Multi-protein bridging factor 1(Mbf1), Rps3 and Asc1 prevent stalled ribosomes from frameshifting
Abstract
Reading frame maintenance is critical for accurate translation. We show that the conserved eukaryotic/archaeal protein Mbf1 acts with ribosomal proteins Rps3/uS3 and eukaryotic Asc1/RACK1 to prevent frameshifting at inhibitory CGA-CGA codon pairs in the yeast Saccharomyces cerevisiae. Mutations in RPS3 that allow frameshifting implicate eukaryotic conserved residues near the mRNA entry site. Mbf1 and Rps3 cooperate to maintain the reading frame of stalled ribosomes, while Asc1 also mediates distinct events that result in recruitment of the ribosome quality control complex and mRNA decay. Frameshifting occurs through a +1 shift with a CGA codon in the P site and involves competition between codons entering the A site, implying that the wobble interaction of the P site codon destabilizes translation elongation. Thus, eukaryotes have evolved unique mechanisms involving both a universally conserved ribosome component and two eukaryotic-specific proteins to maintain the reading frame at ribosome stalls.
https://doi.org/10.7554/eLife.39637.001eLife digest
Proteins perform all the chemical reactions needed to keep a cell alive; thus, it is essential to assemble them correctly. They are made by molecular machines called ribosomes, which follow a sequence of instructions written in genetic code in molecules known as mRNAs. Ribosomes essentially read the genetic code three letters at a time; each triplet either codes for the insertion of one of 20 building blocks into the emerging protein, or serves as a signal to stop the process. It is critical that, after reading one triplet, the ribosome moves precisely three letters to read the next triplet. If, for example, the ribosome shifted just two letters instead of three – a phenomenon known as “frameshifting” – it would completely change the building blocks that were used to make the protein. This could lead to atypical or aberrant proteins that either do not work or are even toxic to the cell.
For a variety of reasons, ribosomes will often stall before they have finished building a protein. When this happens, the ribosome is more likely to frameshift. Cells commonly respond to stalled ribosomes by recruiting other molecules that work as quality control systems, some of which can disassemble the ribosome and break down the mRNA. In budding yeast, one part of the ribosome – named Asc1 – plays a key role in recruiting these quality control systems and in mRNA breakdown. If this component is removed, stalled ribosomes frameshift more frequently and, as a result, aberrant proteins accumulate in the cell. Since the Asc1 recruiter protein sits on the outside of the ribosome, it seemed likely that it might act through other factors to stop the ribosome from frameshifting when it stalls. However, it was unknown if such factors exist, what they are, or how they might work.
Now, Wang et al. have identified two additional yeast proteins, named Mbf1 and Rps3, which cooperate to stop the ribosome from frameshifting after it stalls. Rps3, like Asc1, is a component of the ribosome, while Mbf1 is not. It appears that Rps3 likely stops frameshifting via an interaction with the incoming mRNA, because a region of Rps3 near the mRNA entry site to the ribosome is important for its activity. Further experiments then showed that the known Asc1-mediated breakdown of mRNAs did not depend on Mbf1 and Rps3, but also assists in stopping frameshifting. Thus, frameshifting of stalled ribosomes is prevented via two distinct ways: one that directly involves Mbf1 and Rps3 and one that is promoted by Asc1, which reduces the amounts of mRNAs on which ribosomes frameshift.
These newly identified factors may provide insights into the precisely controlled protein-production machinery in the cell and into roles of the quality control systems. An improved understanding of mechanisms that prevent frameshifting could eventually lead to better treatments for some human diseases that result when these processes go awry, which include certain neurological conditions.
https://doi.org/10.7554/eLife.39637.002Introduction
Accurate translation of mRNA into protein depends upon precise, repetitive three base translocation of the ribosome to maintain the correct reading frame throughout a coding sequence. Reading frame maintenance is challenging because multiple movements of the tRNAs and mRNA as well as conformational changes within the ribosome itself are required to complete a single elongation cycle (Noller et al., 2017). For instance, the tRNA acceptor stems move within the large subunit during formation of the hybrid state, while the joining of EF-G-GTP (eEF2 in eukaryotes) results in additional movement of tRNA, and finally completion of translocation, driven by Pi release, requires additional movements (Belardinelli et al., 2016; Brilot et al., 2013; Noller et al., 2017; Pulk and Cate, 2013; Ramrath et al., 2013; Ratje et al., 2010; Tourigny et al., 2013; Zhou et al., 2014). To accomplish this cycle, many interactions between the tRNAs and ribosome are disrupted, and new interactions are created, but the relative position of the tRNA anticodon to the mRNA codon must be maintained throughout all of these events (Noller et al., 2017; Dever et al., 2018; Rodnina, 2018). Thus, it is critical that mechanisms exist to prevent slippage during these transitions.
Reading frame maintenance is facilitated by structures within the ribosome as well as by tRNA modifications. Structural features that contribute to reading frame maintenance, inferred from analysis of prokaryotic translation intermediates, include a swivel of the 30S head relative to the 30S body to form a contracted mRNA tunnel downstream of the A site prior to translocation (Jenner et al., 2010; Schuwirth et al., 2005). In addition, during translocation, two conserved bases in the 16S rRNA intercalate into different positions of the mRNA to prevent slippage (Zhou et al., 2013), while domain IV of EF-G contacts the codon and tRNA in the A/P site and h44 of 16S rRNA, likely coupling mRNA and tRNA movement (Ramrath et al., 2013; Zhou et al., 2014). tRNA modifications within the anticodon loop also assist in reading frame maintenance, inferred both from genetic and structural analyses. Mutants that affect several such modifications in both bacteria and eukaryotes result in increased frameshifting (Atkins and Björk, 2009; Jäger et al., 2013; Tükenmez et al., 2015; Urbonavicius et al., 2001; Waas et al., 2007). Moreover, a cross-strand base stacking interaction between a modified ms2i6A37 in an E. coli tRNAPhe and the mRNA codon is proposed to prevent slippage of P site tRNA on the mRNA (Jenner et al., 2010). Thus, a number of mechanisms exist to prevent loss of reading frame.
Nevertheless, ribosomes do move into alternative reading frames in response to specific sequences and structures in mRNA (Atkins and Björk, 2009; Dever et al., 2018; Dinman, 2012). The existence of such events has implied that ribosomal plasticity with respect to reading frame movement is an integral function of the translation machinery. The common feature of all frameshifting events in bacteria to humans is that the ribosome stalls (Dever et al., 2018). The stall can be mediated by combined effects of the A and P site codons (Farabaugh et al., 2006; Gamble et al., 2016), by the presence of downstream structures, or by an upstream Shine-Dalgarno sequence in bacteria (Caliskan et al., 2014; Dinman, 2012). Analysis of programmed frameshifting indicates that additional sequences or protein factors are frequently required to mediate efficient frameshifting (Atkins and Björk, 2009; Dinman, 2012). For instance, +1 programmed frameshifting events are frequently enhanced by stimulatory sequences, although the role of these sequences is not always clear (Guarraia et al., 2007; Taliaferro and Farabaugh, 2007).
The identification of mutants that either affect programmed frameshifting or suppress frameshift mutations has pointed to four key factors in reading frame maintenance. First, mutations of ribosomal proteins, particularly those that contact the P site tRNA can cause increased frameshifting. In bacteria, frameshifting mutations are suppressed by deletions within the C terminal domain of ribosomal protein uS9, which contacts the P site tRNA anticodon loop (Jäger et al., 2013). In the yeast Saccharomyces cerevisiae, programmed frameshifting in the L-A virus is affected by mutations in 5S rRNA or its interactors uL18 or uL5, that also contact the P site tRNA (Meskauskas and Dinman, 2001; Rhodin and Dinman, 2010; Smith et al., 2001). Frameshifting mutations are also suppressed by a mutation in the yeast RPS3, although this mutation does not affect a tRNA contact (Hendrick et al., 2001). Second, mutations in the basal translation machinery can also affect frameshifting. For instance, frameshifting mutations are suppressed by mutations in both EF-1α, which delivers tRNA to the ribosome (Sandbaken and Culbertson, 1988), and in SUP35, encoding the translation termination factor eRF3 (Wilson and Culbertson, 1988). Third, miRNAs can affect the efficiency of programmed frameshifting, for instance at CCR5 in humans (Belew et al., 2014). Fourth, mutations that affect proteins with previously unknown functions in translation can either alter programmed frameshifting or suppress frameshifting mutations. For instance, in yeast, frameshifting mutations are suppressed by mutations in MBF1, encoding Multi-protein Bridging Factor 1 (Hendrick et al., 2001), or in EBS1 (Ford et al., 2006), while in the porcine virus PRRSV, the RNA binding protein nsp1β stimulates both −1 and −2 frameshifting events (Li et al., 2014). Thus, reading frame maintenance is modulated by ribosomal components, many of which contact the tRNAs, as well as by non-ribosomal proteins and miRNAs. However, the roles of many of these proteins are not understood.
We set out to work out the mechanisms that maintain reading frame when eukaryotic ribosomes encounter a stall, the common feature of all frameshifting events. In bacteria, ribosome stalls due to limited availability or functionality of tRNA seem to suffice to cause frameshifting (Gurvich et al., 2005; Seidman et al., 2011). In wild type yeast, ribosomes stall at CGA codon repeats, which inhibit translation due to wobble decoding of CGA by its native tRNAArg(ICG) (Letzring et al., 2010; Letzring et al., 2013). Although frameshifting was detected at several underrepresented heptanucleotide sequences in yeast (Shah et al., 2002), it was not detected at CGA codon repeats (Wolf and Grayhack, 2015). Instead, eukaryotes have evolved new pathways to regulate inefficient translation events, such as the Ribosome Quality Control (RQC) pathway, in which these stalled ribosomes undergo ubiquitination of ribosomal proteins, followed by dissociation of the subunits, and recruitment of the RQC Complex, which mediates CAT tailing and degradation of the nascent polypeptide (Bengtson and Joazeiro, 2010; Brandman and Hegde, 2016; Brandman et al., 2012; Defenouillère et al., 2013; Joazeiro, 2017; Kostova et al., 2017; Shao et al., 2013; Shen et al., 2015; Verma et al., 2013; Wilson et al., 2007; Juszkiewicz and Hegde, 2017; Matsuo et al., 2017; Simms et al., 2017b; Sitron et al., 2017; Sundaramoorthy et al., 2017). The ribosomal protein Asc1/RACK1 mediates these events (Brandman et al., 2012; Kuroha et al., 2010); in the absence of Asc1, ribosomes fail to engage the RQC (Sitron et al., 2017), and also undergo substantial frameshifting at CGA codon repeats (Wolf and Grayhack, 2015). However, Asc1 sits on the outside of the ribosome at the mRNA exit tunnel and likely functions as scaffold for recruitment of other proteins, such as the E3 ubiquitin ligase Hel2/mammalian ZNF598 and Slh1 (Juszkiewicz and Hegde, 2017; Matsuo et al., 2017; Simms et al., 2017b; Sitron et al., 2017; Sundaramoorthy et al., 2017). Based on the location of Asc1 and the precedent that Asc1 recruits other proteins to abort translation, we considered it likely that Asc1 cooperates with additional proteins to mediate reading frame maintenance at CGA codon repeats and set out to find such factors.
