Dynamic change of electrostatic field in TMEM16F permeation pathway shifts its ion selectivity
Abstract
TMEM16F is activated by elevated intracellular Ca2+, and functions as a small-conductance ion channel and as a phospholipid scramblase. In contrast to its paralogs, the TMEM16A/B calcium-activated chloride channels, mouse TMEM16F has been reported as a cation-, anion-, or non-selective ion channel, without a definite conclusion. Starting with the Q559K mutant that shows no current rundown and less outward rectification in excised patch, we found that the channel shifted its ion selectivity in response to the change of intracellular Ca2+ concentration, with an increased permeability ratio of Cl- to Na+ (PCl-/PNa+) at a higher Ca2+ level. The gradual shift of relative ion permeability did not correlate with the channel activation state. Instead, it was indicative of an alteration of electrostatic field in the permeation pathway. The dynamic change of ion selectivity suggests a charge-screening mechanism for TMEM16F ion conduction, and it provides hints to further studies of TMEM16F physiological functions.
https://doi.org/10.7554/eLife.45187.001Introduction
Mammalian TMEM16F (Anoctamin-6, ANO6) is a membrane protein with dual functions of phospholipid scrambling and ion conduction, both activated by elevation of intracellular Ca2+ (Whitlock and Hartzell, 2017; Falzone et al., 2018; Bevers and Williamson, 2016; Pedemonte and Galietta, 2014). When activated, TMEM16F mediates the exposure of phosphatidylserine, a lipid normally restricted to the inner leaflet of cell membrane lipid bilayer, to the cell surface (Suzuki et al., 2010). This process, known as lipid scrambling, initiates many physiological processes such as recruitment of tissue factors to the platelet surface for thrombin production to trigger blood coagulation and modulation of immune responses in T lymphocytes (Suzuki et al., 2010; Yang et al., 2012; Hu et al., 2016; Zaitseva et al., 2017). TMEM16F is also a Ca2+-activated small-conductance ion channel (Yang et al., 2012), raising the possibility that it has additional physiological functions that have not been revealed. However, there is discrepancy regarding TMEM16F ion selectivity reported by different labs. Recorded with excised-patch inside-out configuration, TMEM16F channels are quickly activated by micromolar Ca2+ and they are more permeable to cations (mainly physiological cations such as Na+, K+ and Ca2+) than to Cl- (Yang et al., 2012; Alvadia et al., 2019). Surprisingly, many groups have reported that the TMEM16F whole-cell current is activated several minutes after cytoplasmic Ca2+ elevation, and it displays less cation-selectivity (Yu et al., 2015) or even higher permeability to Cl- than to Na+ (Grubb et al., 2013; Scudieri et al., 2015; Shimizu et al., 2013; Tian et al., 2012). This disagreement hampers our further understanding of the functions of this membrane protein.
TMEM16F belongs to the mammalian TMEM16 membrane protein family with 10 members (Whitlock and Hartzell, 2017; Falzone et al., 2018; Pedemonte and Galietta, 2014). The founding members TMEM16A (ANO1) and TMEM16B (ANO2) represent the only two canonical Ca2+-activated Cl- channels (CaCC) without lipid scrambling functions (Schroeder et al., 2008; Caputo et al., 2008; Yang et al., 2008; Stephan et al., 2009), while several other mammalian homologs show a modest selectivity between cations and anions but with lipid scrambling capacities (Suzuki et al., 2010; Suzuki et al., 2013; Gyobu et al., 2017; Le et al., 2019a; Watanabe et al., 2018), a property closer to that of their fungal homologues (Malvezzi et al., 2013; Brunner et al., 2014; Lee et al., 2016). Mammalian TMEM16 proteins are dimeric, as revealed by structural analyses of TMEM16A, TMEM16F and TMEM16K via electron cryo-microscopy (cryo-EM) or crystallography (Alvadia et al., 2019; Paulino et al., 2017a; Paulino et al., 2017b; Bushell, 2018; Dang et al., 2017; Feng et al., 2019), and each subunit contains a permeation pathway that works independently (Paulino et al., 2017b; Lim et al., 2016; Jeng et al., 2016). For TMEM16A, each subunit contains 10 transmembrane helices (TM1 ~TM10), and the stabilization of TM6 by the binding of Ca2+ ions to the binding-pocket formed by acidic residues on TM6, TM7 and TM8 within the transmembrane domain opens the permeation pathway, composed of TM3-TM8 (Paulino et al., 2017a; Peters et al., 2018). Recent studies suggested that, in addition to the pore-lining residues on TM3-TM8 along the TMEM16A permeation pathway for anion conduction, the positive electrostatic field introduced by the Ca2+ ions bound to the binding-pocket also contributes to anion accessibility to the pore (Lam and Dutzler, 2018). This finding raised the possibility that TMEM16 proteins might adopt a strategy of utilizing the electrostatic field to control ion accessibility to the pore, a process that allows these proteins to be modulated by their surroundings such as Ca2+ level. We hypothesize that TMEM16F shifts its ion selectivity in response to elevation of intracellular Ca2+ concentrations. However, because TMEM16F current in inside-out excised patch exhibits rapid desensitization and rundown in high Ca2+ (Ye et al., 2018), it is challenging to test for TMEM16F ion permeability ratio under a wide range of Ca2+ concentrations.
In TMEM16F, glutamine 559 (Q559) faces the ionic permeation pathway, and lysine substitution of this pore-lining residue (Q559K) reduces the ratio of Na+ permeability to Cl- permeability (PNa+/PCl-) (Yang et al., 2012; Alvadia et al., 2019; Feng et al., 2019). Previous studies also show that the current of Q559K persists with prolonged exposure of the excised patch to high intracellular Ca2+, and that it displays reduced outward rectification (Alvadia et al., 2019; Nguyen et al., 2019). In this study, we found that this mutant has different ratio of Na+ permeability to Cl- permeability (PNa+/PCl-) in different Ca2+ concentrations. The shift of permeability ratio does not correlate with alterations of channel open states, but instead is regulated by the change of electrostatic field along the permeation pathway, on which divalent cations such as Ca2+ and Zn2+ have more significant impact than monovalent ions. Depolarization, which facilitates intracellular cation entry into the membrane electric field, promotes the shift of the relative permeability toward a preference for Cl- over cations. Such an electrostatic modulation could reflect a general feature of the mechanism of ionic transportation by TMEM16 proteins, and it suggests that TMEM16F harbors an inherent machinery that allows it to dynamically modulate preference between cations and anions in response to its local environment.
Results
TMEM16F Q559K increases permeability to Cl- as intracellular Ca2+ increases
Recording from inside-out membrane patch held at +80 mV revealed that wild-type mouse TMEM16F current was activated by intracellular Ca2+ in a dose-dependent manner. The current started to decrease when Ca2+ was higher than ~30 µM, as a result of both desensitization and rundown (Figure 1A,C,D) (Ye et al., 2018). Here, desensitization refers to a decreased sensitivity to Ca2+, caused by degradation of PIP2 via membrane-tethered phospholipase activated by high intracellular Ca2+. Desensitization is also reflected by the requirement of stronger depolarization for channel activation, as a result of the synergy between depolarization and Ca2+ level (to be shown later). Rundown refers to a reduction of current magnitude (induced by 1 mM Ca2+ in this case). To measure the voltage dependence of activation, we recorded with a voltage-family protocol from −40 mV to +160 mV with 10 mV increments followed by holding at 100 mV to obtain ‘tail-currents’, which we used as indicators of steady-state conductance at the range of voltages. Fitting to the sigmoidal conductance-voltage (G-V) relationship yielded V1/2 of 76 ± 11 mV in 15 µM Ca2+ for wild-type TMEM16F, while in 1 mM Ca2+, desensitization strongly reduced the voltage-gating, as was previously reported (Figure 1—figure supplement 1A,B,C) (Ye et al., 2018). The combination of rundown and desensitization rendered it difficult to record the reversal potential (Erev) of wild-type TMEM16F in 1 mM Ca2+. In our experiments, we measured the Erev from the current recorded with a hyperpolarizing ramp (from +80 mV to −80 mV, 1 V/s) following holding the excised patch at +80 mV. When we switched the bath (equivalent to intracellular solution) from 150 mM NaCl to 45 mM or 15 mM NaCl (osmolarity balanced with mannitol), the current recorded from Tmem16f-transfected cells immediately diminished with the hyperpolarizing voltage and was indistinguishable from the background current endogenous to HEK293 cells (Figure 1—figure supplement 2E,F). For TMEM16F current induced by 15 µM Ca2+ before desensitization, despite the channel closing with the hyperpolarizing voltage, the remaining current was still large enough, distinguishable from the current recorded in 0 Ca2+ (referred to as ‘background current’, Figure 1—figure supplement 2B,C). Consistent with previous reports (Yang et al., 2012; Alvadia et al., 2019), it was moderately selective for Na+ over Cl- (Figure 1E).
