α-actinin accounts for the bioactivity of actin preparations in inducing STAT target genes in Drosophila melanogaster
Abstract
Damage-associated molecular patterns (DAMPs) are molecules exposed or released by dead cells that trigger or modulate immunity and tissue repair. In vertebrates, the cytoskeletal component F-actin is a DAMP specifically recognised by DNGR-1, an innate immune receptor. Previously we suggested that actin is also a DAMP in Drosophila melanogaster by inducing STAT-dependent genes (Srinivasan et al., 2016). Here, we revise that conclusion and report that α-actinin is far more potent than actin at inducing the same STAT response and can be found in trace amounts in actin preparations. Recombinant expression of actin or α-actinin in bacteria demonstrated that only α-actinin could drive the expression of STAT target genes in Drosophila. The response to injected α-actinin required the same signalling cascade that we had identified in our previous work using actin preparations. Taken together, these data indicate that α-actinin rather than actin drives STAT activation when injected into Drosophila.
https://doi.org/10.7554/eLife.38636.001Introduction
Metazoan organisms need to be able to recognise damaged tissues in order to induce processes that promote tissue repair and that prevent infection caused by barrier breach. Tissue damage is generally accompanied by cell death and release of damage-associated molecular patterns (DAMPs), molecules that are sequestered within healthy cells but become exposed to the extracellular milieu upon loss of membrane integrity and that trigger inflammation, modulate immunity or promote tissue repair (Rock et al., 2010; Zelenay and Reis e Sousa, 2013). Examples of DAMPs include ATP, uric acid, IL-33, IL1α, RNA and DNA (Zitvogel et al., 2010), as well as actin, a ubiquitous and abundant component of the cytoskeleton of all eukaryotes. Exposure of filamentous (F-) actin by dead cells can be recognised by an innate immune receptor known as DNGR-1 (aka CLEC9A) expressed by specialised leucocytes (Ahrens et al., 2012; Hanč et al., 2015; Zhang et al., 2012). DNGR-1 is only found in mammals but we recently provided evidence that cytoskeletal exposure may be sign of cell damage also in Drosophila melanogaster. In Drosophila, mechanical and other stresses elicit JAK-STAT pathway signalling, which is implicated in stem cell mobilisation and tissue repair (Ekengren and Hultmark, 2001; Ekengren et al., 2001; Agaisse et al., 2003; Brun et al., 2006; Jiang et al., 2009). We found that injection of purified actin into adult flies led to selective induction of STAT target genes in the fat body, the Drosophila equivalent of the mammalian liver (Srinivasan et al., 2016). The response to actin required the NADPH oxidase Nox, the Src family kinase Src42A, and the adapter Shark and led to an autocrine and paracrine amplification loop that involved the cytokine Upd3 and the JAK-STAT-coupled cytokine receptor Domeless (Srinivasan et al., 2016). Here, we revise our interpretation of those data and report that alpha-actinin (α-actinin), a cytoskeletal protein tightly associated with F-actin, is a more potent inducer of STAT target genes than actin. Like the response to actin, the response to α-actinin requires Nox, Src42A and Shark. Notably, α-actinin can be found in trace amounts in the purified actin preparations that we had used in our initial study and recombinant actin expressed in bacteria and devoid of α-actinin is no longer capable of eliciting the STAT response upon injection into flies. We conclude that α-actinin rather than actin is the key trigger of STAT activation upon injection into Drosophila, suggesting that distinct cytoskeletal proteins can serve as DAMPs across species.