Here, we provide evidence that the Multi-protein Bridging Factor 1 (Mbf1) and ribosomal proteins Rps3 and Asc1 (homolog of human Rack1) work together to prevent translational slippage at CGA codon repeats. Frameshifting results from inactivation of MBF1, or from mutations in amino acids in Rps3 located on an exposed surface of the protein near the mRNA entry site. Asc1 was previously known to mediate recruitment of the RQC, mRNA cleavage and mRNA decay at similar stall sites (Ikeuchi and Inada, 2016; Kuroha et al., 2010; Letzring et al., 2013; Sitron et al., 2017), as well as reading frame maintenance (Wolf and Grayhack, 2015). We provide evidence that Asc1 and Mbf1 cooperate to mediate reading frame maintenance at similar sites, acting on a common set of substrates, including the seven most slowly translated codon pairs in yeast (Gamble et al., 2016). We examined the precise frameshift at one of these inhibitory pairs, CGA-CGG, purifying the frameshifted polypeptide, followed by analysis with mass spectrometry. We found that frameshifting occurs in the +1 direction at the CGA codon and moreover, that frameshifting is modulated by the competition between the in-frame and +1 frame tRNAs.
Results
MBF1 (Multi-protein Bridging Factor 1) prevents frameshifting at CGA codon repeats
We considered it likely that proteins other than Asc1 worked to prevent frameshifting at CGA codon repeats for two reasons. First, Asc1 binds on the outside of the ribosome, not in the decoding center (Rabl et al., 2011), and thus is not positioned in any obvious way to assist with reading frame maintenance. Second, Asc1 recruits other proteins, Hel2 and Slh1, to recruit the RQC (Brandman and Hegde, 2016; Joazeiro, 2017; Sitron et al., 2017), and thus is likely to work with other proteins in reading frame maintenance. Thus, we set out to identify genes responsible for reading frame maintenance at CGA codon repeats.
To isolate mutants that frameshift due to translation of CGA codon repeats, we set up a selection in which a +1 frameshift (caused by 6 adjacent CGA codons) was required to express the URA3 gene. The native URA3 gene was placed in the +1 reading frame downstream of an N-terminal domain of GLN4 encoding amino acids 1–99 (GLN4(1-99)), followed by 6 CGA codons and one additional nucleotide upstream of the URA3 coding region (Figure 1A). This strain exhibits an Ura- phenotype, due to the low levels of frameshifting in an otherwise wild-type background, allowing for an Ura+ selection to obtain mutants with increased frameshifting. As an independent secondary screen for frameshifting mutants due to CGA codon repeats, we integrated a modified version of the RNA-ID reporter with GLN4(1-99) followed by 4 CGA codons and one additional nucleotide upstream of the GFP coding region into the ADE2 locus (Dean and Grayhack, 2012; Wolf and Grayhack, 2015). Thus, GFP expression was dependent upon frameshifting efficiency (Figure 1A). To avoid obtaining mutations in the ASC1 gene, the selection strain also contained a second copy of the ASC1 gene on a plasmid. (Figure 1A). We selected Ura+ mutants from 40 independent cultures each of MATa and MATα parents and then analyzed three Ura+ mutants from each culture by flow cytometry to measure GFP and RFP expression. Most mutants (60% of MATα mutants and 80% of MATa mutants) showed elevated expression of GFP, and we studied those that exhibited relatively high levels of frameshifting, >30% of that in an asc1Δ mutant (Figure 1B). Most mutants (43 of 48 examined) were recessive and mapped to a single complementation group, based on growth of diploids on media lacking uracil (Figure 1—figure supplement 1A), although four dominant mutants were also identified.
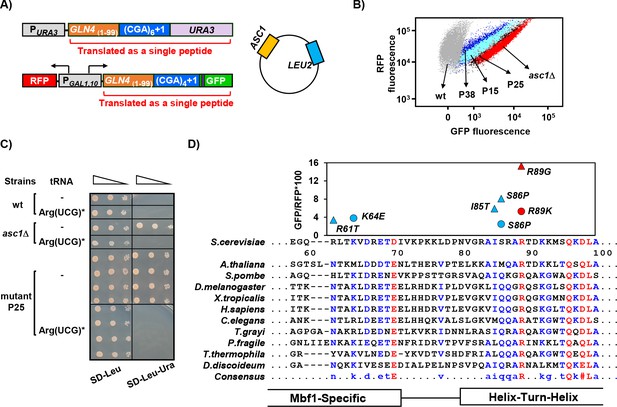
MBF1 (Multi-protein Bridging Factor 1) prevents frameshifting at CGA codon repeats.
(A) Schematic of selection for mutants that frameshift at CGA codon repeats. The indicated CGA codon repeats plus one extra nucleotide were inserted upstream of the URA3 and GFP coding region (with an upstream HA tag shown in purple), resulting in an Ura- GFP- parent strain. Additional copies of the ASC1 gene were introduced on a LEU2 plasmid to avoid recessive mutations in the native ASC1 gene. To obtain mutants with increased frameshifting efficiency, Ura+ mutants were selected and screened for increased GFP/RFP. (B) Expression of GLN4(1-99)-(CGA)4+1-GFP is increased in the MATa Ura+ mutants from this selection. Flow cytometry scatter plot showing GFP fluorescence versus RFP fluorescence for three mutants from this selection (P15: mbf1-R89K, light blue; P25: mbf1Δ125–151, black; P38: mbf1-K64E, dark blue), for the asc1Δ mutant (red) and for the wild-type parent strain (gray). (C) Expression of the non-native tRNAArg(UCG)* suppressed the Ura+ phenotype of mutant P25 at 30°C. Serial dilutions of the indicated strains with empty vector or expressing the mutant tRNAArg(UCG)* were grown on the indicated media. (D) Mutations in the MBF1 mutants map in conserved amino acids in both the MBF1-specific domain and the Helix-Turn-Helix (HTH) domain of Mbf1 protein. Alignment of yeast Mbf1 amino acids 60–100 with other eukaryotic species is shown (full alignment see Figure 1—figure supplement 3A). GFP/RFP of frameshifted (CGA)4+1 reporter is shown for mutants obtained from MATa (circles) and MATα (triangles) strains, with the color of markers corresponding to the consensus level of this residue (Blue: 50–90%, Red: >90%), however the conserved residue for R61 is N, and for S86 is Q, with all others identical to yeast.
To confirm that inhibitory decoding of CGA codon repeats is required for frameshifting in these mutants, we showed that introduction of an anticodon-mutated exact match tRNAArg(UCG)* suppressed the Ura+ phenotype of one mutant (Figure 1C). We have shown previously that expression of this exact match tRNAArg(UCG)* results in efficient decoding of CGA codons and suppresses their inhibitory effects on gene expression (Letzring et al., 2010). Thus, the Ura+, GFP+ phenotype of this mutant was due to frameshifting that occurs when the ribosome translates CGA codon repeats inefficiently.
We demonstrated that mutations in the yeast gene MBF1, Multi-protein Bridging Factor 1, were responsible for the defects in reading frame maintenance in recessive high GFP mutants. We identified the mutated gene by complementation of the Ura+ phenotype of the P25 recessive mutant with two plasmids from a library that contains 97.2% of the entire yeast genome (Figure 1—figure supplement 1B) (Jones et al., 2008). The complementing plasmids share a single ORF, MBF1. We confirmed that mutations in the MBF1 gene are responsible for frameshifting in three ways. First, a plasmid with only the MBF1 gene complemented the frameshifting Ura+ phenotype of two mutants (Figure 1—figure supplement 2A). Second, deletion of MBF1 in the parent selection strain (Figure 1A) converted that strain from GFP- to GFP+, similar to deletion of ASC1 (Figure 1—figure supplement 2B). Third, 19/19 mutants tested contain mutations in the MBF1 gene. Point mutations isolated in our selection are located at conserved residues near the junctions between two domains (Figure 1D).
MBF1 is a highly conserved gene in eukaryotes and archaea, generally less than 160 amino acids with an N-terminal Mbf1-specific domain (that differs between archaea and eukaryotes) and a conserved cro-like helix-turn-helix (HTH) domain (Figure 1D, Figure 1—figure supplement 3A). Mbf1, which was initially identified as a transcription co-activator in Bombyx mori (Li et al., 1994; Takemaru et al., 1997), has been implicated in a similar function in yeast, in this case, interacting with the Gcn4, transcription regulator of the general amino acid control pathway (Takemaru et al., 1998). In testing sensitivity to 3-aminotriazole (3-AT) (Hilton et al., 1965; Schürch et al., 1974), a phenotype of gcn4 mutants due to inability to induce expression of HIS3, we found that two frameshifting point mutants (mbf1-K64E and mbf1-I85T) exhibit no growth defect even on high concentrations of 3-AT (Figure 1—figure supplement 3B). Moreover, deletion of GCN4 does not affect frameshifting at CGA codon repeats in an asc1Δ mutant (Wolf and Grayhack, 2015). Thus, it is unlikely that the defect in reading frame maintenance in our mbf1 mutants is related to GCN4. However, Mbf1 has also been implicated in translation, based on isolation of mutations in yeast MBF1 that suppress frameshifting mutations (Hendrick et al., 2001), and the weak association of the archaeal homolog with ribosomes (Blombach et al., 2014), but there is no information on its molecular role in translation.