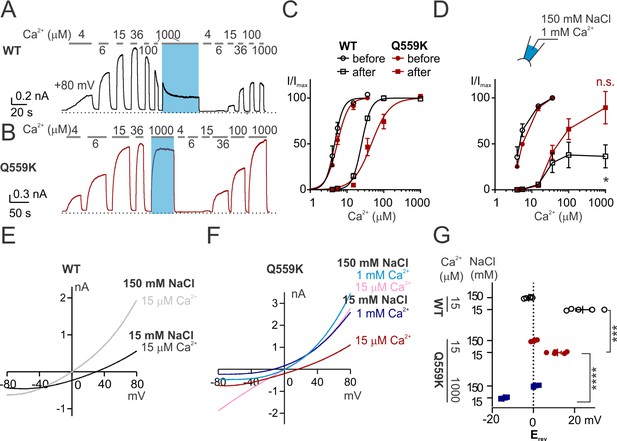
TMEM16F Q559K shifts its reversal potential in response to change of intracellular Ca2+ concentration.
(A, B) Representative recordings of TMEM16F wild type (WT) and Q559K in different Ca2+ concentrations. Traces were recorded from transfected HEK293 cells and the inside-out patches were held at +80 mV. The shades illustrate 1 min treatment with 1 mM Ca2+ that catalyzes PIP2 degradation by membrane-tethered phospholipase. (C) Dose-response curves for Ca2+-activation of WT and Q559K before and after 1 mM Ca2+ treatment, respectively. Currents before and after 1 mM Ca2+ were separately fitted to the Hill equation and normalized to their respective maximal amplitudes. (D) Change of current magnitudes of WT and Q559K. The currents were normalized to the maximal magnitudes before 1 mM Ca2+ for each cell. *p<0.05 (one sample t test against hypothetical value ‘1’). (E, F) Representative I-V relationships of WT and Q559K recorded in indicated conditions. The traces were recorded with a hyperpolarizing ramp from +80 mV to −80 mV (−1 V/s) following holding at +80 mV. (G) Scatter plot of reversal potentials (Erev) obtained from traces as in E and F without background correction. p-Values were determined with Sidak's multiple comparisons following two-way ANOVA.
In contrast, the mutant Q559K current recorded at +80 mV showed minimal rundown in 1 mM Ca2+, in spite of desensitization to Ca2+ activation (Figure 1B) (Alvadia et al., 2019; Ye et al., 2018). Normalized to the respective maximal magnitudes of the currents before and after 1-min-treatment of 1 mM Ca2+, EC50 of Ca2+-activation was shifted from 5.6 ± 0.3 µM to 52 ± 11 µM (Figure 1C), while the normalized fully activated current magnitude was 0.89 ± 0.18 (current in 1 mM Ca2+ normalized to that before 1 mM Ca2+), significantly different from 0.36 ± 0.13 for wild type (Figure 1D). We also measured the steady-state conductance-voltage relationship from −40 mV to +160 mV. Desensitization caused a right-shift of the voltage-dependence of Q559K current in 15 µM Ca2+ (according to the comparison of 15 µM Ca2+-activated current before and after 1 mM Ca2+-treatment, Figure 1—figure supplement 1E,G), but in 1 mM Ca2+ the left-shift overrode the effect of desensitization, with V1/2 being 18 ± 10 mV (Figure 1—figure supplement 1F,H). The left shift of voltage-dependence in 1 mM Ca2+ probably explains the absence of rundown in Q559K current as observed for wild type, since at +80 mV Q559K in 1 mM Ca2+ is activated to a greater level than Q559K in 15 µM Ca2+ (with endogenous PIP2 in cell membrane) or wild type in 1 mM Ca2+ (Figure 1—figure supplement 1). Taken together, the Q559K mutation that minimized rundown allowed us to record TMEM16F current around physiological membrane potentials in a wide-range of Ca2+ concentration, here particularly, to measure Erev in 1 mM Ca2+.
Interestingly, the Erev of TMEM16F Q559K current activated by 1 mM Ca2+ exhibited a left-shift to −14 ± 1 mV in 15 mM NaCl, suggesting that it was more permeable to Cl- than to Na+ (Figure 1F,G), in contrast to 12 ± 2 mV as the Erev of it in 15 µM Ca2+ (recorded with the same protocol as for wild type above). Q559K mutant channels in 1 mM Ca2+ had a lower threshold for voltage activation than in 15 µM Ca2+ (Figure 1—figure supplement 1H), but the current quickly diminished with the hyperpolarizing voltage as in the case of wild-type TMEM16F (Figure 1—figure supplement 2K). The remaining current (confirmed with the ‘tail current’ recorded at +80 mV immediately following the ramp, Figure 1—figure supplement 2L) was nonetheless distinguishable from the background current endogenous to HEK293 cells, revealing that it reversed at a negative membrane potential. The current endogenous to HEK293 cells was partially inhibited in 1 mM Ca2+ (Figure 1—figure supplement 3A,B,C), thus rendering it difficult to measure for background correction in each recording in 1 mM Ca2+. However, with the confirmation that the endogenous current was selective for cation (Figure 1—figure supplement 3A,B,C) so that the ‘real’ TMEM16F-mediated current in 1 mM Ca2+ should reverse at an even lower membrane potential if the contribution of background current could be adequately removed (Figure 1—figure supplement 3F), we chose to take a ‘safer’ step by not performing background subtraction if the comparison involved currents activated by 1 mM Ca2+. Corrected for the liquid junction potential calculated with Clampex, these results indicated that the PNa+/PCl- was 0.47 ± 0.03 in 1 mM Ca2+, in contrast to 2.1 ± 0.4 as calculated from Erev in 15 µM Ca2+.
We asked whether the phenotype of Erev shifting is specific to the introduction of lysine to the position Q559 in TMEM16F. For the data shown above, Erev measurements were made after the solution exchange was complete and the current alteration reached equilibrium, in which case the Erev of wild-type TMEM16F in 1 mM Ca2+ was not measurable. Now we switched to 1 mM Ca2+ when the patch was incubated in 15 mM NaCl and immediately recorded Erev. With this protocol, we found a left-shift of Erev accompanied with rapid current inactivation, until the current was too small to be distinguishable from endogenous current (Figure 1—figure supplement 4A,B,C). Thus, wild-type TMEM16F dynamically underwent the transition of an increased preference for Cl- permeation with the elevation of intracellular Ca2+ concentration. It has been recently reported that mutation of Q559 to aromatic amino acids (such as Q559W) in TMEM16F also prevents current rundown (Nguyen et al., 2019). We measured the Erev of Q559W current activated by 15 µM Ca2+ and 1 mM Ca2+ in 15 mM NaCl with the protocol as for Q559K. Although in each condition the Erev of Q559W was more positive than that of Q559K, the Erev shift persisted, regardless of background subtraction (with background currents recorded in Ca2+-free solutions with the same NaCl concentration) (Figure 1—figure supplement 4D,E,F). The above results showed that TMEM16F increased the relative permeability to Cl- (versus Na+) when Ca2+ concentration was raised from 15 µM to 1 mM, in both wild type and mutants.
TMEM16F has been reported to be permeable to a variety of cations. With the respective published experimental settings, the permeabilities to many physiological cations (Na+, K+, Ca2+) were higher than that to Cl-, while the permeability to some other cations may be lower (Yang et al., 2012; Yu et al., 2015). Among anions, both wild-type TMEM16F and mutant channels are more permeable to I- than Cl- (Figure 1—figure supplement 5) (Nguyen et al., 2019), similar to the preference of CaCC for larger anions (Schroeder et al., 2008; Caputo et al., 2008; Yang et al., 2008). In this study, we will mainly focus on the comparison between Na+ permeability and Cl- permeability unless otherwise stated. Given the dynamic PNa+/PCl- ratio, we could not employ the calculation methods normally used for bi-ionic conditions to analyze data obtained with solutions involving a third ion species. Additionally, for each experiment in the following study, we performed paired-comparison within the same patches to avoid ambiguities arising from large variations across different recordings.