Results and discussion
In our previous study, actin purified from human platelets or rabbit muscle or recombinant actin purified from insect cells elicited the expression of STAT-responsive genes in the fat body when injected into adult Drosophila melanogaster (Srinivasan et al., 2016). While assessing the ability of other cytoskeletal proteins to elicit a similar response, we found that myosin, α-actinin, and, to a lesser degree, tubulin could also drive induction of the STAT responsive gene TotM (Figure 1a). On a per molecule basis, α-actinin was the most potent trigger and was superior to myosin, the second most potent inducer (Figure 1a,b). Robust induction of TotM was observed as early as 6 hr post α-actinin injection and was sustained above control levels for at least two days (Figure 1c). The ability of α-actinin to induce STAT-responsive gene induction was independently reproduced in three laboratories (C.R.S, M.D., L.T.), underscoring the robustness of the result (data not shown). Like actin itself, α-actinin is a component of the cytoskeleton in all higher eukaryotes, where it crosslinks and stabilises actin filaments (Ribeiro et al., 2014). Given its association with actin, α-actinin could therefore be present as a contaminant in purified actin preparations, including the ones used in our studies. Consistent with that possibility, mass spectrometry analysis revealed that α-actinin is the major contaminant of purified actin preparations and accounts for approximately 0.4% of total protein (Figure 1d). Western blot analysis revealed the presence of immunodetectable α-actinin in actin preparations, confirming the mass spectrometry results (Figure 1e). Notably, dose response curves showed that α-actinin was >100 fold more potent than actin on a per molecule basis at eliciting the Drosophila STAT response (Figure 1f). Therefore, it is possible that contamination with α-actinin accounts for the activity of injected actin preparations in Drosophila.
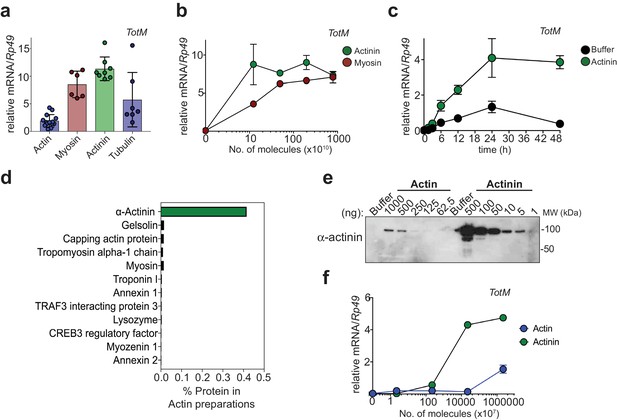
α-actinin is the most potent inducer of STAT-dependent genes.
(a) w1118 flies were injected with equimolar preparations of purified actin, α-actinin, myosin and tubulin (9.4 × 1017 molecules). Relative expression of TotM 24 hr post injection is shown. Data are pooled from two independent experiments with 10 flies/sample with at least triplicate samples. (b) w1118 flies were injected with preparations containing the indicated number of molecules of purified myosin or purified α-actinin. Relative expression of TotM 24 hr post injection is shown. Data are representative of two independent experiments with 10 flies/sample with duplicate samples. (c) w1118 flies were injected with buffer or 3.68 ng of purified α-actinin. Relative expression of TotM over a 48 hr period is shown. Data are representative of two independent experiments with 10 flies/sample with triplicate samples. (d) Purified actin was subjected to mass spectrometry analysis and contaminating proteins are expressed as % of protein preparation. (e) Indicated protein amounts (ng) of purified actin and α-actinin were analysed by western blot using an anti-α-actinin antibody. Data are representative of three independent experiments. (f) w1118 flies were injected with preparations containing the indicated number of molecules of rabbit muscle purified actin or purified α-actinin. Relative expression of TotM 24 hr post injection is shown. Data are representative of three independent experiments with 10 flies/sample with duplicate samples. TotM relative levels were calculated using the housekeeping gene Rp49 as a reference gene. Bars represent mean ± SD.