Ribosomal protein Rps3 also mediates reading frame maintenance at CGA codon repeats
To identify the mutated gene(s) in our dominant mutants, we performed whole genome sequencing in two MATα mutants and found that each mutant contains a single amino acid change (S104Y and G121D) in RPS3. Similarly, the two dominant MATa mutants also contain mutations in the RPS3 gene (L113F and a duplication of N22 to A30). RPS3 encodes a universally conserved ribosomal protein, a core component of the mRNA entry tunnel with a eukaryotic-specific C-terminal extension that interacts with Asc1 (Rabl et al., 2011). One known mutation in RPS3 (K108E) affects reading frame maintenance (Hendrick et al., 2001), while others affect different aspects of translation, from initiation to quality control (Dong et al., 2017; Graifer et al., 2014; Limoncelli et al., 2017; Takyar et al., 2005). The three residues S104, L113 and G121 implicated in reading frame maintenance in our study, as well as K108, are all found in two α-helices near the mRNA entry tunnel of the ribosome; these residues reside on the surface of the ribosome and could interact with mRNA or proteins outside of the ribosome (Figure 2A). Moreover, the identity of all four of these residues is conserved in eukaryotes, but different in bacteria and archaea (Graifer et al., 2014).
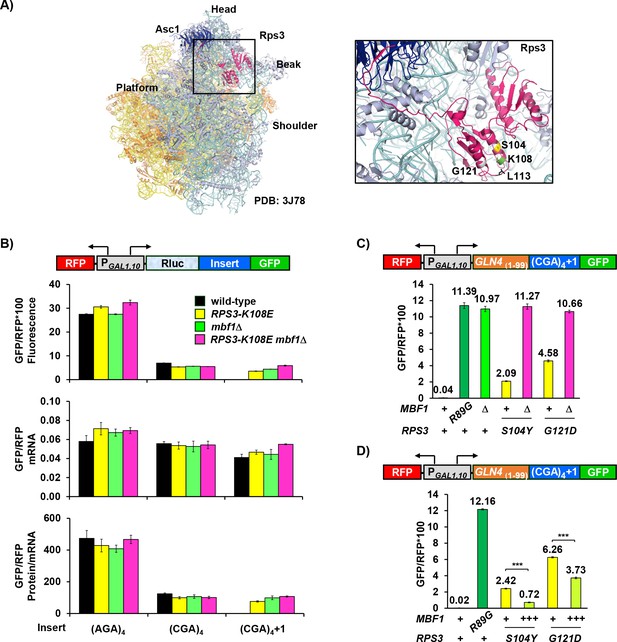
Ribosomal protein Rps3 mediates reading frame maintenance with Mbf1 at CGA codon repeats.
(A) Left: Yeast ribosome from PDB: 3J78 (Svidritskiy et al., 2014) (light blue: small subunit; yellow: large subunit) showing Asc1/RACK1 (dark blue) and Rps3 (pink). Right: Residues of Rps3 in which mutations cause frameshifting are marked- S104 (yellow), K108 (green), L113 (black), G121 (gray). (B) Analysis of effects of RPS3-K108E, mbf1Δ and RPS3-K108E mbf1Δ mutations on expression of GFP/RFP protein (fluorescence), mRNA and protein/mRNA from reporters containing four Arg codons (AGA versus CGA) in-frame and in the +1 frame. (C) Assay for epistatic relationship between RPS3 mutations from this selection and the mbf1Δ mutation. (D) Overproduction of Mbf1 protein in indicated RPS3 mutants significantly decreased expression of frameshifted Gln4(1-99)-GFP fusion protein (***p < 0.001) analyzed by flow cytometry.
-
Figure 2—source data 1
(Source data file for Figure 2B–D).
- https://doi.org/10.7554/eLife.39637.009
We initially examined the effect of the RPS3-K108E mutation on both frameshifting and in-frame expression downstream of CGA codon repeats, and found that this mutation allows frameshifting but does not affect in-frame expression. We chose the K108E mutation because it is known to have only minor effects on the polysome to monosome ratio (Dong et al., 2017), consistent with few nonspecific effects on translation. We introduced modified RNA-ID reporters into rps3Δ::bleR strains in which the only source of RPS3 is a plasmid-borne copy (either wild type or K108E). As described previously, since the expression of GFP and RFP is driven by the bi-directional GAL1,10 promoter, we use the ratio of GFP/RFP to reduce noise and cell type specific differences in induction of this promoter (Dean and Grayhack, 2012). Neither the RPS3 mutant nor a mbf1Δ mutant had a substantial effect on GFP/RFP fluorescence (protein), mRNA or protein/mRNA of reporters with CGA or AGA codon repeats in-frame (Figure 2B). We did note relatively minor, but compensatory effects, of the mutants on both GFP and RFP mRNAs (a 15–30% reduction in mbf1Δ mutants and a similar increase in the RPS3–K108E mutant) (Figure 2—figure supplement 1A). The RPS3-K108E and mbf1Δ mutants each caused substantially increased frameshifted GFP/RFP protein and protein/mRNA in the construct with four CGA codons, but had only small effects on GFP/RFP mRNA; no frameshifting was seen with four AGA codons (Figure 2B; Supplementary file 1).
If Mbf1 and Rps3 proteins function in independent pathways to prevent frameshifting, we expected that RPS3-K108E mbf1Δ double mutants would frameshift more efficiently than either single mutant. Instead, we found that the double mutant RPS3-K108E mbf1Δ exhibited only a slight increase in frameshifted GFP/RFP protein; this increase is likely due to a slight increase in GFP/RFP mRNA relative to either single mutant, resulting in nearly identical protein/mRNA from the mbf1Δ and RPS3-K108E mbf1Δ mutants (Figure 2B, Figure 2—figure supplement 1B). We also examined effects of combining MBF1 mutants with other RPS3 mutants, S104Y and G121D from our selection, to determine epistasis. In these cases again, each single mutant exhibited frameshifting and the double mutants exhibited similar amounts of frameshifted GFP/RFP to that in the mbf1Δ strain, although even an additive effect would be easily detectable (Figure 2C). Thus, we think it is likely that Mbf1 and the two α-helices in the N-terminal Rps3 protein have related roles in reading frame maintenance.
If Mbf1 and these two α-helices in Rps3 mediate a common function, then frameshifting in either RPS3-S104Y or G121D mutants might be suppressed by overproduction of MBF1. We found that introduction of additional copies of the MBF1 gene into either of these mutants resulted in reduced expression of frameshifted GFP (Figure 2D). Frameshifted GFP was reduced to 30% in the S104Y mutant and to 60% in the G121D mutant (Figure 2D). Similarly, growth on media lacking uracil was severely compromised in the RPS3-S104Y mutant when MBF1 was expressed on a multi-copy plasmid, relative to an empty vector control (Figure 2—figure supplement 1C), although both strains grow equally well on SD-Leu media. These observations are consistent with the idea that Mbf1 and Rps3 play similar roles in reading frame maintenance and support the idea that these RPS3 mutations reduce Mbf1 function.
Mbf1 and Asc1 play distinct, but related, roles at CGA codon repeats
Since Asc1 is also required for reading frame maintenance at CGA codon repeats (Wolf and Grayhack, 2015), we examined the relationship between MBF1 and ASC1 on both frameshifting and in-frame expression, comparing GFP/RFP in the single mutants to that in the asc1Δ mbf1Δ double mutant. Asc1 is known to affect expression (both in-frame and +1 frame) downstream of four CGA codons (Letzring et al., 2013; Wolf and Grayhack, 2015), but we previously noted that inhibitory effects of CGA codons are mediated by CGA codon pairs (Gamble et al., 2016; Letzring et al., 2010). Therefore, we compared the effects of these mutants on a set of reporters with three CGA-CGA (or AGA-AGA) codon pairs flanked by two non-Arg codons (Figure 3A, Figure 3—figure supplement 1A, Supplementary file 2) to effects on a set with four adjacent CGA (or AGA) codons (Figure 3—figure supplement 1B). Neither the upstream gene nor the arrangement of CGA codons affected the results.
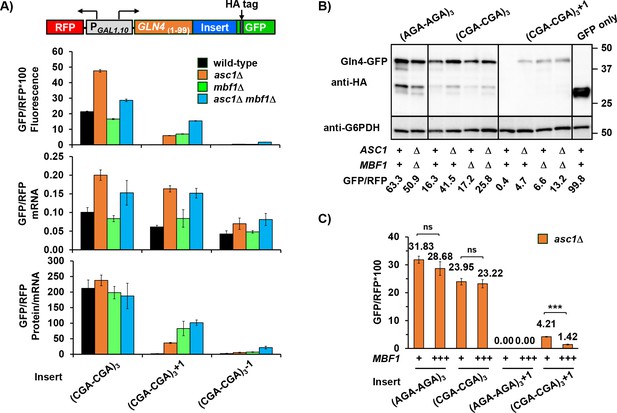
Mbf1 and Asc1 play distinct, but related, roles at CGA codon pairs.
(A) Analysis of effects of asc1Δ, mbf1Δ and asc1Δ mbf1Δ mutations on protein expression (fluorescence), mRNA levels and protein/mRNA of GLN4(1-99)-GFP reporters containing three CGA-CGA codon pairs in the 0, +1, and −1 reading frames. GFP/RFP values are reported in all cases. (B) Western analysis of Gln4(1-99)-GFP fusion protein in yeast strains from (A) indicates the expression of frameshifted Gln4(1-99)-GFP full-length protein in all three mutants. The protein was detected by anti-HA antibody recognizing the HA epitope between the codon insert and GFP. The GFP and RFP values were measured by flow cytometry while harvesting for cell lysis. (C) Overproduction of Mbf1 suppressed frameshifting at CGA-CGA codon pairs in the asc1Δ mutant, but did not affect the in-frame expression, based on GFP/RFP expression from the indicated reporters shown in (A). ns: p > 0.05, ***p < 0.001.
-
Figure 3—source data 1
Source data file for Figure 3A and C).
