency. We generated mice with a heterozygous germ line deletion of a 2-Mb interval of chromosome band 5A3 syntenic to a commonly deleted segment of human 7q22 and show that mutant hematopoietic cells exhibit cardinal features of MDS. Specifically, the long-term hematopoietic stem cell (HSC) compartment is expanded in 5A3+/del mice, and the distribution of myeloid progenitors is altered. 5A3+/del HSCs are defective for lymphoid repopulating potential and show a myeloid lineage output bias. These cell autonomous abnormalities are exacerbated by physiologic aging and upon serial transplantation. The 5A3 deletion partially rescues defective repopulation in Gata2 mutant mice. 5A3+/del hematopoietic cells exhibit decreased expression of oxidative phosphorylation genes, increased levels of reactive oxygen species, and perturbed oxygen consumption. These studies provide the first functional data linking 7q22 deletions to MDS pathogenesis.
https://doi.org/10.7554/eLife.07839.001eLife digest
Stem cells in the bone marrow are essential for creating new blood cells. Myelodysplastic syndrome (MDS) is a common type of blood cancer in the elderly that occurs when blood cells fail to develop normally. Depending on which types of blood cells are affected, individuals with MDS may bleed more easily, feel weak and tired, or be unable to effectively fight off infections.
Animals and plants store their genetic information in the form of chromosomes. Humans have 23 pairs of chromosomes, with one copy inherited from the mother, and the other from the father. The bone marrow cells of many people with MDS delete a section from one of their copies of chromosome 7. As this section contains many different genes, it is difficult to fully understand which specific genes contribute to the development of MDS when one copy is lost.
Wong et al. have now genetically engineered mice to lack a section of one of their copies of chromosome 7 that is often missing in patients with MDS. Bone marrow cells from these mice exhibit many of the same abnormalities found in human MDS. For example, most of the immature blood stem cells expand, but these stem cells do not correctly specialize into mature blood cells—in particular, not enough immune cells are produced. The developing blood cells also have problems expressing several genes, including one that helps to protect the cells from damaging molecules called reactive oxygen species. These problems worsen as the mice age.
These mice provide the first evidence directly linking the missing section of chromosome 7 to abnormalities found in MDS patients. Future studies using the mice will advance our understanding of how the loss of this section of chromosome 7 interacts with other genes involved in MDS to alter the course of this disease and how it responds to treatment.
https://doi.org/10.7554/eLife.07839.002Introduction
The myelodysplastic syndromes (MDSs) are clonal stem cell disorders characterized by ineffective hematopoiesis, morphologic dysplasia, and a variable risk of progression to acute myeloid leukemia (AML) (Elias et al., 2014). Monosomy 7 (−7) and deletions affecting the long arm of chromosome 7 [del(7q)] are highly prevalent acquired cytogenetic abnormalities in de novo and in therapy-related MDS and AML (t-MDS/t-AML) (Smith et al., 2003). The proportion of −7/del(7q) cells is markedly increased in the hematopoietic stem cell (HSC) and progenitor compartments of MDS patients relative to T and B lymphocytes (Kere et al., 1987b; Abrahamson et al., 1991; Bernell et al., 1996; Will et al., 2012; Elias et al., 2014). Recent studies demonstrating quantitative changes in the frequencies of phenotypic primitive long-term HSCs, common myeloid progenitors (CMPs), and granulocyte-monocyte progenitors (GMPs) in MDS patients with −7/del(7q) further support diverse effects on hematopoiesis (Will et al., 2012; Pang et al., 2013).
Multiple studies of MDS and AML specimens with interstitial deletions on 7q have implicated three putative commonly deleted segments (CDSs) at chromosome bands 7q22, 7q34, and 7q35-36 (Figure 1A) (Kere et al., 1987a; Le Beau et al., 1996; Fischer et al., 1997; Liang et al., 1998; Tosi et al., 1999; Jerez et al., 2012; McNerney et al., 2013; Hosono et al., 2014). Of these intervals, 7q22 is deleted most frequently in cases of MDS or AML with a del(7q) (Le Beau et al., 1996). Targeted sequencing of candidate myeloid tumor suppressor genes (TSGs) located within a 2.5 Mb 7q22 CDS delineated by Le Beau et al. (1996), and recent comprehensive genomic analyses of clinical specimens strongly implicate a haploinsufficient role of 7q22 deletions in leukemogenesis (Kere et al., 1989; Fischer et al., 1997; Liang et al., 1998; Tosi et al., 1999; Ebert, 2011; Jerez et al., 2012; McNerney et al., 2013; Hosono et al., 2014). Consistent with this proposed mechanism, biallelic inactivation of any 7q gene is rare in MDS patients with −7/del(7q). For example, whole exome sequencing of 68 myeloid malignancies characterized by −7/del(7q) uncovered recurrent mutations in only EZH2 (located at 7q36; n = 4) and LUC7L2 (at 7q34; n = 3). Biallelic inactivation of these genes and of CUX1 (located at 7q22) was observed in a small cohort of patients with 7q isodisomy (Hosono et al., 2014). SAMD9L, RASA4, DOCK4, and MLL3 are other 7q genes that have been implicated as contributing to leukemogenesis by haploinsufficiency or epigenetic transcriptional repression (Figure 1A) (Asou et al., 2009; Zhou et al., 2011; Nagamachi et al., 2013; Chen et al., 2014; Poetsch et al., 2014). Here, we demonstrate hematopoietic abnormalities in mice with a germ line deletion of a contiguous CDS of chromosome band 5A3 (5A3+/del) syntenic to a 2.5 Mb 7q22 CDS delineated by Le Beau et al. (1996) that support a mechanistic role of 7q22 deletions in MDS pathogenesis.
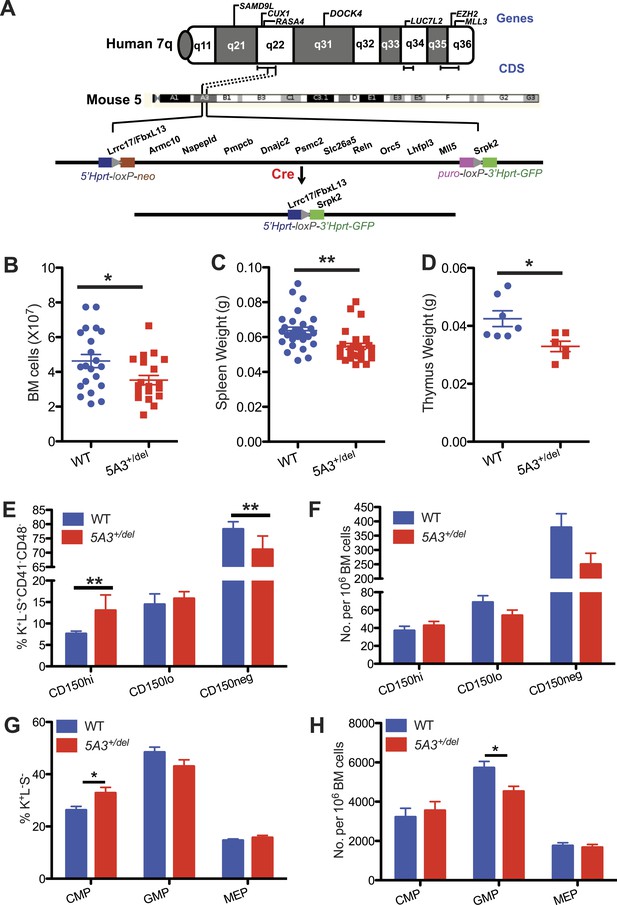
A heterozygous 5A3 deletion corresponding to human 7q22 perturbs steady-state hematopoiesis.