Q559K channel permeability ratio PNa+/PCl- varies with intracellular Ca2+ concentration
We wondered whether the two types of ion selectivity of the Q559K mutant channel correspond to multiple open states. For example, the channel may be in an intermediate open state in 15 µM Ca2+ but a fully open state in 1 mM Ca2+. To test this possibility, we first tested the ion permeability ratio of current activated by 6 µM Ca2+, a condition where both WT and Q559K channels were activated to yield about half of the maximal current magnitudes (Figure 1A,B,C). To obtain accurate measurements, we subtracted the current recorded in Ca2+-free solution (with 15 mM NaCl) from those recorded in 6 and 15 µM Ca2+ for each excised patch. We found that for each recording from either WT or Q559K, the current evoked by 6 µM Ca2+ reversed at a more positive voltage than that by 15 µM Ca2+ (Figure 2A,B,C). To dissociate the effect of Ca2+ concentration from that attributable to different open states of the channel, we made use of the Ca2+-binding-site mutant, E667Q, that exhibits an elevation of EC50 of Ca2+-activation (Yang et al., 2012; Alvadia et al., 2019). The EC50 of the double-mutant, Q559K_E667Q, was 0.88 ± 0.06 mM, suggesting that the double mutant should not be in the fully open state in 1 mM Ca2+ (Figure 2D,E). If the increase of anion permeability only occurs when channel is fully open, the current through Q559K_E667Q channels should reverse at a positive potential in 1 mM Ca2+. Strikingly, the current in 1 mM Ca2+ reversed at −6.9 ± 2.0 mV, corresponding to a channel with higher permeability to Cl- than to Na+ (Figure 2F). This indicates either that TMEM16F ion permeability preference is not correlated with its open state, or that the E667Q mutation circumvents the intermediate open state (if any) and causes the mutant channel to directly enter a fully-open conformation.
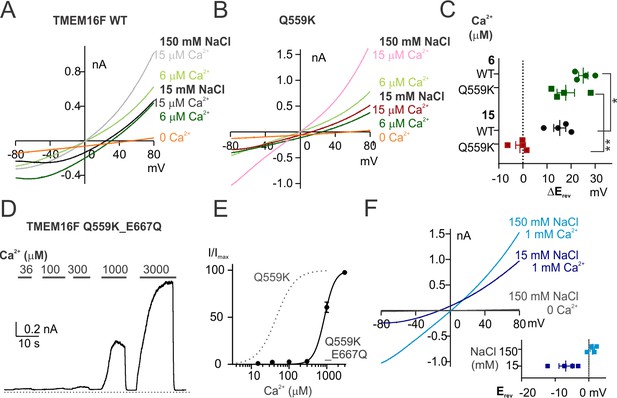
TMEM16F Q559K_E667Q channel is more permeable to Cl- than to Na+ in 1 mM Ca2+despite being half activated.
(A, B) Representative I-V relationships of WT and Q559K currents recorded in indicated conditions. The recording protocol was the same as in Figure 1E,F. Ca2+-free 15 mM NaCl solution was applied at the end for background subtraction. (C) Scatter plot of the changes of reversal potentials (ΔErev) when solution was switched to 15 mM NaCl, obtained from traces as in A and B. p-Values were determined with Fisher's LSD test after two-way ANOVA. (D) Representative recordings of TMEM16F Q559K_E667Q in different Ca2+ concentrations. The recording protocol was the same as in Figure 1A. (E) Dose-response curve for Ca2+-activation of Q559K_E667Q. The gray dotted line represents the curve for Q559K after 1 mM Ca2+, replotted from Figure 1C. (F) Representative I-V relationships of Q559K_E667Q recorded in indicated conditions. The recording protocol was the same as in Figure 1E,F. The insert shows the scatter plot of reversal potentials (Erev) obtained from traces as in F.
To dissociate the correlation between Ca2+ concentration and activation state, we utilized the desensitization phenotype of TMEM16F Ca2+-gating via exposure to high Ca2+. We previously reported that TMEM16F in 36 µM Ca2+ undergoes a slow desensitization with time constant of ~60 s possibly due to the slow degradation of PIP2. TMEM16F Q559K activation by 36 µM Ca2+ reached full or less-than-half open state, respectively, before or after desensitization (Figure 1B,C). We maintained the inside-out patches from Q559K-transfected cells in 36 µM Ca2+ (15 mM NaCl) for 90–100 s and measured Erev once every 10 s (Figure 3). The Erev was left-shifted when the Ca2+ concentration was raised from 15 µM to 36 µM. During the slow desensitization in 36 µM Ca2+, Erev (corrected with subtraction of background current recorded in 0 Ca2+ afterwards) remained unchanged despite the constant decrease of current magnitude and the increase of outward rectification. We therefore concluded that the reversal potential of Q559K shifted only when Ca2+ concentration was changed, independently of current magnitude, open state, or rectification level. Also, although it was not clear whether the permeation pathway could be partially composed of the headgroups of lipids being scrambled, we inferred that at least PIP2, which was slowly depleted during incubation in 36 µM Ca2+, did not contribute to the shift of ion permeability ratio.
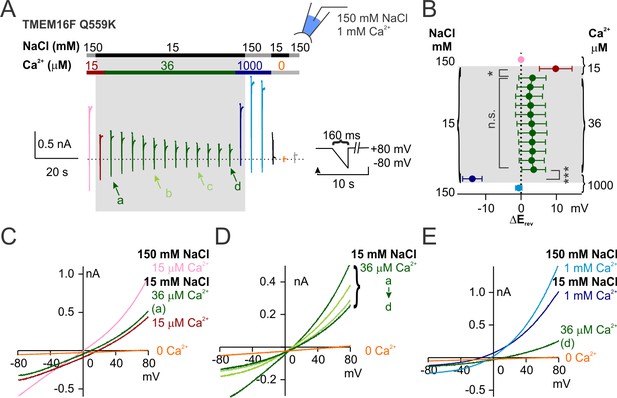
Q559K Erev only shifts with the change of Ca2+ concentration.
(A) Representative recording of TMEM16F Q559K held at +80 mV with a hyperpolarizing ramp (−1 V/s) once every 10 s in indicated conditions. (B) Summary of the averaged Erev with background subtraction (subtraction of the current in Ca2+-free 15 mM NaCl solution, orange trace in A, except for the current in 1 mM Ca2+) in indicated conditions. For all the traces recorded in 15 mM NaCl, Erev only shifts when Ca2+ concentration is changed. (C, D, E) Representative I-V relationships of Q559K at arrowed points in A, showing that Erev shifts when Ca2+ is increased from 15 µM to 36 µM, persists in 36 µM Ca2+ despite the constant rundown, and shifts when Ca2+ is increased from 36 µM to 1 mM.
We investigated whether the shift of relative ion permeability involves the conformational change of TM6, a critical step in channel activation. In TMEM16A, the glycine in TM6 (G640 or G644 depending on isoform) works as a hinge to allow the rearrangement of the helical segments during channel activation to generate the ionic permeation pathway (Paulino et al., 2017a; Peters et al., 2018). Alanine substitution of this glycine stabilizes TM6 at the open conformation and increases TMEM16A Ca2+ sensitivity (Paulino et al., 2017a; Peters et al., 2018). This glycine hinge in TMEM16A TM6 corresponds to G615 in TMEM16F according to sequence alignment (Figure 4—figure supplement 1A). Cryo-EM studies reveal that TM6 is bent at this glycine hinge in Ca2+-free but not Ca2+-bound TMEM16F (Feng et al., 2019). Previous studies have shown that its alanine substitution (G615A) increases Ca2+ sensitivity (Alvadia et al., 2019; Han et al., 2019), and we confirmed that G615A left-shifted the voltage dependence of channels recorded in 15 μM Ca2+ (Figure 4A,D). In contrast, mutation of the adjacent glycine, G614A, did not have similar or further effects (Figure 4—figure supplement 1E,F,G). We also confirmed the effect of G615A mutation by comparing its voltage-dependent activation with another two TM6 mutants, I612A and N620A, which were chosen because the alanine substitutions of their corresponding amino acids in TMEM16A (I637A or I641A, Q645A or Q649A, depending on isoform) represent the stabilization of the two steps of TM6 conformational rearrangement during TMEM16A activation, respectively (Peters et al., 2018). TMEM16F I612A, mutation of the isoleucine in TM6 upper segment, caused shifts in Ca2+- and voltage-gating by an extent comparable with those of G615A (Figure 4—figure supplement 1D,G) (Han et al., 2019), indicative of a relationship similar to that between TMEM16A G644A and I641A (amino acid labels using TMEM16A isoform as in Lam and Dutzler, 2018; equivalent to G640A and I637A for the isoform as in Peters et al., 2018 ), indicating that the two mutants might be stabilized in the same state. In the lower segment, TMEM16A Q649 (Q645 in Peters et al., 2018 ), whose alanine substitution facilitates channel opening (Peters et al., 2018; Lam and Dutzler, 2018), corresponds to a gap of the alignment in TMEM16F (Figure 4—figure supplement 1A); alanine substitution of the asparagine ‘5-amino-acid-away’ in TMEM16F TM6, N620, did not facilitate Ca2+ (Han et al., 2019) or voltage gating (Figure 4—figure supplement 1B,C). Taken together, mutation of G615 to alanine (G615A) might sufficiently stabilize TM6, which in wild-type TMEM16F undergoes rearrangement to generate the ionic permeation pathway.