- https://doi.org/10.7554/eLife.39637.013
As expected based on a previous report (Sitron et al., 2017), deletion of ASC1 resulted in increased protein and mRNA levels of reporters with in-frame CGA-CGA codon pairs. By contrast, deletion of MBF1 did not affect protein or mRNA levels substantially (Figure 3A, Figure 3—figure supplement 1A and B, Supplementary file 2). Thus, Asc1 clearly has a unique role in regulating mRNA and RQC recruitment at CGA codon pairs, but overall expression, measured as protein/mRNA, of all in-frame reporters is similar in the wild type, asc1Δ, mbf1Δ, and asc1Δ mbf1Δ double mutants (Figure 3A). For the in-frame reporters, the relationship between GFP/RFP fluorescence and mRNA is linear; none of these mutants affect RFP mRNA (Figure 3—figure supplement 1C and D). However, the increase in mRNA in an asc1Δ mutant does not explain the increase in frameshifted GFP/RFP protein in this mutant. That is, the 2.7-fold increase in GFP/RFP mRNA from the +1 reporter in an asc1Δ mutant (relative to the wild type) cannot account for the >50 fold increase in frameshifted GFP/RFP fluorescence (Figure 3A). Thus, an asc1Δ mutant clearly exhibits a defect in reading frame maintenance.
If increased frameshifted protein/mRNA in an asc1Δ mutant is due to a failure of the Mbf1 pathway, then we expected that asc1Δ mbf1Δ double mutants would frameshift with similar efficiency to the mbf1Δ mutant. In fact, the level of frameshifted GFP/RFP protein per mRNA was very similar in the single mbf1Δ mutant to that in the double asc1Δ mbf1Δ mutant (Figure 3A, Figure 3—figure supplement 1D, Supplementary file 2). These results are most consistent with a single pathway of reading frame maintenance, which Asc1 influences.
We confirmed that the +1 GFP signal detected in our mutants was due to frameshifting rather than another aberrant translation event by directly measuring both the size and amount of GFP fusion protein. The amount of full-length GFP protein in the Western blot corresponds to the GFP/RFP values obtained from flow analysis (Figure 3B) indicating that +1 GFP/RFP signal in our mutants is due to frameshifting.
If the defect in the asc1Δ mutant that results in frameshifting is due to a failure of the Mbf1 pathway, then overproduction of Mbf1 in the asc1Δ mutant might suppress frameshifting in this mutant. We found that expression of MBF1 on a multi-copy plasmid did suppress frameshifting in the asc1Δ strain to 1/3 that seen with an empty vector, but did not affect in-frame expression (Figure 3C). The overproduction of Mbf1 was not complementing a reduced abundance of Mbf1 in this mutant. We did not detect a reduction in Mbf1-HA (which complements the mbf1Δ mutant) in the asc1Δ strain (Figure 3—figure supplement 2A), although asc1 mutants generally exhibit a defect in expression of small proteins (Thompson et al., 2016). We also considered that mbf1 mutants might require additional Asc1 protein, but additional copies of ASC1 did not suppress frameshifting in an mbf1Δ mutant (Figure 3—figure supplement 2B). Thus, the frameshifted GFP/RFP fluorescence in the asc1Δ strain is likely a result of both an increase in mRNA and a defect in the Mbf1 pathway. We infer that Mbf1 and Asc1 contribute in distinct ways to the response to CGA codon pairs, but we do not know if Asc1 also has a direct role in the reading frame maintenance pathway.
Mbf1 and Asc1 work at a common subset of inhibitory codon pairs and at a single inhibitory codon pair in a context-dependent manner
To address the mechanism of frameshifting and to understand the relationship between Asc1 and Mbf1, we set out to identify the protein and sequence requirements for efficient frameshifting. We began by examining frameshifting at 12 of 17 inhibitory codon pairs all of which cause reduced expression and many of which exhibit high ribosome occupancy, indicative of slow translation (Gamble et al., 2016), a common feature of many frameshifting sites.
We found that Mbf1 and Asc1 act on the same inhibitory codon pairs. Frameshifting occurs with high efficiency at three codon pairs (CGA-CGA, CGA-CGG, and CGA-CCG) in the mbf1Δ mutant (Figure 4A), the only three pairs at which Asc1 substantially modulates in–frame expression levels relative to synonymous optimal reporters (Figure 4B). As might be expected, frameshifted GFP/RFP for CGA-CCG and CGA-CGA is greater in the asc1Δ mbf1Δ mutant than in the mbf1Δ single mutant (Figure 4A, Figure 4—figure supplement 1A, Supplementary file 2). Surprisingly, this is not true for the CGA-CGG construct at which frameshifting is remarkably high (~76% based on data from Figure 4—figure supplement 2C). We address the source of this high frameshifting below and the lack of synergy in the Discussion.
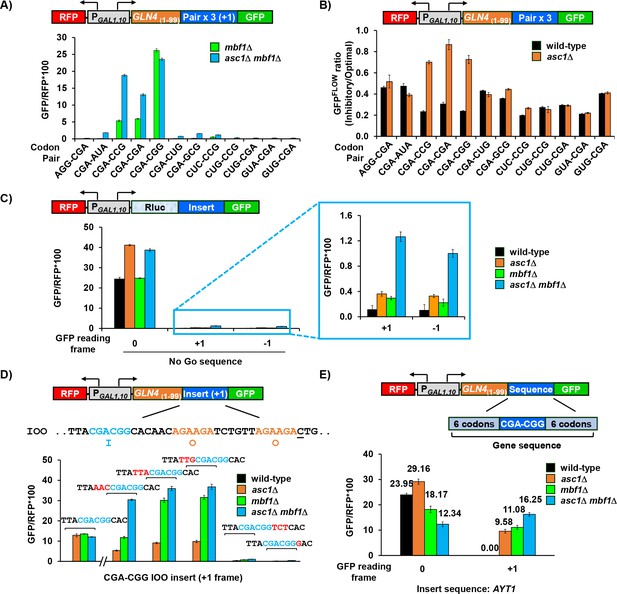
Mbf1 and Asc1 work at a common subset of inhibitory codon pairs and at a single inhibitory codon pair in a context-dependent manner.
(A) Frameshifted GFP/RFP fluorescence was detected at three inhibitory codon pairs (Gamble et al., 2016) in the mbf1Δ mutant, and at seven codon pairs in the asc1Δ mbf1Δ double mutant. Frameshifting was assayed from reporters bearing 3 copies of the indicated inhibitory codon pair and a +1 nucleotide to place GFP in the +1 frame. (B) In-frame expression downstream of three inhibitory codon pairs (CGA-CGA; CGA-CCG; CGA-CGG) was improved by the deletion of ASC1. The GFPFLOW ratio is the ratio of GFP/RFP from reporters with three copies of an inhibitory pair relative to GFP/RFP from synonymous reporters with three copies of the optimized pair. (C) Mutation of either ASC1 or MBF1 allowed frameshifting at no-go sequences in the GFP reporter, and mutation of both ASC1 and MBF1 resulted in significantly more frameshifted GFP/RFP. (D) Variation of the sequences surrounding a single CGA-CGG inhibitory codon pair indicated that the 3’ nucleotide downstream of the pair was required for efficient frameshifting. Frameshifted GFP/RFP from GLN4(1-99)-insert-+1 GFP reporters with a single CGA-CGG inhibitory codon pair was analyzed in the four indicated strains. Variations in the sequences surrounding this pair are shown in red. The construct, IOO contains the inhibitory CGA-CGG pair in position 1(I) and synonymous optimal pairs (AGA-AGA) in the positions 2 and 3 (OO) sites. The original IOO construct was measured separately (hash marks) and also reported in Figure 4—figure supplement 2B. (E) Analysis of effects of asc1Δ, mbf1Δ and asc1Δ mbf1Δ mutations on expression of GLN4(1-99)-GFP reporters containing the native yeast AYT1 sequence with a single CGA-CGG codon pair in 0 and +1 reading frames. This native yeast sequence provoked significant amount of frameshifting.
-
Figure 4—source data 1
Source data file for Figure 4A–E.
- https://doi.org/10.7554/eLife.39637.019
Lower levels of frameshifting were also detected at 4 additional pairs (CGA-AUA, CGA-CUG, CGA-GCG, and CUC-CCG) in the asc1Δ mbf1Δ mutant, but not in the mbf1Δ single mutant (Figure 4A, Figure 4—figure supplement 1B). Analysis of these seven codon pairs indicates that frameshifting was detected at the seven most slowly translated codon pairs in the yeast genome [based on analysis in (Gamble et al., 2016)] and at every inhibitory pair with CGA in the 5’ position, consistent with slow decoding of CGA in the P site (Tunney et al., 2018).
To test the idea that Asc1 and Mbf1 prevent frameshifting at any slowly translated sequence, we measured frameshifting at a sequence which forms a secondary structure to slow down translation and induces no-go mRNA decay (Doma and Parker, 2006; Harigaya and Parker, 2010; Passos et al., 2009). In accordance with this idea, frameshifted GFP/RFP protein from both +1 and −1 constructs was detectable in wild type, greater in each single mutant and even greater in the asc1Δ mbf1Δ mutant (Figure 4C). By contrast, these mutants did not affect frameshifting efficiency at the programmed frameshift site for TY1 (Figure 4—figure supplement 1C) (Belcourt and Farabaugh, 1990). Thus, Mbf1 and Asc1 regulate reading frame maintenance at a translational pause (no-go site), but do not enhance frameshifting at site in which translational slippage is encoded.
To define the source of efficient frameshifting in the CGA-CGG reporter, which has 3 CGA-CGG pairs (Figure 4A), we initially determined that the first CGA-CGG codon pair was responsible for highly efficient frameshifting (Figure 4—figure supplement 2A and B). To define the sequence requirements for efficient frameshifting, we varied the sequences surrounding this single CGA-CGG pair and measured frameshifted GFP/RFP in the various mutants. Either of two changes to the sequence downstream of the CGA-CGG pair (one a point mutation and another a codon insertion) eliminated efficient frameshifting in all three mutant strains (Figure 4D). Furthermore, altering the two nucleotides downstream of the first codon pair in the three codon pair reporter reduced frameshifted GFP/RFP in all three mutants and also restored the synergistic dependence on MBF1 and ASC1 (Figure 4—figure supplement 2C and D). By contrast, none of three upstream changes (to the single CGA-CGG reporter) substantially reduced frameshifting (Figure 4D). Thus, the CGA-CGG-C 7-mer is required for efficient frameshifting.
To find out if frameshifting can occur at single CGA-CGG pairs in other sequence contexts, we tested sequences from seven yeast genes in our reporters, including six codons on either side of CGA-CGG pair. Frameshifted GFP/RFP was detected in asc1Δ mbf1Δ mutants in all cases (Figure 4E, Figure 4—figure supplement 2E). In particular, the two native sequences with CGA-CGG-C resulted in frameshifted GFP/RFP in the single mutants (Figure 4E, Figure 4—figure supplement 2E). Thus, CGA-CGG-C is likely a frameshifting sequence, and contexts that allow frameshifting have not been eliminated from native genes.