(A) Top, candidate 7q myeloid tumor suppressor genes described previously (Asou et al., 2009; Ernst et al., 2010; Nikoloski et al., 2010; Zhou et al., 2011; McNerney et al., 2013; Chen et al., 2014; Hosono et al., 2014; Poetsch et al., 2014) appear above the diagram of chromosome 7q while commonly deleted segments (CDSs) within 7q22, 7q34, and 7q35-36 identified by different research groups (Kere et al., 1987a; Le Beau et al., 1996; Fischer et al., 1997; Liang et al., 1998; Tosi et al., 1999; Jerez et al., 2012; McNerney et al., 2013; Hosono et al., 2014) are depicted by brackets immediately below it. Middle, dotted lines demarcate the segments of mouse chromosome 5A3 corresponding to the human 7q22 CDS targeted in this study. Bottom, expressing Cre recombinase in embryonic stem (ES) cells doubly targeted with LoxP sequences within the Fbxl13 and Srpk2 genes excised a 2-Mb interval. Gene order is based on the Ensembl database and is not drawn to scale. (B) Total numbers of bone marrow (BM) cells from 2 femurs and 2 tibiae in 5A3+/del mice and wild-type (WT) littermates at 8–12 weeks of age. (C, D) Spleen (C) and thymus (D) weights in 5A3+/del mice and WT littermates at 8–12 weeks of age. (E, F) Percent contributions (E) and frequencies (F) of cells with high (CD150hi HSC), low (CD150lo HSC), and absent CD150 expression (CD150neg MPP) within the K+L−S+CD41−CD48− compartment of WT and 5A3+/del mice at 8–12 weeks of age (n = 6 of each genotype). (G) Percent contribution of common myeloid progenitor (CMP), granulocyte-monocyte progenitor (GMP), and megakaryocyte erythroid progenitors (MEP) within the Lin−Sca1+c-kit+ compartment of 5A3+/del mice and WT littermates. (H) Frequencies of CMP, GMP, and MEP in WT and 5A3+/del BM. The error bars indicate S.E.M. with significant differences between WT and 5A3+/del mice designated by asterisks as follows: *p < 0.05, **p < 0.01.
Results and discussion
Abnormal differentiation and repopulation of 5A3+/del stem and progenitor cells
We generated mice carrying a 2 Mb germ line 5A3 deletion that removes 13 genes syntenic to a human 7q22 CDS (Figure 1A) (Wong et al., 2010). 5A3+/del mice are smaller than wild-type (WT) littermates, and homozygous deletion of the 5A3 region causes embryonic lethality before 10.5 dpc (data not shown). Total nucleated bone marrow (BM) cell counts as well as spleen and thymus weights are reduced in mutant animals (Figure 1B–D), which maintain normal peripheral blood cell counts.
Differential expression of CD150 distinguishes HSC populations with different self-renewal, differentiation, and repopulating potentials. Specifically, HSCs, with a surface c-kit+, lineage−, Sca-1+ (KLS), and CD150hi (CD150hi HSC) immunophenotype possess potent self-renewal capacity, are predisposed to myeloid differentiation and expand upon aging (Kiel et al., 2005; Beerman et al., 2010a; Hock, 2010). Strikingly, the proportion of CD150hi HSCs is increased in 5A3+/del BM with a corresponding decrease in the percentage of CD150 negative multi-potent progenitors (CD150neg MPP) (Figure 1E, Figure 1—figure supplement 1A). This results in a normal frequency of CD150hi HSCs in 5A3+/del mice, despite an overall reduction in the size of the stem/progenitor compartment (Figure 1F). The proportion of CMPs is elevated in 5A3+/del mice and the frequency of GMPs is decreased (Figure 1G,H, Figure 1—figure supplement 1B), which is consistent with changes in these populations in MDS patients (Will et al., 2012; Pang et al., 2013). Thus, the 5A3 deletion perturbs HSC and myeloid progenitor populations. By contrast, the proportions and frequencies of common lymphoid progenitors are similar in WT and 5A3+/del mice (Figure 1—figure supplement 1C–E).
We mixed donor 5A3+/del or WT BM cells with WT BM at ratios of 1:1 and 1:2 and transplanted them into irradiated recipients. Whereas 5A3 mutant BM had markedly reduced lymphoid repopulating capacity, these cells efficiently contributed to the c-kit+lin−Sca-1+ (KLS) compartment (Figure 2A,B). To investigate if the altered repopulating potential of 5A3+/del BM is intrinsic to CD150hi HSC, we injected 15 of these cells into lethally irradiated recipients with WT BM competitors. Similar to whole BM, CD150hi HSC from 5A3+/del mice exhibited reduced overall repopulation potential due to defective lymphoid reconstitution (Figure 2C–F). Importantly, however, 5A3+/del HSC efficiently reconstituted KLS and myeloid compartments in both primary and secondary recipients (Figure 2E,F). 5A3+/del CD150hi HSC exhibited markedly reduced lymphoid repopulating potential in WT and 5A3+/del recipients, whereas WT cells restored lymphoid repopulation in 5A3+/del hosts almost as well as in WT recipients, demonstrating that the repopulation defects in 5A3+/del CD150hi HSCs are cell intrinsic (Figure 2C–F).
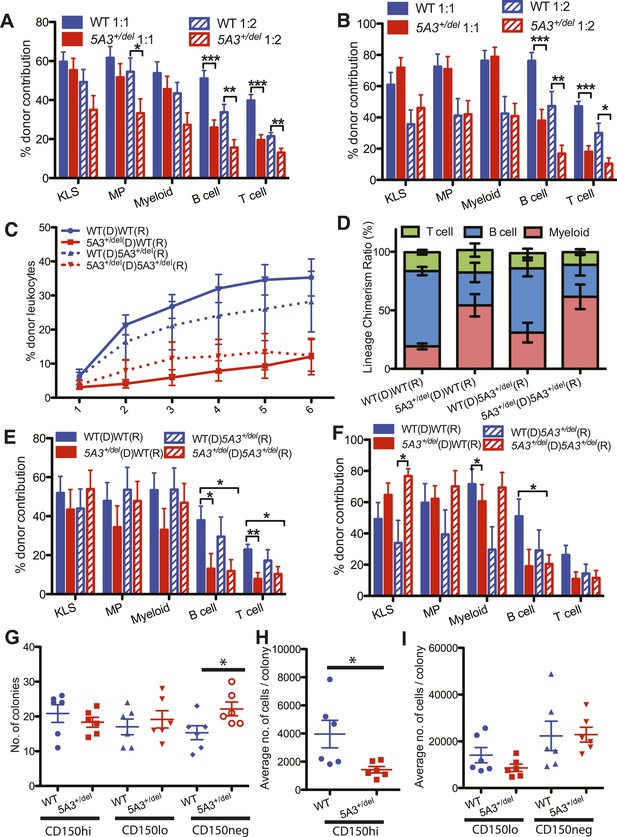
Defective repopulating potential of 5A3+/del BM and CD150hi HSC.