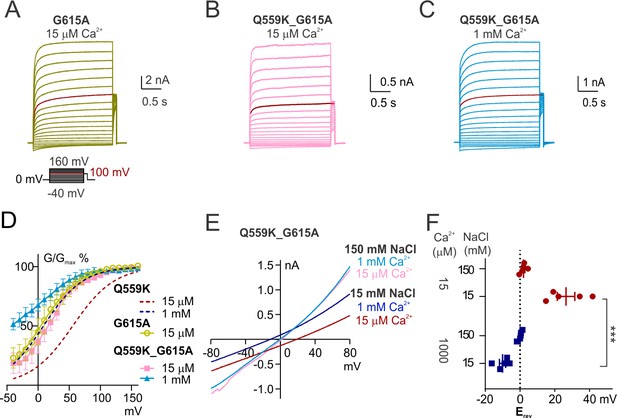
The change of ion permeability ratio is preserved despite TM6 conformational stabilization.
(A, B, C) Representative traces of G615A and Q559K_G615A recorded with a voltage family protocol as in Figure 1—figure supplement 1. The currents recorded at +100 mV are highlighted for comparison. (D) Averaged G-V relationships of G615A and Q559K_G615A currents. The method for data analysis was the same as that for WT in 15 µM Ca2+. The two traces for Q559K were replotted from Figure 1—figure supplement 1H. (D) Representative I-V relationships of Q559K_G615A recorded in indicated conditions. (F) Scatter plot of reversal potentials (Erev) obtained from traces as in E without background correction. p-Values were determined with Sidak's multiple comparisons following two-way ANOVA.
Notably, the steady-state voltage dependence of TMEM16F G615A channel in 15 μM Ca2+ was comparable to that of Q559K in 1 mM Ca2+ (Figure 4A,D). We then generated the double-mutant Q559K_G615A, which in 1 mM Ca2+ showed further left-shift of G-V relationship (Figure 4B,C,D). Recorded with the fast hyperpolarizing ramp, the current was almost linear, consistent with the notion that removal of the glycine hinge stabilizes the open conformation (Figure 4—figure supplement 2). The change of the permeability ratio PNa+/PCl- with rising Ca2+ in this double mutant was similar to that of Q559K (and wild-type) TMEM16F (Figure 4E,F). This double mutant revealed that in the presence of Q559K mutation, TMEM16F channel in 15 μM Ca2+ was modestly more permeable to Na+, but it became more permeable to Cl- in 1 mM Ca2+ regardless of TM6 stabilization. Taken together, these results suggest that TMEM16F ion selectivity more likely depends on intracellular Ca2+ concentration directly rather than its open state(s), although it remains possible that TMEM16F might undergo miniscule conformational changes at different Ca2+ concentrations that contribute to the transition of relative permeability.
The shift of permeability ratio correlates with electrostatic change in permeation pathway
Intrigued by the possibility that Ca2+ alters anion accessibility to the TMEM16A pore through electrostatic effect (Lam and Dutzler, 2018), we hypothesize that the shift of permeability ratio of TMEM16F Q559K in response to elevation of intracellular Ca2+ is due to a change of electrostatic field along its permeation pathway. In this scenario, the effect ought to be elicited not only by Ca2+ but also by other divalent or trivalent cations (Lam and Dutzler, 2018). We chose to test Zn2+ and Gd3+, divalent and trivalent cations that are smaller in ionic radius than Ca2+ but capable of activating TMEM16F. Both Zn2+- and Gd3+-activations of TMEM16F were coupled with rapid inactivation even at low concentrations (Figure 5—figure supplement 1A,D), precluding the possibility to accurately plot the dose-response curves. The exposure to divalent-free solution (with EGTA) for ~30 s to 1 min following channel inactivation by Zn2+ allowed the current to fully recover in magnitude (Figure 5—figure supplement 1B,C), suggesting that the rapid current rundown was not attributed to desensitization triggered by PIP2 degradation, although it is unclear whether Zn2+ can shield the negative charges in PIP2 headgroups to elicit a reversible rundown.
We found that the wild-type TMEM16F channels activated by 10 μM Zn2+ were more permeable to Na+ than to Cl-, as evident from the right-shift in Erev as intracellular NaCl dropped to 15 mM (Figure 5A). The Erev in 1 mM Zn2+ could not be determined owing to the rapid current inactivation, so we again used the Q559K mutant to this end. Notably, TMEM16F Q559K channels exhibited an increased relative permeability to Cl- (versus to Na+) with elevation of Zn2+ concentration from 10 μM to 1 mM (Figure 5B,C). In contrast to the drastic inactivation in 1 mM Zn2+, wild-type TMEM16F current activated by Gd3+, even with rundown, was still distinguishable from background current at both low and high concentrations. The remaining current underwent the Erev shift when Gd3+ concentration was switched from 10 µM to 1 mM (Figure 5D,E,F). Note that wild-type TMEM16F current activated by Gd3+ showed weaker outward rectification (Figure 5E, compared with Figure 1—figure supplement 3D). However, the steady-state conductance-voltage relationship (Figure 5—figure supplement 1E,F) suggested that, instead of being activated independently of depolarization, the change of rectification was more likely due to a delayed channel closing during hyperpolarization.
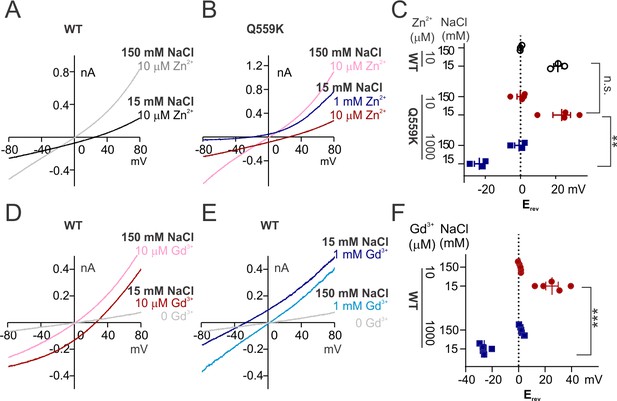
The change of ion permeability ratio is preserved when current is activated by Zn2+ or Gd3+.
(A, B) Representative I-V relationships of WT and Q559K recorded in indicated Zn2+-containing solutions. The currents were recorded with the same protocol as in Figure 1E,F. (C) Scatter plot of reversal potentials (Erev) obtained from traces as in A and B. p-Values were determined with Sidak's multiple comparisons following two-way ANOVA. (D, E) Representative I-V relationships of WT TMEM16F recorded in indicated Gd3+-containing solutions. Note that the current in 1 mM Gd3+ 15 mM NaCl is still distinguishable from background current. (F) Scatter plot of reversal potentials (Erev) obtained from traces as in E and F. p-Values were determined with Sidak's multiple comparisons following two-way ANOVA.
Although monovalent ions generate weak electrostatic fields compared with divalent and trivalent ions, given their abundance they should also be able to modulate the electrostatic field along TMEM16F permeation pathway and alter the relative ion permeability. TMEM16F is permeable to most generally-used cations including N-methyl-D-glucamine (NMDG) and tetraethylammonium (TEA) (Yang et al., 2012; Yu et al., 2015), so introducing any other ion species will cause difficulties in distinguishing whether the shift of Erev is due to the change of PNa+/PCl- or to the permeation of the new ion species. Thus, we first calculated the respective permeability ratios from the measured Erev with different intracellular NaCl concentrations (Figure 1—figure supplement 2). Compared with 15 mM NaCl, the stronger ionic strength of 45 mM intracellular NaCl enhances the screening effect for anions, and thus is predicted to increase the relative permeability to Cl-. Indeed, the calculated PNa+/PCl- values measured in 15 µM Ca2+ were 4.8 ± 0.6 and 2.3 ± 0.2 for wild-type TMEM16F in 15 mM and 45 mM NaCl, respectively; and the calculated PNa+/PCl- values measured in 15 µM Ca2+ were 2.1 ± 0.4 and 1.4 ± 0.1 for Q559K in 15 mM and 45 mM NaCl, respectively (Figure 6), consistent with previous findings that Q559K reduces PNa+/PCl- (Yang et al., 2012; Alvadia et al., 2019). The PNa+/PCl- for Q559K in 1 mM Ca2+ showed no significant difference between 15 mM NaCl and 45 mM NaCl (Figure 6), suggesting that millimolar Ca2+ outweighs Na+ in controlling the electrostatic effect. The shift of Erev by changing the intracellular NaCl is also consistent with our proposed scenario that the shift of ion permeability ratio is not due to TM6 conformational change associated with different open states, but instead, due to the alteration of electrostatic field along the permeation pathway.