+1 frameshifting occurs with the CGA codon in the P site
To understand how frameshifting occurs, we wanted to define the direction and position of the actual frameshift. The high efficiency of frameshifting at the CGA-CGG-CAC sequence provided a useful tool to study frameshifting since there is only a short potential frameshifting sequence (a single inhibitory codon pair). We inserted this sequence with its neighboring codons from the RNA-ID reporter into a construct for purification of the frameshifted polypeptide (Figure 5A). The construct was designed such that the protein could be purified either with an upstream affinity tag (GST) to yield all polypeptides or with a downstream affinity tag (Strep II or the ZZ domain of IgG) to yield only frameshifted polypeptides. Treatment with LysC, which cleaves after lysine was expected to yield a 16 or 17 amino acid peptide for analysis by mass spectrometry, depending upon the mechanism of frameshifting.
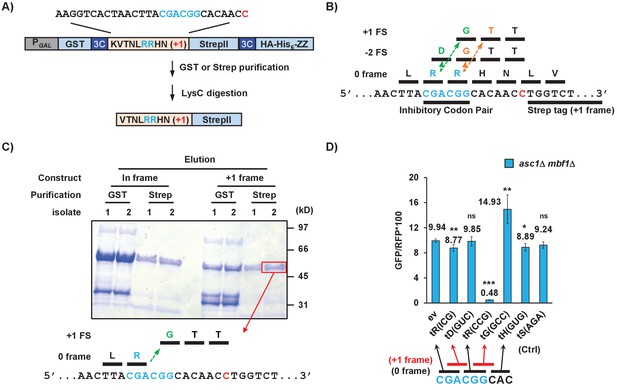
Frameshifting occurs in the +1 direction with the CGA codon in the P site and is modulated by tRNA competition at the A site.
(A) Schematic of the purification construct for the frameshifted peptide. An eight amino acid sequence with a single CGA-CGG pair from the RNA-ID reporter was inserted between a GST tag and an out-of-frame StrepII tag. LysC treatment of purified frameshifted protein yields a 16 or 17 amino acid peptide. The red nucleotide indicates the extra nucleotide in the +1 frame construct. (B) Schematic of four possible frameshifting events at the inhibitory CGA-CGG codon pair, each of which can be distinguished by one or two amino acids in the resulting peptide. Ribosomes can frameshift either in the forward direction (+1) or in the reverse direction (−2) when the P site is occupied by either the CGA codon (first amino acid in the out-of-frame peptide shown in green) or the CGG codon (the first amino acid in the out-of-frame peptide shown in orange). (C) Purified protein products of both in-frame and +1 frame constructs were analyzed by SDS-PAGE, stained with Coomassie Blue. The frameshifted protein of +1 frame construct from Strep purification (in red box) was excised, cleaved with LysC and analyzed by Mass Spectrometry, resulting in identification of the peptide shown below the figure. This peptide corresponds to that expected of a +1 frameshift occurring when the CGA codon occupies the P site. (D) Overexpression of tRNA corresponding to +1 frame codon improved frameshifting efficiency, while overexpression of tRNA corresponding to the next in-frame codon significantly reduced frameshifting. ns: p > 0.05, *p < 0.05, **p < 0.01, ***p < 0.001.
-
Figure 5—source data 1
(Source data file for 5D).
- https://doi.org/10.7554/eLife.39637.023
If frameshifting occurred in the local region near the CGA-CGG codon pair, there are four possible events that could all give rise to +1 GFP signal. Ribosomes could frameshift in the +1 direction with either the CGA or the CGG in the P site, yielding the RGTT or the RRTT sequences shown in Figure 5B. Alternatively, ribosomes could undergo −2 frameshifting at either codon, yielding the peptides RDGTT or RRGTT (Figure 5B). In yeast, −2 frameshifting was observed upon expression of the mammalian antizyme (Matsufuji et al., 1996) and −2 frameshifting also occurs in PRRSV virus (Fang et al., 2012). We purified the frameshifted protein, as well as an in-frame control protein with the sequence expected for a −2 frameshift at CGG (Figure 5C) and subjected them to mass spectrometry. The frameshifted protein yielded the peptide VTNLRGTTWSHPQFEK, the expected peptide from a +1 frameshift beginning with the CGA codon in the P site of the ribosome. Thus, we infer that frameshift occurs with CGA in the P site, yielding only one Arg amino acid on the nascent peptide, then switches to a glycine codon GGC.
To determine if aminoacyl tRNA amounts affect frameshifting, we compared the effects of additional copies of specific Arg and Gly tRNAs on frameshifting in the asc1Δ mbf1Δ double mutant. We found that introduction of additional copies of the gene encoding tRNAArg(CCG), which decoded the in-frame CGG codon, severely reduced frameshifting (Figure 5D), as expected if arg-tRNAArg(CCG) competes with gly-tRNAGly(GCC) for the A site. Similarly, we found that addition of extra copies of tRNAGly(GCC) which decodes +1 frame GGC codon significantly increased frameshifting in our original CGA-CGG-CAC context, as might be expect if the GGC codon is used (Figure 5D). Additional copies of tRNAArg(ICG), tRNAAsp(GUC), tRNAHis(GUG), tRNASer(AGA) had little or no effect, as expected since none of the codons decoded by these tRNAs should be occupying the A site during frameshifting. These results indicate that the frameshifting occurs within the single CGA-CGG-CAC sequence and is modulated by the concentration of aminoacyl tRNAs decoding the out-of-frame codon.
Discussion
We have uncovered a eukaryotic specific system that maintains the reading frame when ribosomes stall. Reading frame maintenance of stalled ribosomes is achieved in two ways: by direct inhibition of frameshifting; and by aborted translation coupled with mRNA decay. The system is composed of two proteins that lack bacterial homologs, the archaeal/eukaryotic Mbf1 protein and the eukaryotic ribosomal protein Asc1/RACK1, as well as one universally conserved ribosomal protein Rps3. Mutations in any of these proteins result in increased frameshifting at CGA codon repeats. Moreover, the Rps3 residues in which mutations affect reading frame maintenance are specifically conserved in eukaryotes (and differ in archaeal and bacterial Rps3), consistent with a eukaryotic-specific mechanism. We suggest that when ribosomes stall (Simms et al., 2017b), two distinct sets of events occur: Asc1 triggers a set of responses that result in aborted translation, recruitment of the RQC complex and mRNA decay (Brandman et al., 2012; Defenouillère et al., 2013; Shao et al., 2013; Simms et al., 2017b; Sitron et al., 2017; Verma et al., 2013); while Mbf1 and Rps3 cooperate at the stalled ribosomes to prevent frameshifting (Figure 6A). In the absence of Mbf1 and Asc1, ribosomes frameshift efficiently, even at a single CGA-CGG pair in some cases, including sequences found in the native yeast genome. Frameshifting on the CGA-CGG codon pair occurs in the +1 direction, with the CGA codon in the P site of the ribosome and is modulated by availability of in-frame and +1 frame A site tRNAs.
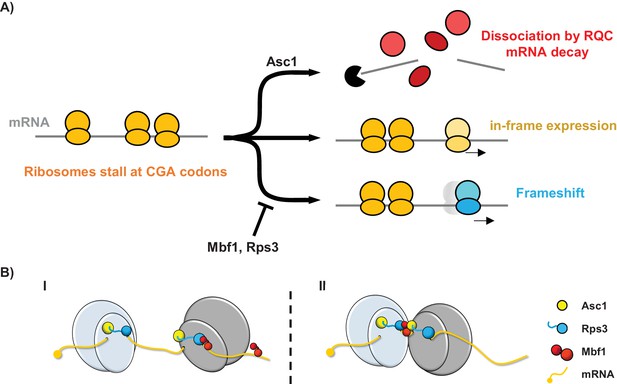
Models for the interplay between the Mbf1/Rps3 and Asc1-mediated RQC pathways and for the role of Mbf1 in reading frame maintenance.
(A) Model of the two pathways that maintain reading frames of stalled ribosomes at CGA codons. Mbf1 and Rps3 act on stalled ribosomes to prevent frameshifting while Asc1 causes removal of many of these ribosome from the translating pool and destroys the mRNA with the stall sequence. (B) Two models of roles of Mbf1 and Rps3 in reading frame maintenance. In model I, Mbf1 (two domains shown in red) interacts with mRNA, and is recruited through an interaction with Rps3 (blue) to the leading stalled ribosome (gray) to restrict mRNA movement. The interaction must be transient, with removal of Mbf1 when the ribosome translocates. In model II, Mbf1 is recruited to the colliding ribosomes (light blue) possibly by both Asc1 (yellow) and Rps3. We postulate that again an interaction with mRNA could buffer the effects of ribosome collision.
The coordinated activities of both the Asc1 and Mbf1/Rps3 pathways are likely important to maintain the reading frame, since the absence of either pathway results in increased frameshifting. We think both pathways are likely engaged by similar stalls, since we noted evidence that Asc1 regulates expression (either in-frame or out-of-frame) at every sequence at which Mbf1 acted. However, there may be differences in the extent to which each pathway contributes at particular stall sites. For instance, at sequences at which frameshifting occurs rapidly (i.e. CGA-CGG-C), Mbf1 may play the critical role; in the absence of Mbf1, ribosomes frameshift before they can be captured by the Asc1 pathway. Generally, some fraction of ribosomes are removed from the translating pool with concomitant mRNA decay, while ribosomes that remain stalled are kept in-frame by Mbf1/Rps3 (Figure 6A).