(A, B) BM cells from WT or 5A3+/del mice (n = 9 per genotype) were mixed at ratios of 1:1 and 1:2 with WT competitor cells and transplanted into 2–3 irradiated WT recipients. Percent contribution to the K+L−S+ (KLS), K+L−S− (MP), myeloid, B and T cell lineages in the BM of recipient mice 6 months after primary (A) and secondary (B) transplants are shown. (C) Leukocyte chimerism after competitive transplantation of 15 5A3+/del or WT CD150hi HSC into WT or mutant recipients (n = 12 for WT hematopoietic stem cell (HSC) in WT recipients; n = 12 for 5A3+/del HSC in WT recipients; n = 8 for WT HSC in 5A3+/del recipients; n = 9 for 5A3+/del HSC in 5A3+/del recipients). (D–F) Relative proportions of donor-derived B, T, and myeloid cells in the blood of recipient mice 6 months after transplantation (D). Percent contribution to the K+L−S+ (KLS), K+L−S− (MP), myeloid, B and T cell lineages in the BM of recipient mice 6 months after primary (E) and secondary (F) transplants. Data shown are mean values ±SEM of results from four independent experiments. (G–I) 100 CD150hi HSC, CD150lo HSC, and CD150neg MPP from 8- to 12-week-old 5A3+/del mice and their WT littermates were plated into methylcellulose medium supplemented with cytokines (n = 6 for each genotype). The total number of colonies (G) and the average number of cells per colony (H, I) were assessed after 7 days. Dots represent individual mice, and the horizontal lines indicate median values. Data shown are mean values ±SEM of results from three independent experiments with significant differences between WT and 5A3+/del mice designated by asterisks as follows: *p < 0.05, **p < 0.01, ***p < 0.001.
WT and 5A3+/del HSCs generated similar numbers of myeloid colonies in methylcellulose cultures supplemented with cytokines; however, colonies grown from 5A3+/del CD150hi HSC contained significantly fewer cells (Figure 2G,H). By contrast, 5A3+/del CD150neg MPP showed a 1.4-fold increase in colony forming activity but a similar number of cells per colony as WT MPP (Figure 2G,I). In vivo labeling experiments revealed similar rates of BrdU incorporation, cell division, and apoptosis in WT and 5A3+/del K+L−S+ and myeloid progenitor (MP) cells (Figure 2—figure supplement 1A,B and data not shown) (Nygren and Bryder, 2008).
Effects of aging on 5A3+/del HSC and interaction with Gata2 haploinsufficiency
Physiologic aging is characterized by impaired HSC repopulating potential, diminished lymphoid differentiation, the dominance of CD150hi HSCs that are skewed toward myeloid differentiation and a markedly increased risk of MDS (Beerman et al., 2010a; Beerman et al., 2010b). Similarly, the abnormal distribution of HSCs is exacerbated in 24- to 30-month-old 5A3+/del mice (Figure 3A,B). Consistent with data from younger mice (Figure 2A–F), aged 5A3 mutant BM displayed markedly reduced lymphoid repopulating potential, but efficiently contributed to myeloid reconstitution (Figure 3C,D). Old 5A3+/del BM cells also repopulated the KLS compartment significantly better than WT BM in recipients analyzed 4 months after transplantation, and 5A3+/del cells exhibited a twofold increase in contribution to the KLS and MP populations upon serial transplantation (Figure 3C,D). Despite these HSC abnormalities, 5A3+/del mice have a normal lifespan, and the underlying causes of death are similar to WT littermates (data not shown).
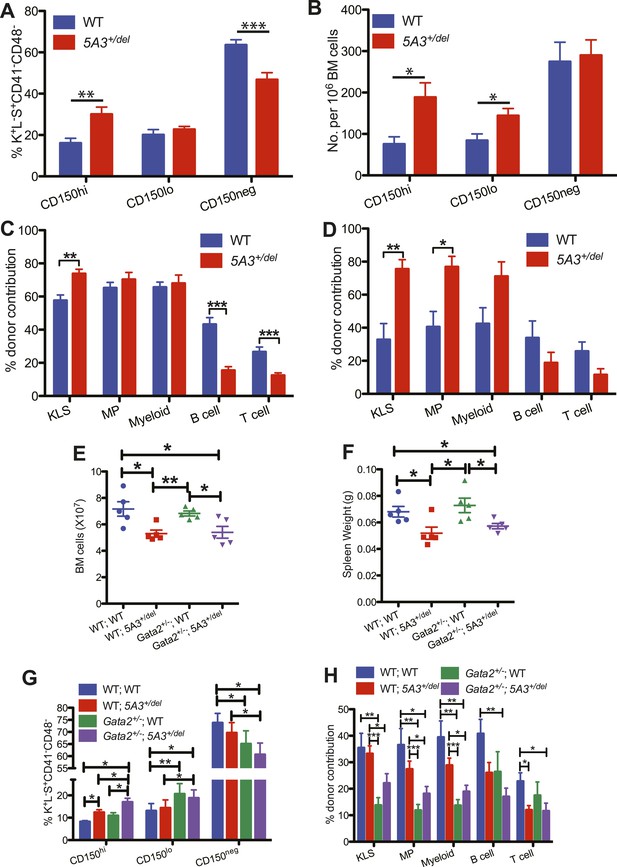
Effects of aging and Gata2 inactivation on 5A3+/del hematopoietic cells.