To address this possibility, we tried to deplete α-actinin from actin preparations. However, we failed to satisfactorily separate actin and α-actinin using multiple approaches, including size exclusion chromatography, ionic strength chromatography or immunodepletion with 14 different antibodies (data not shown). We therefore pursued an alternative strategy of testing recombinant actin and α-actinin expressed in BL21 E. coli, an organism that does not have an actin-based cytoskeleton and in which, therefore, co-purification of α-actinin with actin is not possible. We first assessed the functional integrity of the recombinant proteins purified from bacteria. Using a pelleting assay (Ahrens et al., 2012), we confirmed that recombinant actin was able to form filaments when incubated in polymerisation-favouring conditions (Figure 2a) and that α-actinin was able to associate with those filaments (Figure 2b). Having ascertained that actin and α-actinin are functional and, therefore, correctly folded, we next tested whether they could elicit the expression of STAT-responsive genes in Drosophila. Strikingly, injection of bacterially-expressed actin did not elicit the expression of TotM or of another STAT target gene, Diedel, unlike actin purified from rabbit muscle (Figure 2c). In contrast, bacterially-expressed α-actinin, like α-actinin purified from rabbit muscle, elicited the expression of various STAT-responsive genes, including TotM, TotA and Diedel, in a dose-dependent manner (Figure 2d and data not shown). Neither recombinant bacterially-expressed nor rabbit musclederived α-actinin elicited the expression of Drs and Dpt, Toll and Imd target genes, respectively, 24 hr post injection (Figure 2—figure supplement 1a), indicating that the proteins were devoid of microbial contaminants and confirming specificity for STAT targets. Lack of expression of Drs and Dpt by α-actinin was confirmed at multiple timepoints within a 48 hr period (Figure 2—figure supplement 1b). In contrast, septic injury (Escherichia coli and Micrococcus luteus) induced both Drs and Dpt by 24 hr (Figure 2—figure supplement 1c). Finally, RNAi-mediated knockdown of Nox, Src42A and Shark using three fat body-restricted drivers (c564, r4 and Lpp) abrogated the induction of STAT-responsive genes in response to injection of α-actinin (Figure 3a), whereas knockdown of Nox, Src42A and Shark using a haemocyte-restricted driver (HmlΔ) did not alter the response (Figure 3b). Therefore, injection of bacterially-expressed or rabbit muscle α-actinin into Drosophila melanogaster is sufficient to induce the same response that we previously described to be elicited by injection of actin preparations. Taken together, these data suggest that α-actinin is a major bioactive component in cytoskeletal protein preparations, including actin.
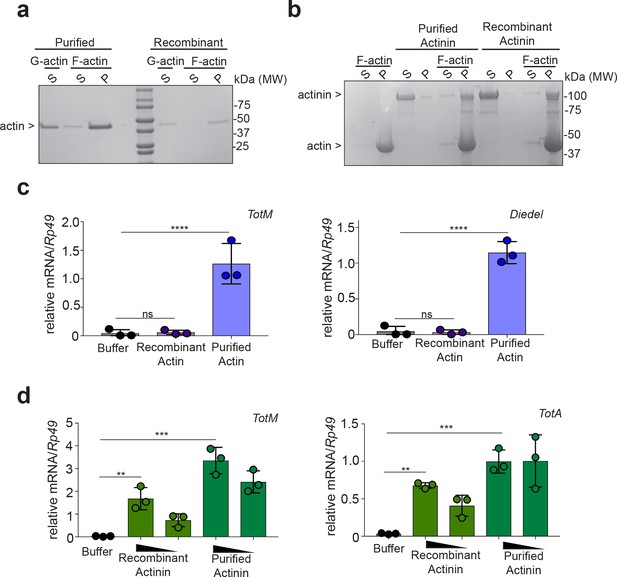
Bacterially-expressed recombinant α-actinin but not actin induces STAT-responsive genes.
(a) Actin purified from rabbit muscle or recombinantly made in bacteria was incubated in either G-actin or F-actin buffers before subjecting samples to ultracentrifugation to separate globular and filamentous actin. Supernatant (S) and pelleted (P) fractions were analysed by Tris-Gylcine gels and visualized by Coomassie staining. Data are representative of two independent experiments. (b) α-actinin from rabbit muscle or recombinantly made in bacteria was added or not to polymerised F-actin. Then, samples were subjected to ultracentrifugation, and proteins in the supernatant (S) or pellet (P) were analysed by Tris-Gylcine gels and visualized by cCoomassie staining. (c) w1118 flies were injected with PBS buffer or equal amounts of bacterially-expressed recombinant or rabbit muscle purified actin (11.04 ng per fly). Relative expression of TotM and Diedel 24 hr post injection is shown. Data are representative of two independent experiments with 10 flies/sample with triplicate samples. (d) w1118 flies were injected with PBS buffer, or equal amounts of bacterially-expressed recombinant or rabbit muscle purified α-actinin (3680 pg or 368 pg per fly). Relative expression of TotM and TotA 24 hr post injection is shown. Data are representative of two independent experiments with 10 flies/sample with triplicate samples. TotM relative levels were calculated using the housekeeping gene Rp49 as a reference gene. Bars represent mean ± SD. Statistical analysis was performed using one-way ANOVA with Sidak’s multiple comparison test as post-test for pairwise comparisons. Significant differences with Sidak’s multiple comparison test are shown (ns, not significant; *p<0.05; **p<0.01; ***p<0.001; ****p<0.0001).