The Asc1-mediated events couple mRNA decay to abortive translation (Sitron et al., 2017), thus effectively reducing the number and duration of ribosome stalls. The integration of RNA decay with translation is extensive: cleavage of mRNA occurs upstream of many ribosome stalls including at CGA repeats (Chen et al., 2010; Doma and Parker, 2006; Guydosh and Green, 2014; Letzring et al., 2010; Simms et al., 2017b) and is modulated by Asc1 (Ikeuchi and Inada, 2016; Kuroha et al., 2010). mRNA decay is triggered by many problems in translation, such as nonsense mediated decay (NMD), no-go and NonStop decay [See (Shoemaker and Green, 2012; Simms et al., 2017a) and by slow translation (Presnyak et al., 2015; Radhakrishnan et al., 2016). For reading frame maintenance, it seems likely that both reducing the number of stalled ribosomes (by aborting translation) and removing the mRNA are important. Deletion of ASC1 in a mbf1Δ mutant results in an increase in frameshifted GFP/RFP protein that is directly proportional to the increase in mRNA. However, asc1Δ mutants exhibit a 50-fold increase in frameshifting relative to wild type cells with less than a 3-fold increase in mRNA levels. One likely explanation for frameshifting in the asc1Δ mutant is that Mbf1 becomes limiting due to an increase in the number of stalled ribosomes; this idea is strengthened by the observation that overproduction of Mbf1 suppressed frameshifting in the asc1Δ mutant. Alternatively, Asc1 might also play a direct role in the reading frame maintenance function. Asc1 interacts with the C terminal region of Rps3 and could affect the conformation of Rps3 or its interaction with Mbf1. Mbf1 was found in the vicinity of Asc1 (Opitz et al., 2017) lending credence to the idea that Asc1 could interact with Mbf1 (although such an interaction cannot be obligatory).
We think Rps3 and Mbf1 inhibit frameshifting in a coordinated manner, perhaps due to their interactions with mRNA or to Mbf1’s interaction with the ribosome. The role of Rps3 in this process is likely to involve interactions with either the incoming mRNA or proteins external to the ribosome. The RPS3 mutations that affect frameshifting map to residues (S104, L113, G121, K108) on two α-helices or their connecting loop right next to the entering mRNA. Although this section of Rps3 is involved in helicase activity and initiation selectivity (Dong et al., 2017; Takyar et al., 2005), the residues mutated in frameshifting selections were not specifically those involved in these activities. Instead, these residues all sit on the solvent side of the ribosome and could form an interface interacting with mRNA or mRNA-bound proteins. The role of Mbf1 is likely mediated by interactions with either or both of the mRNA (Beckmann et al., 2015; Klass et al., 2013) and the ribosome (Blombach et al., 2014; Opitz et al., 2017). The apparent RNA binding domain maps to the less conserved N terminal domain (Klass et al., 2013), while ribosome binding activity of the archaeal homolog of Mbf1 maps to its C-terminal HTH domain and the linker at the N terminus of this domain, which are both conserved with eukaryotes (Blombach et al., 2014); our frameshifting mutations cluster in the conserved linker region of Mbf1. Moreover, Mbf1 is sufficiently abundant with ~85,000 molecules per cell to participate in general translation cycles, although it is less abundant than core ribosomal proteins (~200,000) (Kulak et al., 2014).
There are two reasonable models to account for the role of Mbf1 and Rps3 in reading frame maintenance (Figure 6B). The first model is that Mbf1 has a loose association with mRNA and is recruited to the leading stalled ribosome by an interaction with Rps3; the interactions with the ribosome and the mRNA at the stall site could restrict mRNA movement in the ribosome. Based on structures of prokaryotic ribosomes caught in translocation, mRNA flexibility may occur in ribosomes lacking an A site tRNA due to few contacts with the region of mRNA near the A site (Zhou et al., 2013), or due to a failure of two rRNA pawls that lock the mRNA in a translocating ribosome (Zhou et al., 2013), or due to defects in the interactions with elongation factor 2 (Zhou et al., 2014). In the absence of Mbf1, the ribosome stall might allow sufficient time for mRNA flexibility, resulting in frameshifting. The second model, which is based on the observation that ribosome collisions trigger no-go decay (Simms et al., 2017b), is that Mbf1 is recruited to colliding ribosomes to buffer the collision effects; in this case Asc1 and Rps3 might both participate in Mbf1 recruitment. Mbf1 could prevent ribosome collision-mediated movement of the leading ribosome on the mRNA.
Frameshifting occurs by a mechanism that involves the interplay between the two adjacent codons, in which I•A wobble interaction in the P site in conjunction with competition between tRNAs entering the A site results in the frameshift, consistent with a model proposed by Baranov et al. (Baranov et al., 2004). Two lines of evidence support this mechanism. First, we demonstrated that, in the asc1Δ mbf1Δ double mutant, ribosomes frameshift at a single CGA-CGG codon pair (in a particular context) when the CGA codon occupies the P site. We infer that CGA codon in the P site is generally important for frameshifting, because six of the seven codon pairs on which ribosomes frameshift are CGA-NNN and the three efficient pairs are CGA-CNN. The wobble interaction between the CGA codon and tRNA could weaken the interaction between mRNA and the ribosome, which in turn could slow down the elongation cycle. Second, we found that frameshifting is influenced by the abundance of the in-frame and out-of-frame tRNAs for next position, which implies that the frameshift occurs after translocation of the CGA from the A site to the P site. We speculate that the flexibility of the wobble base pair interaction between inosine and other nucleotides could actively facilitate the acceptance of out-of-frame A site tRNA. For instance, we consider that a rare instance in which the A base in CGA is bulged out might be stabilized by the very strong I•C interaction, increasing the time available to accept the out-of-frame tRNA.
The eukaryotic specific reading frame maintenance activity, involving Mbf1 and ribosomal proteins Rps3 and Asc1, is likely to be important for translation accuracy in the yeast genome. Mutations in either RPS3 or MBF1 suppressed frameshifting mutations in several native yeast genes (Hendrick et al., 2001). Moreover, mutations in MBF1 and ASC1 resulted in detectable frameshifting in a set of native gene sequences with only a single inhibitory codon pair flanked by six adjacent codons on each side, although it is apparent that the frameshifting potential within a particular sequence is not simply due to the presence of a single inhibitory codon pair. These results confirmed that Mbf1 with Rps3 and Asc1 play a critical role in maintaining the reading frame during normal translation cycles. It is still unknown why this eukaryote-specific reading frame maintenance system evolved and why it is important to eukaryotes, but not bacteria.
Materials and methods
Reagent type | Designation | Source or reference | Identifiers | Additional information |
---|---|---|---|---|
Gene (Saccharomyces cerevisiae) | MBF1/ YOR298C-A | SGD:S000007253 | ||
Gene (S. cerevisiae) | ASC1/ YMR116C | SGD:S000004722 | ||
Gene (S. cerevisiae) | RPS3/ YNL178W | SGD:S000005122 | ||
Strain, strain background (S. cerevisiae, MATa) | BY4741 | Open Biosystems | ||
Strain, strain background (S. cerevisiae, MATα) | BY4742 | Open Biosystems | ||
Genetic reagent (S. cerevisiae) | See Supplementary table 1 in Supplementary file 3 | this paper | ||
Antibody | anti-HA High Affinity (Rat monoclonal) | Roche | 3F10, catalog# 11867423001; RRID:AB_10094468 | 1:3000 dilution |
Antibody | Goat anti-Rat IgG-HRP conjugate | Jackson Immuno Research | catalog# 112-035-003; RRID:AB_2338128 | 1:5000 dilution |
Antibody | anti-G-6-PDH (Rabbit monoclonal) | Sigma | catalog# A9521; RRID: AB_258454 | 1:5000 dilution |
Antibody | Goat anti- Rabbit IgG- HRP conjugate | Biorad | catalog# 1706515; RRID:AB_11125142 | 1:10000 dilution |
Recombinant DNA reagent | See Supplementary table 2 in Supplementary file 3 | this paper | ||
Sequence- based reagent | See Supplementary table 3 in Supplementary file 3 | this paper | ||
Sequence -based reagent | Random Primers | Invitrogen | catalog# 48190011 | |
Commercial assay or kit | T4 DNA Polymerase, LIC-qualified | Novagen | catalog# 70099 | Used for ligation- independent cloning |
Commercial assay or kit | Super Script II Reverse Transcriptase | Invitrogen | catalog# 18064014 | |
Commercial assay or kit | RQ1 Rnase- Free Dnase | Promega | catalog# M6101 | |
Commercial assay or kit | RiboMAX Large Scale RNA Production System-T7 | Promega | catalog# P1300 | |
Commercial assay or kit | MicroSpin G-25 columns | GE | catalog# 27-5325-01 | |
Commercial assay or kit | Fast SYBR Green Master Mix | Applied Biosystems | catalog# 4385612 | |
Commercial assay or kit | Glutathione sepharose-4B | GE | catalog# 17-0756-01 | |
Commercial assay or kit | MagStrep ‘type3’ XT beads | IBA | catalog# 2-4090-002 | |
Chemical compound, drug | 5-FOA | USBiological | catalog# F5050 | |
Chemical compound, drug | Complete mini EDTA-free protease inhibitor | Roche | catalog# 11836170001 | |
Chemical compound, drug | leupeptin | Roche | catalog# 11017128001 | |
Chemical compound, drug | pepstatin | Roche | catalog# 11359053001 | |
Chemical compound, drug | Glutathione | Sigma | catalog# G4251 | |
Chemical compound, drug | Biotin | IBA | catalog# 2-1016-002 | |
Software, algorithm | MultAlin | (Corpet, 1988) | http://multalin.toulouse.inra.fr/multalin/ |
Strains, plasmids, and oligonucleotides
Request a detailed protocolStrains, plasmids, and oligonucleotides used in these studies are listed in Supplementary file 3, Tables 1-3. Parents for all yeast strains described in this study were either BY4741 (MATa his3Δ1 leu2Δ0 met15Δ0 ura3Δ0) or BY4742 (MATαhis3Δ1 leu2Δ0 lys2Δ0 ura3Δ0) (Open Biosystems). The GLN4(1-99)-(CGA)6+1-URA3 reporter used in the selection was constructed with PCR-amplified DNAs (using oligonucleotides OJYW085, 086, 041, 089, 095 and 099), assembled by Ligation Independent Cloning (LIC) methods (Alexandrov et al., 2004; Aslanidis and de Jong, 1990) and then integrated into the CAN1/YEL063C locus on the chromosome V, selecting for canavanine-resistance; constructs were checked by sequencing of genomic PCR fragments. RNA-ID reporters were constructed as described previously and integrated at the ADE2 locus, using selection with MET15 marker in MATa strains or S.pombe HIS5 marker in MATα strains (Dean and Grayhack, 2012; Gamble et al., 2016; Wolf and Grayhack, 2015).