(A and B) Percent contributions (A) and frequencies (B) of CD150hi HSC, CD150lo HSC, and CD150neg MPP within the K+L−S+CD41−CD48− compartment in 5A3+/del mice (n = 12) and their WT littermates (n = 11) at 24–30 months of age. (C and D) Competitive transplantation of BM cells from 24- to 30-month-old WT or 5A3+/del mice. Percent donor contribution of 5A3+/del cells to K+L−S+ (KLS), K+L−S− (MP), myeloid, B cell, and T cell populations in the BM of recipient mice 4 months after primary (C) and secondary (D) competitive transplantation (n = 5 donors and 10 recipients of each genotype). Data shown are mean values ±SEM of results from two independent experiments. (E) Total numbers of BM cells from 2 femurs and 2 tibiae in 5A3+/del mice, Gata2+/− mice, compound Gata2+/−; 5A3+/del mice and WT littermates at 8–12 weeks of age. (F) Spleen weights in 5A3+/del mice, Gata2+/− mice, compound Gata2+/−; 5A3+/del mice, and WT littermates at 8–12 weeks of age. (G) Percent contributions of cells with high (CD150hi HSC), low (CD150lo HSC), and absent CD150 expression (CD150neg MPP) within the K+L−S+CD41−CD48− compartment of WT, 5A3+/del, Gata2+/−, and compound Gata2+/−; 5A3+/del mice at 8–12 weeks of age (n = 5 of each genotype). (H) BM cells from WT, 5A3+/del, Gata2+/− or compound Gata2+/−; 5A3+/del mice (n = 5 of each genotype) were each mixed at ratios of 1:1 with WT competitor cells and transplanted into two irradiated WT recipients. Percent contribution to the K+L−S+ (KLS), K+L−S− (MP), myeloid, B and T cell lineages in the BM of recipient mice 6 months after primary transplants. Data shown are mean values ±SEM from five independent experiments with significant differences designed by asterisks as follows: *p < 0.05, **p < 0.01, ***p < 0.001. The enhanced repopulating ability of compound Gata2+/−; 5A3+/del vs Gata2 singly mutant HSC achieved borderline statistical significance in three myeloid populations (KLS (p = 0.09), MP (p = 0.09), and myeloid cells (p = 0.12)).
GATA2 mutations cause familial MDS, which is frequently characterized by −7/del(7q) (Hahn et al., 2011; Bodor et al., 2012; Pasquet et al., 2013). We generated Gata2+/−; 5A3+/del mice, quantified HSC and progenitor populations, and performed competitive repopulation experiments. Like 5A3+/del mutant mice, Gata2+/−; 5A3+/del mice have reduced BM cellularity and spleen weights (Figure 3E,F), and the CD150hi HSC bias is augmented by concomitant Gata2 deletion (Figure 3G). Whereas lymphoid reconstitution is similarly impaired in 5A3+/del and compound mutant mice, the 5A3 deletion partially rescues the repopulating deficit of Gata2+/− BM in the KLS, MP, and myeloid compartments (Figure 3H) (Rodrigues et al., 2005). These data suggest that the 7q22 CDS contributes to transformation in familial MDS by impairing lymphoid differentiation while also modestly enhancing the growth of GATA2 mutant HSC and their myeloid progeny.
Changes in gene expression and metabolic activities in 5A3+/del hematopoietic cells
Transcriptome (RNA-Seq) and TaqMan quantitative real-time PCR analyses revealed a ∼50% reduction in the expression of multiple genes and of the long intergenic non-coding RNA 503142E22Rik within the 5A3 interval in mutant HSC and MPP (Figure 4A,B). Gene Set Enrichment Analysis (GSEA) of the RNA-Seq data from 5A3+/del HSCs further demonstrated reduced expression of multiple gene sets related to oxidative phosphorylation (OXPHOS) that are similarly down-regulated in the early stages of human therapy-induced MDS and AML (Figure 4C) (Mootha et al., 2003; Li et al., 2011). OXPHOS is the metabolic pathway used by cells to generate adenosine triphosphate (ATP). OXPHOS is regulated by mitochondrial membrane potential, and defects in this metabolic pathway can increase levels of reactive oxygen species (ROS). However, sorted WT and 5A3+/del HSC and MPP showed similar ATP levels (Figure 4D). Membrane potential and intracellular ROS levels were also similar in HSC and MPP from young mice, but ROS levels were increased by ∼50% in the HSC and MPP of aged 5A3+/del mice (Figure 4E,F). Elevated ROS levels in HSCs correlate with reduced self-renewal capacity, impaired multi-lineage repopulating ability, and myeloid-biased differentiation (Jang and Sharkis, 2007). ROS levels are also increased in t-MDS/AML BM (Reinecke et al., 2009; Li et al., 2011).
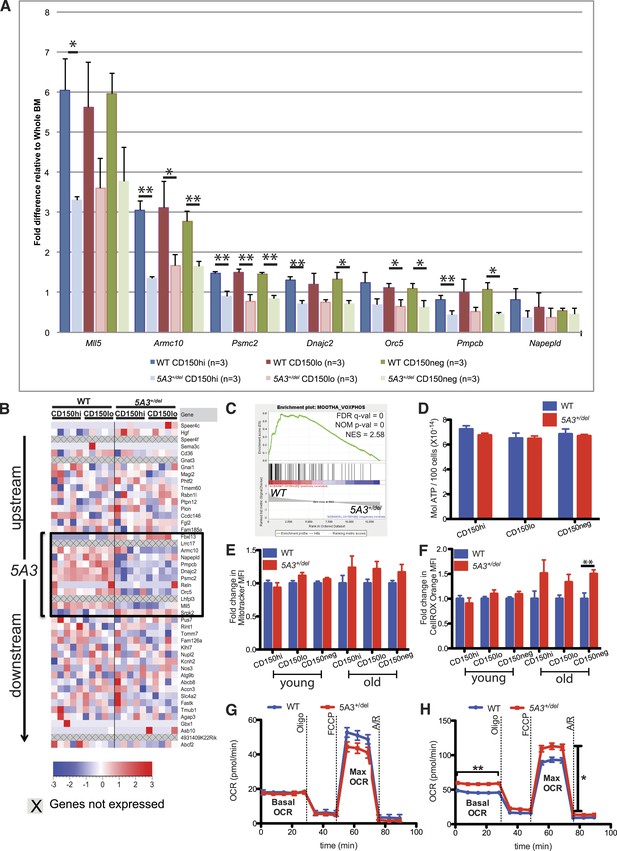
Changes in gene expression and oxidative phosphorylation in 5A3+/del HSC and MP.