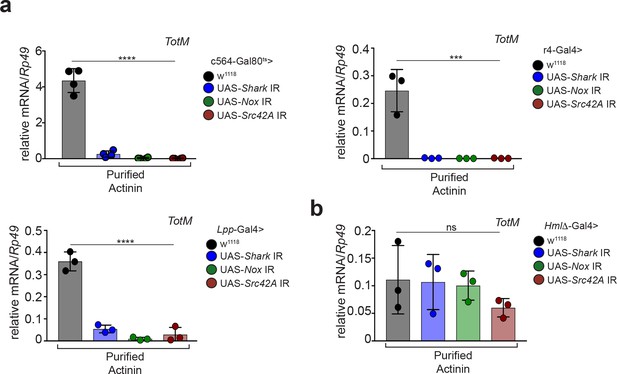
α-actinin induced STAT-dependent genes require Nox, Shark and Src42A expression in the fat body.
(a) Flies in which Shark, Nox, or Src42A were knocked down in the fat body using three different driver lines (c564-Gal80ts, r4-Gal4 or Lpp-Gal4), or control flies lacking UAS-target sequences, were injected with either PBS buffer or rabbit muscle purified α-actinin (13.8 pg per fly). Relative expression of TotM 24 hr post injection is shown. Data are representative of two independent experiments with 5 – 10 flies/sample with at least triplicate samples. (b) Flies in which Shark, Nox, or Src42A were knocked down in haemocytes (HmlΔ-Gal4), or control flies lacking UAS-target sequences, were injected with either PBS buffer or rabbit muscle purified α-actinin (13.8 pg per fly). Relative expression of TotM 24 hr post injection is shown. Data are representative of two independent experiments with 5 – 10 flies/sample with triplicate samples. TotM relative levels were calculated using the housekeeping gene Rp49 as a reference gene. Bars represent mean ± SD. Statistical analysis was performed using one-way ANOVA with Sidak’s multiple comparison test as post-test for pairwise comparisons. Significant differences with Sidak’s multiple comparison test are shown (ns, not significant; *p<0.05; **p<0.01; ***p<0.001; ****p<0.0001).
We have previously reported that actin injection, mimicking extracellular cytoskeletal exposure, can trigger the expression of STAT target genes in Drosophila (Srinivasan et al., 2016). Actin is difficult to produce as a recombinant protein in bacteria and commercially-available actin (>99% pure) is traditionally purified from rabbit muscle or human platelets. Here, we demonstrate that the activity of such actin preparations is likely attributable to trace amounts of co-purifying α-actinin. This conclusion is supported by several findings: (1) α-actinin engages the same fat body Nox/Src42A/Shark pathway previously described for injected actin; (2) contamination with α-actinin can account stoichiometrically for the activity of actin preparations, α-actinin being more than one-hundred fold more potent that actin; (3) recombinant α-actinin expressed in bacteria retains activity while activity is lost for bacterially-expressed actin. These new findings support our earlier conclusion that exposure of cytoskeletal components is an evolutionarily-conserved sign of cell damage but suggest that the nature of the cytoskeletal element acting as a DAMP can vary across species. Thus, while (F-) actin is sensed by at least one mammalian receptor, DNGR-1, in Drosophila melanogaster α-actinin appears to act as the dominant trigger for the STAT-dependent response we have studied. Consistent with the notion that α-actinin may be the common denominator of the response to extracellular proteins in Drosophila, preliminary mass spectrometry analysis suggests that trace amounts of α-actinin are also found in tubulin and myosin preparations (data not shown). The inability to deplete α-actinin using antibodies makes it difficult to address the issue of contamination, especially in the case of large multi-subunit proteins such as myosin for which recombinant expression in bacteria is not feasible. More work is needed to clarify whether any cytoskeletal proteins besides α-actinin act as inducers of STAT-responsive genes in Drosophila and to identify the putative receptor(s) responsible for initiating the response.