Yeast strains bearing MBF1 deletions were constructed by amplification of the kanR cassette in the yeast strain from the corresponding knockout strain in the systematic deletion collection (Open Biosystems) (Giaever et al., 2002). The MATa yeast strain bearing a deletion of RPS3 was constructed by amplification of bleR cassette (Gueldener et al., 2002) (oligos OW443 and OW445) and integration of this DNA into a strain bearing an URA3 [RPS3] covering plasmid (pEAW433). Yeast strains bearing deletions of ASC1 marked with the S. pombe HIS5 marker (AW768), which have been described previously (Wolf and Grayhack, 2015), were constructed and maintained in the presence of a plasmid born copy of ASC1 on a 2µ, URA3 plasmid. To obtain the asc1Δ strain from the selection parent strain, the ASC1 gene was deleted by a bleR cassette obtained by PCR amplification with oligos OW125 and OW126.
Plasmids bearing the MBF1 gene were constructed by amplification of chromosomal MBF1 gene from −580 in 5’ UTR to +300 in 3’ UTR with oligos OJYW124 and OJYW125, followed by cloning into the 2µ, LEU2 vector (pAVA0577) and into the CEN, LEU2 vector (pAVA0581) to create pEJYW203 and pEJYW176 respectively. The chromosomal HA-tagged MBF1 was constructed by PCR amplification of HA-kanR sequence from pYM45 (Euroscarf) (Janke et al., 2004) with oligos OJYW130 and OJYW132, bearing homology to MBF1, followed by integration into the MBF1 locus. This MBF1-HA KanR cassette from −580 in 5’UTR to +300 in 3’UTR of MBF1 (+1992 including KanR sequences) was amplified from the chromosome with oligos OJYW157 and OJYW158, cloned into the XmaI and NheI sites in Bluescript as pEJYW279. The mbf1 point mutations K64E and I85T were individually introduced into the plasmid pEJYW279 to make pEJYW302 and pEJYW307 respectively. The mbf1-K64E cassette was directly PCR-amplified from the mutant strain YJYW290-P38 with oligos OJYW157 and OJYW158 followed by digestion with XmaI and BamHI and integration into these two sites on pEJYW279. The mbf1-I85T mutation was introduced by PCR amplification from MBF1-HA cassette with OJYW170, which contains the mutation, and OJYW166, followed by integration into pEJYW279 between BamHI and AatII sites. Reconstructed mbf1 point mutants were introduced into YJYW2566 (BY4741, HIS3+) with XmaI/NheI digested pEJYW302 and pEJYW307 selecting with KanR marker. The plasmid template for in vitro transcription of GFP and RFP fragments (pEJYW407 and pEJYW409) was constructed by PCR-amplifying pEAW315 with oligos OJYW295/OJYW296 (for GFP) or OJYW297/OJYW299 (for RFP) followed by digestion with SphI and XmaI and integration into these two sites on pSP73 (Promega, cat.# P2221). Plasmids expressing tRNAs were obtained from Phizicky and Grayhack lab stocks (Guy et al., 2012; Han et al., 2015; Letzring et al., 2010).
Selection for frameshifting mutants and identification of mutations
Request a detailed protocolUra+ mutants were selected from 40 independent cultures of each MATa and MATα parent strains (YJYW289, YJYW329), and then were analyzed by flow cytometry to measure GFP and RFP expression. Ura+ GFP+ mutants, indicative of increased frameshifting efficiency, were selected for further study, with an emphasis on mutants that exhibited higher levels of frameshifting, that is GFP/RFP >4, (28% MATα and 66% MATa mutants). Diploids between 12 MATa mutant and 20 MATα mutants were created by mating in YPD for 2 hr at 25°C and selection on SD-Lys-Leu-His media for diploid cells, followed by streaking for single colonies. Then overnights of the resultant diploids and their haploid parents were spotted on SD-Leu and SD-Leu-Ura plates, which were grown at 30°C.
To identify the relevant mutation in YJYW290-P25, we obtained the Leu- derivative of this mutant (YJYW315) by screening replica plated single colonies from an overnight in YPD on YPD and SD-Leu plates. The Ura+/FOA-sensitive phenotype of this mutant was complemented with a genomic tiled library (Jones et al., 2008), selecting for FOA-resistant cells. First, 17 pools of DNA, each of which contained 96 plasmids (Jones et al., 2008), were transformed individually with >1000 colonies per plate. Transformants of each pool were then scraped and saved in 2 ml YPD +8% DMSO. These saves were plated based on their OD600 (2 × 107 cells/OD600 x ml) to obtain approximately 5,000 cells on SD-Leu and 50,000 cells on SD-Leu +0.5 xFOA. For 16 of 17 pools, there were no colonies on the FOA plates, while transformants of pool 15 had 330 FOA-resistant colonies with 1404 colonies on SD–Leu plate, corresponding to FOA-resistance for 2.3% cells. The plasmids responsible for FOA-resistance was identified by complementing with plasmids from individual rows and columns in this pool as described above, followed by complementation with individual plasmids. Two plasmids from this pool conferred FOA-resistance and share a single gene, MBF1. The MBF1 gene in 19 recessive mutants was amplified from their genomic DNA with oligos OJYW124 and OJYW125, followed by sequencing to confirm the mutated residues.
Whole genome sequencing on two dominant MATα mutants was performed to identify the mutated genes. For each strain,~30 OD600 yeast cells were harvested and re-suspended in 1 ml prep buffer (2% Triton X-100, 1% SDS, 100 mM NaCl, 10 mM Tris-Cl pH 8.0, 1 mM EDTA) with ~1.5 g Zirconia/Silica beads (from BioSpec, catalog# 11079105z) and 1 ml PCA pH 8.0. The suspension was then vortexed at top speed for 3 min and mixed with 1 ml TE pH 8.0, followed by centrifugation in prespun PLG tubes (from 5prime, catalog# 2302830). Nucleic acids in the aqueous layer were ethanol precipitated with 5 ml 100% ethanol, followed by freezing on dry ice and centrifugation for 20 min at 4,000 rpm at 4°C. The pellet was re-suspended in 200 µl TE and incubated at room temperature for 1 hr with 0.2 µg/µl RNaseA to remove RNA contamination, followed by addition of 200 µl 1 M Tris-Cl pH 8.0, 2 µl of 5 mg/ml glycogen and 400 µl PCA, and centrifugation for 2 min at top speed at 4°C. The aqueous layer (~360 µl) was precipitated with 720 µl 100% ethanol and frozen on dry ice for 15 min; resulting pellets were re-suspended in 100 µl TE pH 8.0 and 100 µl 1 M Tris-Cl pH 8.0, followed by precipitation again with 400 µl 100% ethanol. The DNA pellet was then washed with 500 µl 70% ethanol and finally re-suspended in 50 µl sterile ddH2O. Whole genome sequencing was performed by the UR Genomics Research Center resulting in RPS3 mutations in these two MATα mutants. Mutations in two MATa dominant mutants were then identified by amplification of RPS3 cassette with oligos OJYW159 and OJYW210, followed by sequencing.
Analysis of yeast growth
Request a detailed protocolAppropriate control strains (previously studied) and 2–4 independent isolates of each strain being tested were grown overnight at 30°C in media indicated, diluted to obtain OD600 of 0.5, then serially diluted 10-fold twice; 2 µl diluted cells were then spotted onto the indicated plates and grown at different temperatures for at least two days.
Flow cytometry
Request a detailed protocolTo examine mutants in either RPS3 or ASC1, reporters were introduced into sets of strains bearing an URA3 covering plasmid with either RPS3 or ASC1, depending upon the chromosomal deletion. All sets of strains in a given panel contained the same URA3 plasmid. Prior to analysis of GFP expression, strains were streaked on FOA containing plates, then single colonies were grown for analysis by flow cytometry.
Yeast strains bearing the modified RNA-ID reporters were grown overnight at 30°C in YP media (for strains without plasmid) or appropriate synthetic drop-out media (for strains with plasmid) containing 2% raffinose + 2% galactose + 80 mg/L Ade. The cell culture was diluted in the morning such that to the culture had a final OD600 between 0.8–1.0. Analytical flow cytometry and downstream analysis were performed for four independent isolates of each strain (Outliers were rejected using a Q test with >90% confidence level) as previously described (Dean and Grayhack, 2012). Each flow experiment was also performed with proper controls including a GFP-, RFP+ strain. The GFP/RFP value from this control strain was subtracted from all tested strains on the same day to show signals above background (negative values are set to 0). P values were calculated using a one-tailed or two-tailed homoscedastic t test in Excel, as indicated in the source data for relevant figures.
Western blotting
Request a detailed protocolWestern analysis of the GFP fusion proteins in the modified RNA-ID reporter and Mbf1 protein in yeast strains were performed with anti-HA antibody as described previously (Gelperin et al., 2005).
RT-qPCR
Request a detailed protocolmRNA measurements with reverse transcription (RT) reaction and quantitative PCR were performed as described previously (Gamble et al., 2016) with one significant difference. Quantification of mRNA was performed using in vitro transcribed GFP and RFP mRNA fragments, synthesized from linearized plasmid pEJYW407 and pEJYW409 using RiboMAX Large Scale RNA Production System-T7 (Promega, cat.# P1300). The synthesis reaction was followed by DNase treatment to remove the DNA template and by elution through MicroSpin G-25 columns (GE, cat.# 27-5325-01) to remove unincorporated nucleotides. The synthesized RNA sample was analyzed by ultraviolet light absorbance at 260 nm on a nanodrop to determine the concentration and by electrophoresis to assess integrity. Each qPCR plate contained 5-point 1:5 dilution standard curves for both GFP and RFP, which were optimized to ensure that all samples fall into the linear range of the curves. For each tested strain, three biological replicates were analyzed.
Purification of frameshifted peptide
Request a detailed protocolTo purify the frameshifted peptide from yeast, a LEU2 plasmid containing either in-frame or +1 frame protein purification constructs were transformed into the asc1Δ mbf1Δ strain (YJYW378). Two independent transformants (FOA treated) of each construct were grown overnight in SD-Leu media and transferred into 80 ml S-Leu + 2% raffinose media in the morning. After reaching an OD600 of 0.8–1.2, expression of the GST-StrepII-ZZ construct was induced by addition of 40 ml 3xYP + 6% galactose and growth was continued for 10 hr. Cells were collected by centrifugation and cell pellets were quick frozen on dry ice. The cell pellets were re-suspended in 1 ml extraction buffer (50 mM Tris-Cl pH 7.5, 1 mM EDTA, 4 mM MgCl2, 5 mM DTT, 10% Glycerol, 1 M NaCl, 2.5 µg/ml leupeptin, 2.5 µg/ml pepstatin) and lysed with bead beating (10 repeats of 20 s beating followed by 1 min on ice), essentially as described previously (Quartley et al., 2009). The cell lysate was collected from the bead beating tubes by puncturing the bottom with a hot needle and blowing with low pressure air. Solid contents were removed by centrifugation before the remaining lysate was divided into half and purified on either GSH or Streptactin resin.