(A) Relative mRNA abundances for genes within the deleted 5A3 interval expressed at detectable levels in sorted HSC populations were determined by TaqMan reverse transcriptase PCR (n = 3 per genotype). (B) Expression levels of genes located within and flanking the deleted interval measured by RNA-Seq in sorted CD150hi HSC and CD150lo HSC from 5 mice of each genotype. Each column presents data from an individual mouse, and genes within the 5A3 deleted region are delimited with a black box. Three non-coding RNAs (6030443J06Rik, AC112688.1 and 5031425E22Rik) are located within the 5A3 deletion. Two of these (6030443J06Rik and AC112688.1) are expressed at extremely low levels in HSC, and the other (5031425E22Rik) showed ∼50% lower expression in 5A3+/del HSC. 5031425E22Rik is homologous to the human KMT2E (a.k.a. MLL5) antisense RNA1. Expression levels of the flanking Fbxl13 and Srpk2 genes are modestly up-regulated in 5A3+/del HSC, which is consistent with the targeting strategy used to generate the segmental deletion. (C) Gene Set Enrichment Analysis of 5A3+/del CD150hi HSCs revealed negative enrichment for genes associated with oxidative phosphorylation (OXPHOS). False discovery rate (FDR) q-val, nominal p-value (NOM p-value), and normalized enrichment scores (NESs) are indicated. (D) ATP levels in HSC and MPP from 8- to 12-week-old WT (n = 6) and 5A3+/del (n = 5) mice. Data shown are mean values ±SEM of results from two independent experiments. (E) Fold change in the mean MitoTracker Orange fluorescence levels in 5A3+/del cells normalized to values in WT cells analyzed in the same experiment. (F) Fold change in the mean fluorescence level (MFI) of 5A3+/del cells that are CellROX Orange positive normalized to values in WT cells analyzed in the same experiment. For the MitoTracker and CellROX experiments, n = 13 for WT and n = 12 for 5A3+/del young mice, three independent experiments; n = 5 for WT and n = 6 for 5A3+/del aged mice, two independent experiments. Data shown are mean values ±SEM of results from independent experiments. (G and H) Oxygen consumption rate (OCR) was assessed basally and in response to the mitochondrial inhibitors oligomycin (oligo), carbonyl cyanide 4-(trifluoromethoxy) phenylhydrazone (FCCP), and antimycin A and rotenone (A/R) for (G) KLS and (H) MP cells. Data are shown as mean ±SEM of n = 5 mice of each genotype from two independent experiments.
Down-regulation of OXPHOS genes is expected to reduce mitochondrial respiration in HSC (Warr and Passegue, 2013). We attempted to directly measure oxygen consumption rates (OCRs) in CD150hi and CD150lo HSC but could not obtain reproducible results due to limiting cell numbers. We therefore compared the OCRs of KLS, MP, B, and T cells isolated from 1-year-old WT and 5A3+/del mice. 5A3+/del KLS cells showed a similar basal OCR as their WT counterparts, but a slightly lower maximal respiratory capacity that did not reach statistical significance (p = 0.2562) (Figure 4G). 5A3+/del MPs surprisingly showed a significantly higher basal respiration and maximal respiratory capacity in comparison to WT cells, supporting an overall increase in energy consumption (Figure 4H). Meanwhile, mature 5A3+/del B and T cells have similar mitochondrial stress profiles as WT cells. We conclude that global changes in OXPHOS gene expression exert a modest impact on cellular metabolism in aged 5A3 mutant HSC and progenitors. Interestingly, treatment with N-acetyl-L-cysteine (NAC) did not reverse the hematopoietic abnormalities in young 5A3+/del mice (data not shown), suggesting that they are a direct consequence of the 5A3 deletion and are not secondary to ROS production.
Segmental deletions are among the most frequent genetic alterations in human cancer, and simultaneous loss of multiple haploinsufficient TSGs that individually have minimal phenotypic consequences appears to underlie the growth advantage conferred by most of these chromosomal losses (Solimini et al., 2012). We show that a haploinsufficient deletion in mice that models loss of a human 7q22 CDS causes hematopoietic abnormalities that include a myeloid lineage output bias, impaired lymphoid repopulating potential, and a pronounced age-associated expansion of HSC and MPs. These functional abnormalities support a role of 7q22 deletions in MDS pathogenesis. The seven genes within the deleted 5A3 segment that are expressed in HSC encode proteins that regulate diverse cellular processes including transcription (Mll5 and Dnajc2), mitochondrial quality control (Pmpcb, Armc10), protein degradation (Psmc2), biosynthesis of N-acylethanolamines (Napepld), and DNA replication (Orc5) (Luciano et al., 1997; Quintana et al., 1998; Fujiki et al., 2009; Sebastian et al., 2009; Richly et al., 2010; Nijhawan et al., 2012; Tsuboi et al., 2013; Zhou et al., 2013; Serrat et al., 2014). Given this, it is likely that the haploinsufficiency for multiple interacting genes leads to altered hematopoietic differentiation and function in 5A3+/del mice. Similar to the 5A3+/del mice described here, other mutations found in MDS patients perturb hematopoiesis, but do not consistently induce hematologic disease in the absence of cooperating mutations (Beurlet et al., 2013). This is not unexpected given the advanced age of most MDS patients and the presence of multiple genetic lesions in diseased BM. The 5A3+/del mice reported here thus provide a novel resource for addressing how this common deletion cooperates with other mutations to drive myeloid transformation, progression to AML, and drug resistance.
Materials and methods
Mice
We expressed Cre recombinase in embryonic stem cells containing LoxP sites and hypoxanthine phosphoribosyl transferase (HPRT) sequences flanking the Fbxl13 and Srpk2 genes (Wong et al., 2010) and analyzed clones that grew in hypoxanthine-aminopterin-thymidine (HAT) medium to identify the desired 2 Mb deletion. 5A3del/+ mice were generated by standard blastocyst injection followed by mating coat color chimeras and were backcrossed for at least 10 generations onto a C57BL/6J background. Gata2+/− mice were a generous gift from Dr Stuart Orkin (Harvard Medical School) (Tsai et al., 1994). Study mice were housed in a specific pathogen-free facility at the University of California San Francisco, and all animal experiments were conducted under protocols approved by the Institutional Animal Care and Use Committee. Genotyping and disease monitoring were performed as previously described (Wong et al., 2010).
Flow cytometry
Request a detailed protocolBM cells flushed from tibias and femurs were subjected to ammonium-chloride potassium red cell lysis before staining with antibodies. For experiments requiring cell sorting, the spinal cord, flat bone of the pelvis, humerus, and sternum of the mice were also crushed and lysed. Low-density mononuclear cells were separated using a HISTOPAQUE-1119 gradient (Sigma–Aldrich, St. Louis, MO, United States). For identification and sorting of CD150hi-HSC, CD150lo-HSC, and CD150neg-MPP, cells were pre-incubated with purified CD16/32 (2.4G2), followed by staining with a lineage cocktail of PE-conjugated antibodies including B220 (RA3-6B2), CD8 (53-6.7), Gr-1 (RB6-8C5), CD3 (17A2), Ter119 (TER-119), CD41 (MWReg30), and CD48 (HM48-1), as well as PE-Cy7 c-kit (2B8), PacBlue Sca-1 (E13-161.7), and APC CD150 (TC15-12F12.2) from BioLegend (San Diego, CA, United States). For experiments requiring cell sorting, cells expressing c-kit were enriched by magnetic cell sorting by staining with mouse CD117 microbeads and positively selected on the MS columns (Miltenyi Biotec, Germany) according to manufacturer's protocol before antibody staining. Cells were classified as CD150hi-HSC, CD150lo-HSC, or CD150neg-MPP based in levels of CD150 expression.