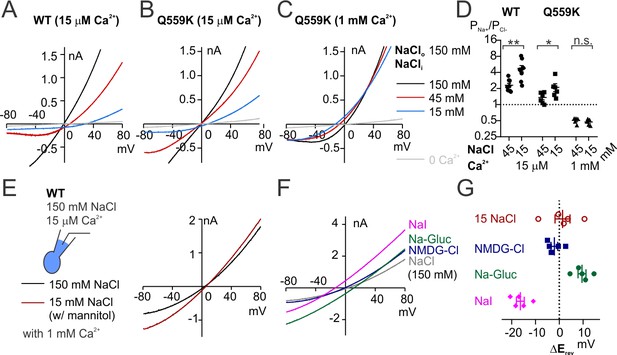
Ion permeability ratio is altered with change of intracellular NaCl concentration.
(A, B, C) Representative I-V relationships of WT and Q559K recorded with inside-out configuration in indicated conditions. The currents were reanalyzed from the recordings as in Figure 1—figure supplement 2. Notice NOT to directly compare the shift of Erev because the intracellular NaCl concentrations are varying. (D) Scatter plot showing the permeability ratio (PNa+/PCl-) calculated from the shift of reversal potentials (ΔErev) obtained from traces as in A, B and C. p-Value for WT was determined with Wilcoxon test. P values for Q559K were determined with Sidak's multiple comparisons following two-way ANOVA. (E, F) Representative I-V relationships of wild-type TMEM16F currents recorded with whole-cell configuration in indicated bath solutions. NMDG: N-methyl-D-glucamine; Gluc: gluconate. (G) Scatter plot of the changes of reversal potentials (ΔErev) obtained from recordings as in E and F. Due to the potentially varying PNa+/PCl-, we did NOT perform statistics or use them to calculate ion permeability ratios.
The minor shift of relative ion permeability by the change of intracellular NaCl concentration shown above might account for the discrepancy regarding TMEM16F ion selectivity recorded with various methods used by different labs. In the experiments above, the osmolarities of low NaCl solutions were all balanced with mannitol to avoid the interference by other ion species. Now, we tested for 15 mM NaCl with equal ionic strength as extracellular solution, which was balanced either with 135 mM NMDG-MES (MES: methanesulfonic acid) or 68 mM NMDG2-SO4. We observed that the wild-type TMEM16F activated by 15 µM Ca2+ in both conditions reversed at ~0 mV (2 ± 1 mV in 15 mM NaCl with NMDG-MES, 3 ± 2 mV in that with NMDG2-SO4; Figure 6—figure supplement 1A,C). Compared with Erev recorded in 15 mM NaCl with mannitol, the reduction of Erev might reflect a combination of NMDG permeation and the reduction of PNa+/PCl-, assuming the permeation of MES- or SO42- was negligible. In neither condition could we determine the Erev for currents activated by 1 mM Ca2+. Using the mutant Q559K, we confirmed that in both conditions, the shift of Erev with rising Ca2+ persisted (Figure 6—figure supplement 1B,D,E), which could be explained by an increase of PCl-/PNa+ or a robustly increased permeation of NMDG, with the latter being less likely.
To confirm that the shift of ion permeability ratio does not depend on recording configuration, we altered the compositions of the pipette solution rather than those of the bath solution. We applied 15 mM NaCl balanced with mannitol, NMDG-MES or NMDG2-SO4 to the pipette solution (equivalent to extracellular solution), while the bath (equivalent to intracellular solution) contained 150 mM NaCl. With the correction of liquid junction potentials (12 mV, 6 mV and 2 mV respectively), the currents reversed at ~0 mV when activated by 15 µM Ca2+ in all the conditions (−1 ± 1 mV, 4 ± 2 mV and −1 ± 2 mV, respectively), while at 11 ± 2 mV, 19 ± 1 mV and 24 ± 1 mV, respectively, when activated by 1 mM Ca2+ (Figure 6—figure supplement 2). For the conditions involving NMDG, the Erev shift either indicated an increased PCl-/PNa+ or a robustly increased permeation of NMDG, with the latter being less likely. These results also complemented the previous tests involving changes of intracellular NaCl concentration (Figure 6A–D), showing that enhancement of intracellular ionic strength ‘unidirectionally’ promotes the relative permeability to Cl-. This can be attributed either to the preceding depolarization which drives cations into the pore, or to the possibility that the pore intrinsically adopts cations to modulate the electrostatic field. In addition, there was no significant difference in Erev shifting between solutions balanced with NMDG-MES and that with NMDG2-SO4, suggesting that the divalent anions are relatively inert whether being applied intracellularly or extracellularly, attributable to the inaccessibility of divalent anions to the permeation pathway.
The above results suggest that TMEM16F ion selectivity is modulated by the change of electrostatic field along its permeation pathway, with divalent or trivalent cations having stronger impacts than monovalent ions. This might account for the lower selectivity for Na+ over Cl- obtained from whole-cell recording, where intracellular solution was usually an isotonic salt solution. In whole-cell recording, TMEM16F current is reported to be elicited a few minutes after the whole-cell configuration is formed, and less selective for cations or in certain conditions even more selective for Cl-. Based on results reported above, we could infer that in these conditions the intracellular ionic strength (mainly maintained by ~150 mM NaCl or KCl) would reduce the channel relative permeability to cations. We performed whole-cell recording with 150 mM NaCl and 15 µM Ca2+ in the pipette, the Erev was 1.4 ± 3.1 mV in 15 mM NaCl, indicative of PNa+/PCl- of 1.0 ± 0.1 (Figure 6D,F). Replacement of extracellular Na+ with NMDG did not significantly shift Erev, indicative of an increased permeability to Cl- and/or a modest permeability to NMDG. Replacement of extracellular Cl- with gluconate (Gluc) or I- shifted Erev to positive or negative values respectively, suggesting the presence of Cl- conduction (Figure 6E,F) as observed in previous studies. However, we could not calculate the permeability ratios due to the potentially varying PNa+/PCl- in these conditions. Notably, it is also reported that Tmem16f-transfected cells undergo an ‘unconventional exocytosis’ which expands the membrane surface area dramatically during recording (Bricogne et al., 2019), leading to challenges in performing membrane capacitance compensation. These technical issues rendered it difficult to perform accurate measurements with whole-cell recording.
Taken together, the elevation of intracellular cation level underlies the increased permeability to Cl-, with divalent cations (Ca2+ and Zn2+) and trivalent cations (Gd3+) having stronger effects than monovalent ions (Na+ and NMDG). This suggests that TMEM16F employs a charge-screening mechanism to dynamically alter the preference for permeating ion species: Increase of positive charges, by introduction of basic amino acids or by entry of cations, enhances the channel preference for anions. This mechanism allows TMEM16F to open as a non-selective ion channel at low intracellular Ca2+ level and as a Cl- channel when local Ca2+ concentration increases, indicative of physiological functions that have not been investigated thus far.
Depolarization alters permeability ratio synergistically with intracellular Ca2+
Previous studies have shown the synergy between Ca2+ and depolarization in the gating of TMEM16A. Depolarization facilitates Ca2+ entry into the membrane electric field and thus reducing the EC50 for channel activation by Ca2+ (Xiao et al., 2011; Peters et al., 2018). The reduction of EC50 of Ca2+ activation by depolarization is also observed in TMEM16F (Yang et al., 2012; Ye et al., 2018), although TMEM16F permeates Ca2+ (Yang et al., 2012), suggesting Ca2+ efflux is not fast enough to deplete Ca2+ from the electric field at the intracellular side. We asked whether depolarization also increases TMEM16F permeability to Cl- by driving Ca2+ into the membrane electric field. To this end, we held the excised membrane patch at potentials ranging from +40 to+160 mV with increments of 40 mV (referred to as ‘conditioning potentials’) followed by a hyperpolarizing ramp from +80 mV to −80 mV (−2 V/s), to measure the Erev (Figure 7C). With this experimental design, the shift of Erev following different conditioning potentials could provide an indication of the changes of relative permeability, under the circumstance that during hyperpolarization TMEM16F can temporarily ‘memorize’ the ion permeability ratio at the preceding conditioning potential. We measured the Erev of TMEM16F wild-type channels in 15 µM Ca2+, Q559K channels in 15 µM and in 1 mM Ca2+. In 15 µM Ca2+, the more depolarized the conditioning potential, the higher the relative Cl- permeability (versus Na+ permeability) (Figure 7A,D). The traces recorded with the hyperpolarizing ramp following different conditioning potentials did not intersect with each other, confirming the Erev alteration even though we did not perform background subtraction. The shift of Erev was not a potential artifact caused by ion accumulation or depletion as previously investigated for other channels (Li et al., 2015; Yu et al., 2014), since if it was, a more depolarized conditioning potential would cause more severe Na+ accumulation or Cl- depletion at the extracellular side (in the pipette solution), leading to a right-shift of Erev, which was opposite to our results. Traces of Q559K currents following different conditioning potentials in 1 mM Ca2+ overlapped at low membrane voltages (Figure 7E), a phenomenon observed for TMEM16A in 0.2 µM Ca2+ (Figure 7B). The gradual increase of relative Cl- permeability caused by preceding depolarization can be attributed to intracellular cations, particularly Ca2+, to the membrane electric field to enhance the attraction of permeation pathway to anions, as can be inferred from the charge-screening mechanism.