Materials and methods
Fly maintenance and injections
Request a detailed protocolFly maintenance, breeding, transgene induction, injections, septic injury, RNA extraction, cDNA synthesis and quantitative real-time PCR were performed as previously described (Srinivasan et al., 2016).
The following stocks were used:
Fly stock | Description |
---|---|
w1118 | Control strain |
;UAS-Shark IR; (Shark Fr RNAi) | Interfering RNA for knockdown of Shark. Kindly donated by Marc Freeman. |
w;c564-Gal4;Tub-Gal80ts | Temperature-sensitive fat body-specific driver line. |
;UAS-Src42A IR | VDRC ID: 100708 |
;UAS-Nox IR | VDRC ID: 100753 |
;;r4-Gal4/TM6C.Sb1 | Fat body-specific driver line |
;;Lpp-Gal4/TM6.Sb1 | Fat body-specific driver line |
HmlΔ-Gal4/CyO | Haemocyte-specific driver |
Purified cytoskeletal proteins
Request a detailed protocolPurified cytoskeletal proteins were reconstituted and stored as advised by the supplier (Cytoskeleton Inc).
Bacterial expression of cytoskeletal proteins
Request a detailed protocolRosetta-2 BL21 E. coli bacteria were transformed with pET8c-His6-human Actinin-2 as described (Ribeiro et al., 2014). A starter culture was made by inoculating Ampicillin-containing LB with a well-defined colony and incubating overnight at 37°C. Large-scale expression was carried out using Overnight Express LB medium (Novagen) supplemented with 10 ml glycerol inoculated with 2 ml of the starter culture. Cell growth and protein expression was carried out at 30°C for 16 hr. Cells from these high-density shaking cultures (OD600 > 8 A.U) were harvested by centrifugation for 15 min at 7500 x g. For purification of the His-tagged protein, the bacterial pellet was re-suspended in 50 ml of lysis buffer containing 50 mM Tris-HCl (pH 8.0), 150 mM NaCl, 0.1% Triton X-100 and antiproteases (Roche). Cells were disrupted by sonication (5 × 30 s) and lysates were centrifuged at 18,000 x g for 30 min at 4°C. Clarified lysates were incubated with 3 ml Ni-NTA agarose at 4°C for 16 hr on rotation. Beads were washed three times for 5 min at 4 ˚C with 25 ml wash buffer (50 mM Tris-HCl (pH 7.5), 150 mM NaCl, antiproteases, 5 mM Imidazole) . After the final wash, beads were incubated with 10 ml buffer A to elute His-tagged proteins (50 mM Tris-HCl (pH 7.5), 150 mM NaCl, antiproteases, 2 mM beta-mercaptoethanol, 250 – 500 mM Imidazole). Two elutions were collected and subsequently purified to homogeneity by anion exchange and size exclusion chromatography. To that end, α-actinin eluted from the Ni-NTA affinity resin was dialysed into buffer containing 50 mM Tris-HCl (pH 7.5), 2 mM beta-mercaptoethanol and applied to a Mono Q 5/50 anion exchange column (GE Life Sciences) equilibrated with 50 mM Tris (pH 7.5) and 1 mM DTT. Bound α-actinin was eluted by application of a NaCl gradient from 0 to 1M over 30 column volumes. The peak containing α-actinin was determined by SDS-PAGE, the relevant fractions pooled and concentrated to 0.5 ml and applied to a S75 10/300 size exclusion column equilibrated with PBS (pH 7.4) and 1 mM DTT. Fractions containing α-actinin were pooled and stored until required. Recombinant bacterially-expressed human smooth muscle actin was purchased from a commercial source (ab134555, Abcam).
Mass spectrometry
Request a detailed protocolProtein preparations were subjected to SDS/PAGE and migrated approximately 2 cm into the gel. The gel lanes were excised and proteins were in-gel digested using trypsin. Tryptic peptides were analysed with an LTQ Orbitrap-Velos mass spectrometer coupled to an Ultimate3000 HPLC equipped with an EASY-Spray nanosource (Thermo Fisher Scientific). Raw data was processed using MaxQuant v1.3.05 with intensity based absolute quantification (iBAQ) selected as the quantification algorithm. The output table was imported into Perseus software v1.4.0.2, and percentages of contamination were calculated using the untransformed iBAQ values.