Percent contribution of common myeloid progenitor (CMP), granulocyte-monocyte progenitor (GMP), and megakaryocyte erythroid progenitors (MEP) within the Lin−Sca1+c-kit+ compartment of 5A3+/del mice and WT littermates. CMP, GMP, and megakaryocyte erythroid progenitors (MEP) populations were identified by flow cytometry after staining with a lineage cocktail of PE-conjugated antibodies including B220 (RA3-6B2), CD8 (53-6.7), Gr-1 (RB6-8C5), CD3 (17A2), and Ter119 (TER-119), as well as APC-750 c-kit (2B8), PacBlue Sca-1 (E13-161.7), Alexa Fluor 647 CD16/32 (93), biotin CD34 (MEC14.7), and a streptavidin PE-Cy7 conjugate (BioLegend). Stained BM cells were analyzed in a FACS LSRII instrument and sorted in a FACS Aria3 (Becton Dickinson, San Jose, CA, United States). FlowJo software (Tree Star, Inc., Ashland, OR, United States) was used to analyze and display the data.
Competitive repopulation
Request a detailed protocolBM cells from WT, 5A3+/del, Gata2+/−, Gata2+/−5A3+/del mice on a C57BL/6J background (CD45.2) were used as donor cells. Competitor cells were isolated from 8- to 12-week-old BoyJ mice (CD45.1). Recipient F1 hybrid mice from a cross between C57BL/6J and BoyJ mice (CD45.1 + CD45.2) were at least 8 weeks old at the time of lethal irradiation (9.5 Gy from a cesium source delivered in split dose 3 hr apart). After irradiation, the cells were injected via the tail vein of recipient mice. For evaluation of the competitiveness of whole BM, we injected 106 whole BM cells at a 1:1 or 1:2 donor to competitor ratio. To evaluate the repopulating potential of purified CD150hi-HSC, we injected 15 CD150hi-HSC sorted from 8- to 12-week-old WT and 5A3+/del mice together with 2.5 × 105 BM competitor cells into lethally irradiated recipients.
Blood was obtained from recipient mice every 30 days beginning 1 month after transplant, and cells were stained with Alexa Fluor 700 CD45.2 (104), PE-Cy7 CD45.1 (A20), PacBlue B220 (RA3-6B2), FITC CD4 (GK1.5), FITC CD8 (53-6.7), PE Mac-1 (M1/70), and PE Gr-1 (RB6-8C5) to determine the percent donor cell contribution to myeloid and B and T lymphoid lineages. Primary recipient mice were euthanized 6 months after transplantation, BM were isolated from the tibiae and femur, and 2 × 106 BM cells were injected into secondary recipients to test serial repopulation potential. To determine the contribution of donor-derived cells in the K+L−S+ compartment, BM cells were stained with V450 CD45.2 (104), APC-780 CD45.1 (A20), PE B220 (RA3-6B2), PE CD8 (53-6.7), PE Gr-1 (RB6-8C5), PE CD3 (17A2), PE Ter119 (TER-119), PE-Cy7 c-kit (2B8), and APC Sca-1 (E13-161.7). Secondary recipients were analyzed as in the primary recipients.
Methylcellulose colony assays
Request a detailed protocolCD150hi-HSC, CD150lo-HSC, and CD150neg-MPP were isolated as described above, and 100 cells were seeded into methylcellulose medium as described (Mohrin et al., 2010). Colonies were counted on day 7, and the entire contents of a methylcellulose culture from an individual plate were then flushed out using phosphate-buffered saline and counted in a hemocytometer. Cells were also spun in a Cytospin 3 Cytocentrifuge (Shandon/Thermo Fisher Scientific, Waltham, MA, United States) at 400 rpm for 8 min, and differential cell counting and morphological analysis performed after Wright-Giemsa staining.
RNA isolation and expression
Request a detailed protocolCD150hi-HSC, CD150lo-HSC, and CD150neg-MPP were sorted as above into 500 µl of TRIzol reagent (Life Technologies, Grand Island, NY, United States). RNA was isolated according to manufacturer's instructions and precipitated with the addition of glycogen (New England BioLabs, Ipswich, MA, United States). The RNA was then treated with DNAse 1 (Ambion, Austin, TX, United States) and purified with the RNeasy MinElute Cleanup Kit (Qiagen, Valencia, CA, United States).
For TaqMan analysis, reverse transcription was carried out using the High Capacity RNA-to-cDNA Master Mix (Life Technologies). Relative quantification of gene expression was determined by performing quantitative real-time PCR using the following TaqMan Gene Expression Assays (Applied Biosystems): Armc10 (Mm03011576_g1), Mll5 (Mm01129502_g1), Napepld (Mm00724596_m1), Orc5 (Mm00457242_m1), Psmc2 (Mm00803207_m1), Dnajc2 (Mm00494389_m1), Pmpcb (Mm01138654_m1), Srpk2 (Mm00486413_m1), Fbxl13 (Mm00622025_m1), Lrrc17 (Mm01167263_m1), Slc26a5 (Mm00446145_m1), Reln (Mm00465200_m1), Lhfpl3 (Mm03038441_m1), and Gapdh (Mm99999915_g1) with the TaqMan Gene Expression Master Mix (ABI). PCR reactions were performed on an ABI 7900HT Real-Time PCR System (Applied Biosystems, Foster City, CA, United States) with the Taqman Gene Expression Master Mix (Applied Biosystems) according to manufacturer's instructions. PCR cycling conditions were 2 min at 50°C and 10 min at 95°C, followed by 40 cycles of 15 s at 95°C and 1 min at 60°C. All reactions were carried out in triplicate, and target quantities were determined using a relative standard curve. The amounts of target were normalized to the endogenous control gene Gapdh and compared with the corresponding WT BM (calibrator sample) to determine relative fold differences.
For RNA-Seq analysis, total RNA (10 ng) was converted into double-stranded cDNA using the Ovation RNA Amplification System V2 (NuGen, San Carlo, CA, United States) per manufacturer's recommendations. The amplified cDNA products were then used to generate RNA-seq libraries using the TruSeq RNA Sample Preparation Kit v2 reagents (Illumina, San Diego, CA, United States) per manufacturer's instructions, with 10 PCR amplification cycles. Library quality and quantity were assessed by the Agilent DNA1000 Chip (Agilent, Santa Clara, CA, United States) and qPCR (Kappa Biosystems Inc, Woburn, MA, United States). 10 pM of each library was sequenced using Illumina SBS chemistry at 2 × 100 bp reads on the HiSeq2000 (Illumina, San Diego, CA, United States).
The RNA-Seq paired-end reads were mapped to the mouse mm9 genome using an in-house mapping and quality assessment pipeline (Zhang et al., 2012). The expression of each gene was estimated by the mean coverage of the highest covered coding exon. Genes with low-expression level (<10) across all samples were filtered out, followed by quantile normalization. Differential expression analysis was performed using limma (Smyth, 2004) with estimation of false discovery rate (Benjamini and Hochberg, 1995). GSEA (Subramanian et al., 2005, 2007) was used to assess pathway enrichment.