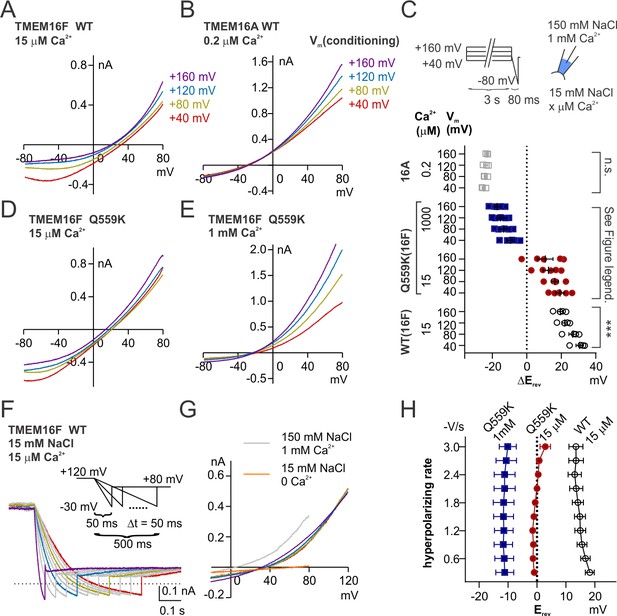
Depolarization alters permeability ratio synergistically with Ca2+ level.
(A, B, D, E) Representative I-V relationships of currents recorded in indicated conditions. The excised patch was held at +40 to+160 mV with increments of 40 mV (‘conditioning potentials’) followed by a hyperpolarizing ramp from +80 mV to −80 mV (−2 V/s). (C) Scatter plot showing the changes of reversal potentials (ΔErev) when solution was switched from 150 mM NaCl to 15 mM NaCl. For TMEM16A and WT TMEM16F, p-values were determined with one-way ANOVA. For TMEM16F Q559K, two-way ANOVA shows p<0.001 across voltages, p<0.0001 across Ca2+ concentrations. (F) Representative WT TMEM16F traces recorded in 15 µM Ca2+ 15 mM NaCl bath solution with the indicated protocol. The excised patch was held at 120 mV followed by a hyperpolarizing ramp from +120 mV to −30 mV (ramping speed from −0.3 V/s to −3 V/s), and the reversal potentials were corrected with background current recorded at the end. (G) The I-V relationships of the highlighted traces as in F, showing the currents reverse at the same point despite the change of rectification. (H). Summary of reversal potentials at different hyperpolarizing speeds obtained from traces as in G. Two-way ANOVA suggests that there is no significant difference among various hyperpolarizing speeds (p=0.28).
The observation that TMEM16F in 15 µM Ca2+ is able to ‘memorize’ the ion permeability ratio at the preceding conditioning potential, suggests that the electrostatic field along the permeation pathway can be maintained even when there is no movement of net charge (i.e. 0 pA). We then recorded the Erev with hyperpolarizing ramps of variable speeds (0.3 V/s to 3 V/s) following holding the patch at +120 mV, to test whether the channel would ‘forget’ the ion permeability ratio at the conditioning potential (+120 mV) by observing Erevs under hyperpolarization ramps of various rates. We did not see a significant shift of the Erev for both WT and Q559K, although wild-type TMEM16F current became more outwardly rectifying at slower hyperpolarizing ramps (Figure 7F,G, Figure 7—figure supplement 1). Thus, the permeability ratio (PCl-/PNa+) can be maintained during hyperpolarization, raising the possibility that the Ca2+ ion that modulates the pore electrostatic field dwells at the permeation pathway or a proximal site that allows it to still affect permeation pathway instead of leaving the pore with the ionic flow.
We summarized the mechanism of TMEM16F dynamic ion selectivity based on the experiments shown above and published previously (Figure 8). TMEM16F ion permeation pathway is opened as a result of protein conformational change, which probably involves the rearrangement of TM6 induced by Ca2+-binding to the conserved Ca2+-binding pocket. The elevation of intracellular divalent or trivalent cation concentration brings positive charges into the permeation pathway, which gradually increases the relative permeability of anions. Depolarization promotes the pore attraction to anions by facilitating Ca2+ entry into the membrane electric field, wherein it may remain until the channel is closed.
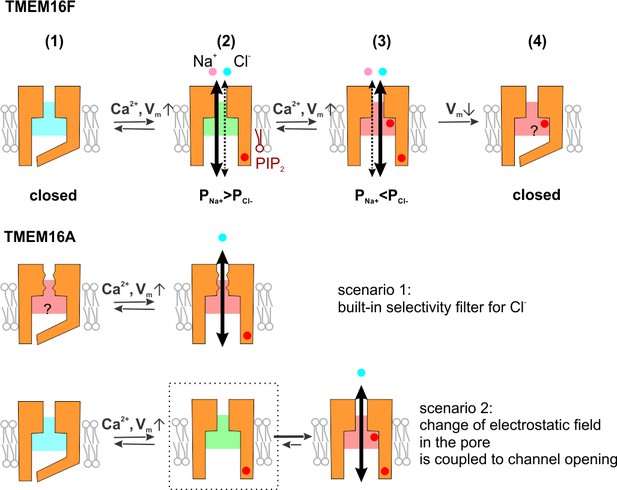
Diagram showing the proposed ion selectivity mechanism for TMEM16F and a comparison with TMEM16A.
(Upper) TMEM16F gating and ion selectivity. The binding of intracellular Ca2+ to the binding-pocket triggers the rearrangement of transmembrane helices and opens the ion permeation pathway (from 1 to 2). The elevation of intracellular Ca2+ level shifts the electrostatic field along the permeation pathway from a Na+-favoring state (shaded with cold color) to that with increased attraction to Cl- (shaded with warm color, from 2 to 3). Each red dot represents a Ca2+-entry event, while the number of Ca2+ ions for every event is not specified. Depolarization drives Ca2+ into both the binding pocket and the permeation pathway; PIP2 stabilizes the open conformation but does not affect the ion selectivity. Hyperpolarization triggers the closing of the channel, preceding the retreating of Ca2+ from the site that allows it to affect the permeation pathway, so that the channel maintains the electrostatic field and ‘memorizes’ the permeability ratio (from 3 to 4). (Lower) TMEM16A might harbor a selectivity filter restrictedly selective for anions (scenario 1), either with a size-dependent mechanism (illustrated with the zigzags) or a strong electrostatic field (illustrated with red shade). Alternatively, the change of electrostatic field might be tightly coupled to Ca2+-gating (scenario 2), so that the pore restrictedly selects for anions once it is opened.
Discussion
Studies regarding TMEM16F physiological functions have mostly focused on its phospholipid scrambling activities, while the understanding of its ion channel functions is confounded by the discrepancy of ion selectivity measurements. In cells with TMEM16F expression, increasing cytoplasmic Ca2+ concentration leads to activation of TMEM16F channel that mediates Ca2+ influx (Feng et al., 2019; Han et al., 2019), which further activates phospholipid scrambling. This is consistent with the results that TMEM16F recorded with inside-out excised patch showed higher permeability to cations than to Cl- (Yang et al., 2012; Alvadia et al., 2019), notwithstanding the whole-cell recording indicating that delayed-activated TMEM16F is an anion channel (Grubb et al., 2013). In light of the Q559K mutant which displays minimal rundown and less outward rectification in 1 mM Ca2+, we measured TMEM16F reversal potentials at different Ca2+ concentrations, revealing a shift toward Cl--selectivity at high intracellular Ca2+. This shift of ion permeability ratio correlates with the change of electrostatic field in the permeation pathway, which is determined by both intracellular ion concentration and membrane potential; divalent cations regardless of ion species are expected to exert stronger electrostatic effects than monovalent cations. This might reflect a general feature in the coupling of gating and conduction for channels in the TMEM16 family, likely relevant to their physiological functions.