For GST purification: the cell lysate was first diluted with equal volume No Salt Wash Buffer (50 mM Tris-Cl pH 7.5, 4 mM MgCl2, 5 mM DTT, 10% Glycerol) to bring the salt to 0.5 M NaCl. GSH resin [Glutathione sepharose-4B from GE, catalog# 17-0756-01; pre-washed with Wash Buffer (No Salt Wash Buffer + 0.5 M NaCl)] (50 µl/ ml of lysate) was added to the diluted cell lysate and the mixture was nutated for 3 hr at 4°C. The resin was separated from the liquid by centrifugation at low speed (<3,000 rpm) and washed twice with 0.5 ml Wash Buffer followed by 20 min nutation. The bound protein products were then eluted by nutating for 40 min with 100 µl Elution Buffer (Wash buffer + 20 mM NaOH + 25 mM glutathione); the elution step was repeated to increase the yield.
For Strep purification: the cell lysate was diluted with 5x volumes No Salt Wash Buffer (100 mM Tris-Cl pH 7.5, 1 mM EDTA, 2.5 µg/ml leupeptin, 2.5 µg/ml pepstatin) to bring the salt to 150 mM NaCl. MagStrep ‘type3’ XT beads [from IBA, cat# 2-4090-002; pre-washed with Wash Buffer (No Salt Wash Buffer + 150 mM NaCl)] were added to the diluted cell lysate (80 µl/ 3.3 ml diluted cell lysate). After nutating for 2 hr at 4°C, resins were separated from liquid using a magnetic separator, then the resin was washed with 1 ml Wash Buffer three times without additional incubation. The bound protein products were then eluted by adding 50 µl Elution Buffer (Wash buffer + 50 mM biotin) and nutating for 10 min, followed by separation using magnetic separator; the elution step was repeated to increase the yield.
Mass spectrometry
Request a detailed protocolThe elution samples from both GST and Strep purification were analyzed by SDS-PAGE, followed by staining with Coomassie Blue. The bands from Strep purification of both in-frame and +1 frame constructs were excised and analyzed on the Q Exactive Plus Mass Spectrometer in the Mass Spectrometry Resource Center of the University of Rochester Medical Center.
Data availability
All data generated or analyzed during this study are included in the manuscript and supporting files.
References
-
A facile method for high-throughput co-expression of protein pairsMolecular & Cellular Proteomics 3:934–938.https://doi.org/10.1074/mcp.T400008-MCP200
-
Ligation-independent cloning of PCR products (LIC-PCR)Nucleic Acids Research 18:6069–6074.https://doi.org/10.1093/nar/18.20.6069
-
A gripping tale of ribosomal frameshifting: extragenic suppressors of frameshift mutations spotlight P-site realignmentMicrobiology and Molecular Biology Reviews 73:178–210.https://doi.org/10.1128/MMBR.00010-08
-
The RNA-binding proteomes from yeast to man harbour conserved enigmRBPsNature Communications 6:10127.https://doi.org/10.1038/ncomms10127
-
Choreography of molecular movements during ribosome progression along mRNANature Structural & Molecular Biology 23:342–348.https://doi.org/10.1038/nsmb.3193
-
Archaeal MBF1 binds to 30S and 70S ribosomes via its helix-turn-helix domainBiochemical Journal 462:373–384.https://doi.org/10.1042/BJ20131474
-
Ribosome-associated protein quality controlNature Structural & Molecular Biology 23:7–15.https://doi.org/10.1038/nsmb.3147
-
Structure of the Dom34-Hbs1 complex and implications for no-go decayNature Structural & Molecular Biology 17:1233–1240.https://doi.org/10.1038/nsmb.1922
-
Multiple sequence alignment with hierarchical clusteringNucleic Acids Research 16:10881–10890.https://doi.org/10.1093/nar/16.22.10881
-
Translation elongation and recoding in eukaryotesCold Spring Harbor Perspectives in Biology 10:a032649.https://doi.org/10.1101/cshperspect.a032649
-
Mechanisms and implications of programmed translational frameshiftingWiley Interdisciplinary Reviews: RNA 3:661–673.https://doi.org/10.1002/wrna.1126
-
Evolution of +1 programmed frameshifting signals and frameshift-regulating tRNAs in the order SaccharomycetalesJournal of Molecular Evolution 63:545–561.https://doi.org/10.1007/s00239-005-0311-0
-
Biochemical and genetic analysis of the yeast proteome with a movable ORF collectionGenes & Development 19:2816–2826.https://doi.org/10.1101/gad.1362105
-
No-go decay: a quality control mechanism for RNA in translationWiley Interdisciplinary Reviews: RNA 1:132–141.https://doi.org/10.1002/wrna.17
-
Yeast frameshift suppressor mutations in the genes coding for transcription factor Mbf1p and ribosomal protein S3: evidence for autoregulation of S3 synthesisGenetics 157:1141–1158.
-
Mode of action of the herbicide, 3-amino-1,2,4-triazole(amitrole): inhibition of an enzyme of histidine biosynthesisArchives of Biochemistry and Biophysics 112:544–547.https://doi.org/10.1016/0003-9861(65)90093-7
-
Structural aspects of messenger RNA reading frame maintenance by the ribosomeNature Structural & Molecular Biology 17:555–560.https://doi.org/10.1038/nsmb.1790
-
Ribosomal stalling during translation: providing substrates for ribosome-associated protein quality controlAnnual review of Cell and Developmental Biology 33:343–368.https://doi.org/10.1146/annurev-cellbio-111315-125249
-
Mediators of activation of fushi tarazu gene transcription by BmFTZ-F1Molecular and Cellular Biology 14:3013–3021.https://doi.org/10.1128/MCB.14.5.3013
-
Reading two bases twice: mammalian antizyme frameshifting in yeastThe EMBO Journal 15:1360–1370.https://doi.org/10.1002/j.1460-2075.1996.tb00478.x
-
The ribosome moves: RNA mechanics and translocationNature Structural & Molecular Biology 24:1021–1027.https://doi.org/10.1038/nsmb.3505
-
Analysis of Dom34 and its function in no-go decayMolecular Biology of the Cell 20:3025–3032.https://doi.org/10.1091/mbc.e09-01-0028
-
Heterologous expression of L. major proteins in S. cerevisiae: a test of solubility, purity, and gene recodingJournal of Structural and Functional Genomics 10:233–247.https://doi.org/10.1007/s10969-009-9068-9
-
A flexible loop in yeast ribosomal protein L11 coordinates P-site tRNA bindingNucleic Acids Research 38:8377–8389.https://doi.org/10.1093/nar/gkq711
-
Translation in prokaryotesCold Spring Harbor Perspectives in Biology 10:a032664.https://doi.org/10.1101/cshperspect.a032664
-
Mutations in elongation factor EF-1 alpha affect the frequency of frameshifting and amino acid misincorporation in Saccharomyces cerevisiaeGenetics 120:923–934.
-
Regulation of tryptophan biosynthesis in Saccharomyces cerevisiae: mode of action of 5-methyl-tryptophan and 5-methyl-tryptophan-sensitive mutantsJournal of Bacteriology 117:1131–1140.
-
Alternative fates of paused ribosomes during translation terminationJournal of Biological Chemistry 286:31105–31112.https://doi.org/10.1074/jbc.M111.268201
-
Translation drives mRNA quality controlNature Structural & Molecular Biology 19:594–601.https://doi.org/10.1038/nsmb.2301
-
Ribosome-based quality control of mRNA and nascent peptidesWiley Interdisciplinary Reviews: RNA 8:e1366.https://doi.org/10.1002/wrna.1366
-
Saturation mutagenesis of 5S rRNA in Saccharomyces cerevisiaeMolecular and Cellular Biology 21:8264–8275.https://doi.org/10.1128/MCB.21.24.8264-8275.2001
-
Yeast coactivator MBF1 mediates GCN4-dependent transcriptional activationMolecular and Cellular Biology 18:4971–4976.https://doi.org/10.1128/MCB.18.9.4971
-
The role of wobble uridine modifications in +1 translational frameshifting in eukaryotesNucleic Acids Research 43:9489–9499.https://doi.org/10.1093/nar/gkv832
-
Accurate design of translational output by a neural network model of ribosome distributionNature Structural & Molecular Biology 25:577–582.https://doi.org/10.1038/s41594-018-0080-2
-
Role of a tRNA base modification and its precursors in frameshifting in eukaryotesJournal of Biological Chemistry 282:26026–26034.https://doi.org/10.1074/jbc.M703391200
-
SUF12 suppressor protein of yeast. A fusion protein related to the EF-1 family of elongation factorsJournal of Molecular Biology 199:559–573.https://doi.org/10.1016/0022-2836(88)90301-4
Article and author information
Author details
Funding
National Institutes of Health (R01 GM118386)
- Elizabeth J Grayhack
The funders had no role in study design, data collection and interpretation, or the decision to submit the work for publication.
Acknowledgements
We thank Eric Phizicky, Christina Brule, Andrew Wolf and Lu Han for discussions of the science and comments on the manuscript; Christina Brule and Blake Bentley for assistance with experiments, Wendy Gilbert and Mary Thompson for assistance with ribosome profiling data. This research has been facilitated by the services and resources provided by the University of Rochester Mass Spectrometry Resource Laboratory and NIH instrument grant (1S10OD021486-01). We thank the University of Rochester Genomics Research Center for performing high-throughput sequencing library construction, sequencing, and primary data analysis for this study. We also thank the URMC Flow Cytometry Resource for technical support. This work was supported by NIH grant R01 GM118386 to EJG.
Copyright
© 2018, Wang et al.
This article is distributed under the terms of the Creative Commons Attribution License, which permits unrestricted use and redistribution provided that the original author and source are credited.
Metrics
-
- 3,080
- views
-
- 522
- downloads
-
- 44
- citations
Views, downloads and citations are aggregated across all versions of this paper published by eLife.
Citations by DOI
-
- 44
- citations for umbrella DOI https://doi.org/10.7554/eLife.39637