ATP quantification
Request a detailed protocolHSC and MPP were sorted into phosphate buffered saline (PBS), and 600 CD150hi-HSC, 1000 CD150lo-HSC, and 1000 CD150neg-MPP were aliquoted into a well of a 96-well plate in triplicate. ATP was quantified using the CellTiter-Glo Luminescent Cell Viability Assay (Promega, Madison, WI, United States) following manufacturer's recommendations. Illumination was quantified with a Synergy 2 (Biotek, Winooski, VT, United States).
Metabolic studies
Request a detailed protocolFor flow cytometric analysis of ROS levels and membrane potential in CD150hi-HSC, CD150lo-HSC, and CD150neg-MPP, BM cells were isolated and enriched for c-kit positive cells as described. c-kit positive cells were stained with an unconjugated cocktail of purified antibodies including B220 (RA3-6B2), CD8 (53-6.7), Gr-1 (RB6-8C5), CD3 (17A2), Ter119 (TER-119), and CD41 (MWReg30) from BioLegend, followed by PECy-5 IgG GOAT anti-RAT pAb (HI47) antibody from Molecular Probes and IgG from rat serum (I4131 Sigma). After incubation with 50 nM CellROX Orange Reagent (Molecular Probes) or 20 nM MitoTracker Orange CMTMRos (Molecular Probes, Eugene, OR, United States) for 30 min at 37°C in IMDM, cells were washed and stained with 7-amino-actinomycin D, PE-Cy7 c-kit (2B8), PacBlue Sca-1 (E13-161.7), PE-Cy5 CD48 (HM48-1), and APC CD150 (TC15-12F12.2) from BioLegend.
The OCR was analyzed in an XF96 extracellular flux analyzer following manufacturer's protocol (Seahorse Biosciences, Billerica, MA, United States). Freshly isolated K+L−S+ cells and K+L−S− cells were cultured in StemSpan serum-free medium (STEMCELL Technologies, Vancouver, Canada) supplemented with SCF (100 ng/ml) and Tpo (100 ng/ml), while freshly isolated thymic cells and B-220+ splenic cells were cultured in RPMI medium 1640 supplemented with 10% fetal calf serum and incubated at 37° in a humidified atmosphere containing 8% CO2 for 12–15 hr. Cells were then washed three times with Mito Stress Media (XF base media supplemented with glucose [3 mg/ml], sodium pyruvate [1 mM], and Glutamax [2 mM] adjusted to a pH = 7.4) and seeded in XF96 microplates coated with poly-L-lysine (Sigma). 60000 K+L−S+ cells, 100000 K+L−S− cells, 200000 thymic cells, and 200000 B220+ splenic cells were plated per well, respectively. K+L−S+ and K+L−S− cells were stimulated with SCF (100 ng/ml) and Tpo (100 ng/ml), while thymic cells were stimulated with interleukin (IL)-2 (20 ng/ml) and IL-7 (10 ng/ml) and B-220+ splenic cells were stimulated with IL-7 (10 ng/ml) and maintained in a non-CO2 incubator for 1 hr before the assay. Five baseline recordings were made, followed by sequential injection of Oligomycin (Sigma), Carbonyl Cyanide 4-(trifluoromethoxy)phenylhydrazone (Sigma), and a combination of Antimycin A (Sigma) and Rotenone (Sigma) to determine the mitochondrial respiration rate under various conditions.
Statistical analysis
Request a detailed protocolData are presented as mean values ±SEM unless stated otherwise. Statistical significance was determined by performing two-tailed Student's t-tests.
Antibodies for flow cytometry of CLP cells
Request a detailed protocolCLP were identified and evaluated by flow cytometry by staining with a lineage cocktail of PE-conjugated antibodies including B220 (RA3-6B2), CD8 (53-6.7), Gr-1 (RB6-8C5), CD3 (17A2), and Ter119 (TER-119), as well as APC-750 c-kit (2B8), PacBlue Sca-1 (E13-161.7), APC IL-7Rα (A7R34) and biotin Flk2 (A2F10), and a streptavidin PE-Cy7 conjugate (BioLegend).
BrdU staining
Request a detailed protocolMice received an initial intraperitoneal injection of BrdU (Sigma–Aldrich, 1 mg/6 g mouse weight) and were then maintained on 1.0 mg/ml BrdU in the drinking water 24 hr prior to sacrifice. To measure BrdU incorporation, BM cells were enriched for c-kit positive cells using c-kit antibody-conjugated microbeads (Miltenyi). Enriched BM cells were pre-incubated with purified CD16/32 (2.4G2), followed by staining with a lineage cocktail of PE-conjugated antibodies including B220 (RA3-6B2), CD8 (53-6.7), Gr-1 (RB6-8C5), CD3 (17A2), Ter119 (TER-119), as well as PECy7 c-kit (2B8) and PacBlue Sca-1 (E13-161.7) (BioLegend). BrdU incorporation was then assayed according to manufacturer's instructions of the APC BrdU Flow Kit (BD Pharmingen, San Jose, CA, United States).
NHS-biotin staining
Request a detailed protocolNHS-biotin (EZ-Link Sulfo-NHS-LC-LC-biotin; Life Technologies) was dissolved at 10 mg/ml in normal saline and injected into mice intravenously via the tail vein at 1 mg/6 g mouse weight. To assess biotin dilution in the HSC subpopulations, BM cells were enriched for c-kit positive cells using c-kit antibody-conjugated microbeads (Miltenyi). Enriched cells were pre-incubated with purified CD16/32 (2.4G2), followed by staining with a lineage cocktail of PE-conjugated antibodies including B220 (RA3-6B2), CD8 (53-6.7), Gr-1 (RB6-8C5), CD3 (17A2), Ter119 (TER-119), CD41 (MWReg30), and CD48 (HM48-1), as well as PerCP/Cy5.5 c-kit (2B8), PacBlue Sca-1 (E13-161.7), APC CD150 (TC15-12F12.2), and PECy-7-conjugated Streptavidin (BioLegend).
Data availability
-
Data from: Functional evidence implicating chromosome 7q22 haploinsufficiency in myelodysplastic syndrome pathogenesisAvailable at Gene Expression Omnibus (GEO) with the accession number GSE72811.
References
-
Identification of a common microdeletion cluster in 7q21.3 subband among patients with myeloid leukemia and myelodysplastic syndromeBiochemical and Biophysical Research Communications 383:245–251.https://doi.org/10.1016/j.bbrc.2009.04.004
-
Functionally distinct hematopoietic stem cells modulate hematopoietic lineage potential during aging by a mechanism of clonal expansionProceedings of the National Academy of Sciences of USA 107:5465–5470.https://doi.org/10.1073/pnas.1000834107
-
Stem cells and the aging hematopoietic systemCurrent Opinion in Immunology 22:500–506.https://doi.org/10.1016/j.coi.2010.06.007
-
Controlling the false discovery rate: a practical andpowerful approach to multiplet testingJournal of the Royal Statistical Society Series B (Methodological) 57:289–300.