Gating and ion selectivity of TMEM16F
The ion permeation pathway of TMEM16F is generated probably through the rearrangement of TM6 in response to elevation of intracellular Ca2+ concentration, as reflected in its conformation in the presence or absence of Ca2+ (Feng et al., 2019), a mechanism similar to that of TMEM16A. Further elevation of intracellular Ca2+ results in an increase of relative permeability to Cl- (versus Na+). With membrane potential and ionic strength unchanged, the permeability ratio PNa+/PCl- is only correlated with Ca2+ concentration rather than Ca2+-induced conformational change, suggesting that the ion selectivity machinery is not coupled to channel gating. At low Ca2+ when channels display modest selectivity between Na+ and Cl-, the ion permeability ratio is also altered by monovalent ions and membrane potential, which indicates that the ion selectivity filter is sensitive to electrostatic disturbance and thus might explain the discrepancy of experimental results from different labs. Depolarization, which drives Ca2+ into membrane electric field and facilitates Ca2+-gating, synergistically promotes the permeability alteration towards a preference for Cl-. The ion permeability ratio can be memorized during the recording with a hyperpolarizing ramp, suggesting that Ca2+ retreating from the site that affects the permeation pathway is preceded by the dissociation of Ca2+ from the binding pocket, so that the pore electrostatic field can be maintained until the channel is closed. PIP2 reduces the EC50 for Ca2+-activation but does not affect ion selectivity, thus facilitating TMEM16F opening when the pore is still more attractive to cations in low intracellular Ca2+. Controlling the selectivity between cations and anions by altering the pore potential, referred to as a charge-screening mechanism, is also documented in other ion channels, such as ligand-gated channels (Keramidas et al., 2004) and bacterial porins (Alcaraz et al., 2004), through charged residues lining the pore and/or through permeating ions within the pore.
It is intriguing to consider the structural basis for Ca2+ regulation of the TMEM16F relative ion permeability. Specifically, we wonder whether Ca2+ ion(s) in the Ca2+-binding pocket, or in the permeation pathway, may alter the electrostatic field of the pore. The former resembles what has been proposed for TMEM16A (Lam and Dutzler, 2018), whose pore is electrostatically modulated by Ca2+ bound to the pocket, but it seems unlikely for TMEM16F, because the Hill coefficient of Ca2+-activation of TMEM16F is ~2, suggesting that the two Ca2+-binding sites should both be occupied when the current displays higher permeability to cations. The latter scenario is that there is room for Ca2+-dwelling in the permeation pathway, consistent with the recently-published TMEM16F structure in which the pore harbors a large vestibule (Alvadia et al., 2019; Feng et al., 2019). Also, its distant homolog, TMEM16K, has multiple Ca2+ ions bound to its cytoplasmic domains (Bushell, 2018), raising the question whether there could be a reservoir in the TMEM16 cytoplasmic domain to store Ca2+. Given the long range electrostatic actions, it will be technically challenging to identify the structural basis for the electrostatic modulation in the pore.
Comparison among TMEM16 members
TMEM16 represents a family of membrane proteins with diverse functions, including Ca2+-activated ion channel activities and Ca2+-activated lipid scrambling. Mammalian TMEM16A and TMEM16B are Ca2+-activated Cl- channels (Schroeder et al., 2008; Caputo et al., 2008; Yang et al., 2008; Stephan et al., 2009), while other members with phospholipid scrambling functions display modest cation-selective or non-selective ion channel activities, such as TMEM16E (Whitlock et al., 2018), TMEM16F (Yang et al., 2012; Alvadia et al., 2019; Yu et al., 2015; Le et al., 2019a), Drosophila subdued (Le et al., 2019b), and two fungal TMEM16 homologs (Malvezzi et al., 2013; Brunner et al., 2014; Lee et al., 2016; Jiang et al., 2017). The lipid scrambling activity is also observed in an amoebozoa TMEM16 homolog (Pelz et al., 2018). All the TMEM16 proteins, based on reported structures and sequence alignment, are dimers with each monomer containing 10 transmembrane helices with conserved Ca2+-binding sites (Alvadia et al., 2019; Brunner et al., 2014; Paulino et al., 2017a; Bushell, 2018; Dang et al., 2017; Feng et al., 2019; Kalienkova et al., 2019; Falzone et al., 2019). Here, our studies reveal that TMEM16F displays a preference for Cl- conduction when Ca2+ concentration is high, potentially indicative of a unifying mechanism regarding TMEM16 channel functions. In the following paragraphs, we will briefly discuss the similarities and differences among TMEM16 members, and focus mainly on the comparison between TMEM16A and TMEM16F.
In both TMEM16A and TMEM16F, the opening of the permeation pathway involves the conformational stabilization of TM6, while the removal of a glycine hinge reduces the Ca2+ level required for activation (Paulino et al., 2017a; Peters et al., 2018). Alanine substitution of the glycine reduces EC50s of Ca2+-activation in both TMEM16A and TMEM16F. However, TMEM16A and TMEM16F currents differ in rectification level (Nguyen et al., 2019). The current of TMEM16A bound to one Ca2+ ion (at low intracellular Ca2+ level) is outwardly rectifying, while at high intracellular Ca2+ level that allows two Ca2+ ions to occupy the binding-pocket, the TMEM16A channel conductance becomes ‘Ohmic’ (Peters et al., 2018). Such a transition can be explained by two mutually compatible mechanisms: the second Ca2+ ion triggers the further rearrangement of TM6 (Peters et al., 2018) and its positive electrostatic field reduces the energy barrier that intracellular anions need to overcome to access the permeation pathway (Lam and Dutzler, 2018). In contrast, TMEM16F current is outwardly rectifying in a wide range of Ca2+ concentration. It is important to keep in mind that TMEM16F rectification indices obtained from the traces recorded with ramp protocols (such as in Figure 1E,F) can be confounded with the voltage-dependent gating. However, according to the ‘instantaneous rectification index’ (Alvadia et al., 2019), which describes the magnitude ratio of outward current to inward current within the time range only allowing minimal change of gating, the TMEM16F pore is intrinsically outwardly rectifying, and wild type and Q559K channels are likely rectifying to a similar extent (Alvadia et al., 2019). Thus, in contrast to the corresponding pore-lining residue K588 in TMEM16A, which electrostatically interacts with the permeating Cl- and is necessary for the ohmic conductance (Paulino et al., 2017b; Nguyen et al., 2019), the reduction of outward rectification of TMEM16F Q559K mutation might result from the alteration of voltage gating. Interestingly, the TMEM16F current activated by 1 mM Gd3+ is potentially approaching the ‘ohmic’ state (Figure 5E), suggesting that the strong electrostatic field of a trivalent cation bound to the protein could enhance the accessibility of intracellular Cl- to the pore.
We propose two scenarios to explain why TMEM16F displays a shifting ion selectivity, while TMEM16A selects for Cl- at all Ca2+ concentrations (Figure 8). In one scenario, TMEM16A has a ‘built-in’ selectivity filter that favors anions, so that only anions can eventually permeate even though both anions and cations can access the pore. Such a ‘built-in’ selectivity filter can either be a size-based screening structure, or a constantly strong positively-charged field to attract anions and repel cations. In the second scenario, TMEM16A couples the change of the electrostatic field to the opening of the pore, in a way that the former event precedes or synchronizes with the latter. We tried to distinguish between the two scenarios by measuring the ion selectivity of TMEM16A activated by low intracellular Ca2+. Notably, TMEM16A current activated by 100 nM Ca2+ or 300 nM Ca2+ is Cl--selective, with the selectivity measured in low NaCl balanced with NMDG-MES being more selective for Cl- (compared with Na+) than that in low NaCl balanced with mannitol (Figure 8—figure supplement 1A,B,C), a phenomenon consistent with a previous report (Lim et al., 2016). To probe TMEM16A ion selectivity in 0 Ca2+, we also generated the Q649A mutant of TMEM16A, which is activated by depolarization in Ca2+-free solution (Peters et al., 2018; Lam and Dutzler, 2018), and we used poly-L-lysine (PLL) to strip off membrane-tethered PIP2 to accelerate current rundown (De Jesús-Pérez et al., 2018). We noticed that TMEM16A Q649A activated by 30 nM Ca2+ was selective for Cl- before or after PLL application, but the ‘rundown’ component (current in 0 Ca2+) displayed a dispersed distribution of Erev (Figure 8—figure supplement 1D–I). Because PLL also triggered the rundown of the background current endogenous to HEK293 cells, which is cation selective (Figure 8—figure supplement 1J), we could not draw a definitive conclusion at this point.