Actin pelleting assay
Request a detailed protocolPerformed as described by the supplier (Cytoskeleton). Briefly, actin was diluted in modified G-actin buffer (5 mM Tris HCl pH 8.0, 0.2 mM CaCl2, 0.2 mM ATP, 0.5 mM DTT) and incubated on ice for 60 min for complete depolymerisation of actin oligomers. Subsequently, samples were supplemented with F-actin buffer (10 mM Tris-HCl pH 7.5, 50 mM KCl, 2 mM MgCl2 and 1 mM ATP) incubated for 60 min at RT. Samples were then centrifuged for 1 hr at 100,000 x g, and protein levels in the supernatant and pellet were assessed by SDS-PAGE and Coomassie staining. For α-actinin pelleting assays the same protocol was used, with the only modification that α-actinin was added to the polymerising actin filaments.
Western blotting
Request a detailed protocolProtein samples were resuspended in 1X Laemmli SDS-PAGE buffer and boiled for 7 min at 95°C. Protein was resolved on Tris-Glycine 4 – 20% precast gels (Bio-Rad). α-actinin and actin was detected via immunoblotting with anti-actin (Clone C4, MAB1501, Millipore) and anti-actinin (3134S, Cell Signaling Technology) antibodies.
Data availability
Data generated or analysed during this study are included in the manuscript. Mass spectrometry data were uploaded as supporting file.
References
-
A family of Turandot-related genes in the humoral stress response of DrosophilaBiochemical and Biophysical Research Communications 284:998–1003.https://doi.org/10.1006/bbrc.2001.5067
-
A humoral stress response in DrosophilaCurrent Biology 11:714–718.https://doi.org/10.1016/S0960-9822(01)00203-2
-
The sterile inflammatory responseAnnual Review of Immunology 28:321–342.https://doi.org/10.1146/annurev-immunol-030409-101311
-
Adaptive immunity after cell deathTrends in Immunology 34:329–335.https://doi.org/10.1016/j.it.2013.03.005
Article and author information
Author details
Funding
Francis Crick Institute (FC001136)
- Caetano Reis e Sousa
Wellcome Trust (WT106973MA)
- Caetano Reis e Sousa
Federation of European Biochemical Societies
- Conor M Henry
Fundação para a Ciência e Tecnologia (PTDC/BEX- GMG/3128/2014)
- Luis Teixeira
The funders had no role in study design, data collection and interpretation, or the decision to submit the work for publication.
Acknowledgements
We thank Nic Tapon, Paul Martin, Maxine Holder, Georgina Fletcher, Ieva Gailite and members of the Immunobiology Laboratory for helpful discussions and suggestions. We are grateful to Kristina Djinovic-Carugo for the α-actinin plasmid. We thank the Francis Crick Institute Genomics Equipment Park, Proteomics, Structural Biology, and the Fly facility for assistance. We also thank the Vienna Drosophila Resource Center for Drosophila lines and Flybase for online resources. This work was supported by The Francis Crick Institute, which receives core funding from Cancer Research UK (FC001136), the UK Medical Research Council (FC001136), and the Wellcome Trust (FC001136), and by Investigator Award WT106973MA from the Wellcome Trust to CRS. LT is funded by the Fundação para a Ciência e Tecnologia (www.fct.pt) grant PTDC/BEX- GMG/3128/2014. CMH was supported by a long-term fellowship from the Federation of European Biochemical Societies (FEBS).
Copyright
© 2018, Gordon et al.
This article is distributed under the terms of the Creative Commons Attribution License, which permits unrestricted use and redistribution provided that the original author and source are credited.
Metrics
-
- 1,573
- views
-
- 205
- downloads
-
- 27
- citations
Views, downloads and citations are aggregated across all versions of this paper published by eLife.
Citations by DOI
-
- 27
- citations for umbrella DOI https://doi.org/10.7554/eLife.38636