-
Clonal cell lineage involvement in myelodysplastic syndromes studied by fluorescence in situ hybridization and morphologyLeukemia 10:662–668.
-
Molecular dissection of the 5q deletion in myelodysplastic syndromeSeminars in Oncology 38:621–626.https://doi.org/10.1053/j.seminoncol.2011.04.010
-
Stem cell origin of myelodysplastic syndromesOncogene 33:5139–5150.https://doi.org/10.1038/onc.2013.520
-
Molecular cytogenetic delineation of deletions and translocations involving chromosome band 7q22 in myeloid leukemiasBlood 89:2036–2041.
-
Some hematopoietic stem cells are more equal than othersThe Journal of Experimental Medicine 207:1127–1130.https://doi.org/10.1084/jem.20100950
-
Chromosome 7 long arm deletion in myeloid disorders: a narrow breakpoint region in 7q22 defined by molecular mappingBlood 73:230–234.
-
Molecular characterization of chromosome 7 long arm deletions in myeloid disordersBlood 70:1349–1353.
-
Monosomy 7 in granulocytes and monocytes in myelodysplastic syndromeThe New England Journal of Medicine 316:499–504.https://doi.org/10.1056/NEJM198702263160902
-
Cytogenetic and molecular delineation of a region of chromosome 7 commonly deleted in malignant myeloid diseasesBlood 88:1930–1935.
-
Molecular anatomy of chromosome 7q deletions in myeloid neoplasms: evidence for multiple critical lociProceedings of the National Academy of Sciences of USA 95:3781–3785.https://doi.org/10.1073/pnas.95.7.3781
-
Functional cooperation of the mitochondrial processing peptidase subunitsJournal of Molecular Biology 272:213–225.https://doi.org/10.1006/jmbi.1997.1231
-
Hematopoietic stem cell and progenitor cell mechanisms in myelodysplastic syndromesProceedings of the National Academy of Sciences of USA 110:3011–3016.https://doi.org/10.1073/pnas.1222861110
-
ORC5L, a new member of the human origin recognition complex, is deleted in uterine leiomyomas and malignant myeloid diseasesThe Journal of Biological Chemistry 273:27137–27145.https://doi.org/10.1074/jbc.273.42.27137
-
OXPHOS gene expression and control in mitochondrial disordersBiochimica et Biophysica Acta 1792:1113–1121.https://doi.org/10.1016/j.bbadis.2009.04.003
-
MLL5, a trithorax homolog, indirectly regulates H3K4 methylation, represses cyclin A2 expression, and promotes myogenic differentiationProceedings of the National Academy of Sciences of USA 106:4719–4724.https://doi.org/10.1073/pnas.0807136106
-
Clinical-cytogenetic associations in 306 patients with therapy-related myelodysplasia and myeloid leukemia: the University of Chicago seriesBlood 102:43–52.
-
Linear models and empirical bayes methods for assessing differential expression in microarray experimentsStatistical Applications in Genetics and Molecular Biology 3:Article3.https://doi.org/10.2202/1544-6115.1027
-
GSEA-P: a desktop application for gene set enrichment analysisBioinformatics 23:3251–3253.https://doi.org/10.1093/bioinformatics/btm369
-
Gene set enrichment analysis: a knowledge-based approach for interpreting genome-wide expression profilesProceedings of the National Academy of Sciences of USA 102:15545–15550.https://doi.org/10.1073/pnas.0506580102
-
Delineation of multiple deleted regions in 7q in myeloid disordersGenes, Chromosomes & Cancer 25:384–392.https://doi.org/10.1002/(SICI)1098-2264(199908)25:4<384::AID-GCC11>3.0.CO;2-D
-
Biosynthetic pathways of bioactive N-acylethanolamines in brainCNS & Neurological Disorders Drug Targets 12:7–16.https://doi.org/10.2174/1871527311312010005
-
Aberrant epigenetic and genetic marks are seen in myelodysplastic leukocytes and reveal Dock4 as a candidate pathogenic gene on chromosome 7qThe Journal of Biological Chemistry 286:25211–25223.https://doi.org/10.1074/jbc.M111.235028
-
Mixed lineage leukemia 5 (MLL5) protein regulates cell cycle progression and E2F1-responsive gene expression via association with host cell factor-1 (HCF-1)The Journal of Biological Chemistry 288:17532–17543.https://doi.org/10.1074/jbc.M112.439729
Article and author information
Author details
Funding
National Cancer Institute (NCI) (T32 CA108462, PO1 CA40046, R37 CA72614)
- Michael R Burgess
- James R Downing
- Kevin Shannon
St. Baldrick's Foundation (Kenneth and Mary Ellen Wilson St. Baldrick's Research Grant 123584A)
- Kevin Shannon
Leukemia and Lymphoma Society (LLS) (Specialized Center of Research Award LLS 7019-04)
- James R Downing
- Kevin Shannon
St. Jude Children's Research Hospital (American Lebanese Syrian Associated Charities)
- James R Downing
National Heart, Lung, and Blood Institute (NHBLI) (RO1 HL092471)
- Emmanuelle Passegué
American Cancer Society (Research Professorship)
- Kevin Shannon
American Cancer Society (Hillcrest Fellowship)
- Michael R Burgess
The funders had no role in study design, data collection and interpretation, or the decision to submit the work for publication.
Acknowledgements
We are grateful to Michelle Le Beau, Qing Li, Scott Lowe, and Megan McNerney for insightful suggestions and to Stuart Orkin for providing the Gata2+/− mutant mice. This work was supported by NIH grants P01 CA40046, R37 CA72614, T32 CA108462, and R01 HL092471, by the Kenneth and Mary Ellen Wilson St. Baldrick's Research Grant, by a Specialized Center of Research award from the Leukemia and Lymphoma Society (LLS 7019-04), and by the ALSAC of St. Jude Children's Research Hospital. KS is an American Cancer Society Research Professor, MRB is supported by the American Cancer Society Hillcrest Fellowship, and EP is a Leukemia and Lymphoma Society Scholar.
Ethics
Animal experimentation: Study mice were housed in a specific pathogen-free facility at the University of California San Francisco, and all animal experiments were conducted in strict accordance with the protocols approved by the Institutional Animal Care and Use Committee (IACUC) of the University of California, San Francisco (Approval number: AN091877-03).
Copyright
© 2015, Wong et al.
This article is distributed under the terms of the Creative Commons Attribution License, which permits unrestricted use and redistribution provided that the original author and source are credited.
Metrics
-
- 2,053
- views
-
- 388
- downloads
-
- 17
- citations
Views, downloads and citations are aggregated across all versions of this paper published by eLife.
Citations by DOI
-
- 17
- citations for umbrella DOI https://doi.org/10.7554/eLife.07839