Functional implication
The shift of relative ion permeability with elevation of Ca2+ concentration may dynamically regulate membrane potential of the cell. Since TMEM16F can function as a Ca2+-activated Ca2+-permeable channel, the dynamic increase of Cl- permeability (versus cation) might provide a brake to the positive feedback to reduce the influx of cations including Ca2+. In a typical neuronal cell, such a transition towards Cl- selectivity might reduce the entry of Ca2+ into the cell and drive membrane potential close to Cl- equilibrium potential, both of which will reduce the neuronal excitability and prevent Ca2+-overloading. The ‘memory’ of the permeability ratio during repolarization allows TMEM16F to modulate the waveform of action potentials in accordance to local cytoplasmic Ca2+ level. In non-excitable cells, this function might enable TMEM16F to sense cellular Ca2+ level and modulate membrane potential, which affects cellular proliferation and differentiation (Sundelacruz et al., 2009). It has also been reported that TMEM16F is localized in primary cilia (Forschbach et al., 2015), a special cellular compartment with elevated Ca2+ level and greater depolarization (Delling et al., 2013), providing a condition for TMEM16F to function dynamically. With the broad expression pattern of TMEM16F, we envision a new perspective to examine the functions of TMEM16F.
Materials and methods
Cell culture and molecular biology
Request a detailed protocolMouse Tmem16f and Tmem16a cDNAs (sequences as in NCBI RefSeq NM_175344.4, Mus musculus Ano6-splice variant 2, and NM_178642.5, Mus musculus Ano1-splice variant 1, respectively) were respectively fused with mCherry and subcloned into pcDNA3, as previously reported (Ye et al., 2018). Site-directed mutagenesis was performed using standard molecular techniques with pHusion polymerase (New England Biolabs, Ipswich, MA) and sequences were all verified (Quintara Biosciences, South San Francisco, CA). HEK (Human embryonic kidney)−293 cells (ATCC, RRID: CVCL_0045) were aliquoted and preserved in liquid nitrogen. One vial of cells was thawed and plated in 10-mL cell culture flask (ThermoFisher Scientific, Waltham, MA) once every 3 months. Cell were cultured in Dulbecco's Modified Eagle Medium (DMEM, with 4.5 g/L glucose, L-glutamine and sodium pyruvate, Mediatech, Manassas, VA) containing 10% FBS (Axenia BioLogix, Dixon, CA) and 1% penicillin-streptomycin, at 37°C and with 5% CO2, and they were passaged into a new flask with a 1:10 ratio once every 3 ~ 4 days. They were disposed of if they were not confluent in the flask in 4 days, their morphologies deviated from pictures in instructions (ThermoFisher website), or if the batch had been used for 3 months, whichever coming first, but were not in particular tested for mycoplasma contamination. Cells for electrophysiology recording were plated on 12 mm round coverslip (Warner Instruments, Hamden, CT) during the passaging procedure. Transient transfection was performed with Lipofectamine 2000 (ThermoFisher Scientific, Waltham, MA) 2 days before recording. The cDNA constructs for wild-type TMEM16F-mCherry, the mutants Q559K-, G615A-, G614A-, I612A-, G614_G615A-, N620A-, and E667Q- mCherry were stably transfected in HEK293 cells as previously reported (Han et al., 2019). Briefly, the cDNAs were subcloned into pENTR1A (Addgene plasmid #17398) and transferred to pLenti CMV Hygro DEST (Addgene plasmid #17454) using Gateway cloning (Campeau et al., 2009). pENTR1A no ccDB (w48-1) and pLenti CMV Hygro DEST (w117-1) were gifts from Dr. Eric Campeau and Dr. Paul Kaufman (University of Massachusetts Medical School, Worcester, MA, USA). TMEM16F-mCherry pLenti was co-transfected into HEK293FT cells with packaging plasmids pMD.2G and psPAX2, which were gifts from Didier Trono (Addgene plasmids # 12259 and #12260). Lentivirus was harvested from the transfected cells 36–48 hr post-transfection and incubated with HEK293 cells to establish stable cell lines under hygromycin selection.
Solutions
For all electrophysiology recordings, bath solution contained 145 mM NaCl, 10 mM HEPES, 2 mM CaCl2, 1 mM MgCl2, 10 mM glucose, pH 7.2 with NaOH. For inside-out recordings, pipette solution contained 150 mM NaCl, 10 mM HEPES, 1 mM CaCl2, unless otherwise stated. The membrane patch was excised to form inside-out configuration in Ca2+-free solution: 150 mM NaCl, 10 mM HEPES, 2 mM EGTA. For solutions with Ca2+ < 100 µM, Ca2+ was added to solutions containing 2 mM EGTA or 2 mM HEDTA, and the final Ca2+ concentration was confirmed with Fluo-3 or Oregon Green BAPTA 5N (ThermoFisher Scientific). For whole-cell recording, the pipette solution contained 150 mM NaCl, 10 mM HEPES, 5 mM HEDTA and 4.1 mM CaCl2, and the final free Ca2+ concentration (15 µM) was confirmed with Oregon Green BAPTA 5N. The osmolality of each solution was adjusted to 290 ~ 310 mOsm/kg.
Solutions with NaCl lower than 150 mM were made by mixing the above solutions (if Ca2+ < 100 µM) with isotonic mannitol solution (300 mM mannitol, 10 HEPES), NMDG-MES solution (150 mM NMDG, 150 mM MES, 10 mM HEPES), or NMDG2-SO4 solution (150 mM NMDG, 75 mM H2SO4, 10 mM HEPES). Ca2+ concentration was subsequently confirmed with Oregon Green BAPTA 5N. The 1 mM Ca2+ solutions were made by directly mixing the solution containing 150 mM NaCl, 10 HEPES with the respective isotonic solutions, and Ca2+ was added at the end. Solutions in Figure 6F contained 150 mM NaI, Na-gluconate or NMDG-Cl with 10 mM HEPES, 1 mM Ca2+. Zn2+ and Gd3+ were directly added to solution of 150 mM NaCl, 10 HEPES from 100 mM stocks. The pH values of all the solutions were confirmed to be ~7.3. All the chemicals were purchased from Sigma-Aldrich (St Louis, MO).
Electrophysiology
Request a detailed protocolCells were lifted with trypsin-EDTA (Life Technologies, Carlsbad, CA) and plated onto 12 mM coverslip (Warner Instruments, Hamden, CT) 3 ~ 4 days before recording. For recording, coverslips with cells were transferred to a chamber on a Nikon-TE2000 Inverted Scope (Nikon Instruments, Melville, NY) and transfection was confirmed with fluorescent microscopy. Patch borosilicate pipets (Sutter Instrument, Novato, CA) were pulled from a Sutter P-97 puller with resistances of 2–3 MΩ for inside-out patch recordings. Solutions were puffed to the excised patch using VC3-8xP pressurized perfusion system (ALA Science, Farmingdale, NY). Data were acquired using a Multiclamp 700B amplifier controlled by Clampex 10.2 via Digidata 1440A (Axon Instruments, Sunnyvale, CA). All experiments were performed at room temperature (22–24°C).
For measurements of reversal potentials, a 3 M KCl salt bridge was used. For experiments with 150 mM NaCl in the pipette solution, based on prediction by Clampex (Molecular Devices, Sunnyvale, CA), the liquid junction potentials for 15 mM NaCl, 45 mM NaCl (balanced with mannitol) were −1.7 mV and −1.0 mV respectively and were only corrected for calculation of permeability ratios (PNa+/PCl-). For recordings with 150 mM NaCl pipette solution, the liquid junction potentials for all the other conditions were within ±2 mV, and were not corrected in the figures. For recordings where the pipette solution contained 15 mM NaCl (balanced with mannitol, NMDG-MES or NMDG2-SO4), the liquid junction potentials were corrected by adding 12 mV, 6 mV and 2 mV, respectively, to the measured values, as estimated by Clampex. The data in Figure 6—figure supplement 2B~F were displayed with the correction of liquid junction potentials.
Data analysis
Request a detailed protocolAll data were analyzed using pClamp10 (RRID: SCR_011323, Molecular Devices, Sunnyvale, CA), OriginLab (OriginLab Corporation, Northampton, MA), and Graphpad Prism (RRID:SCR_002798, GraphPad Software, La Jolla, CA). For the measurement of Ca2+-sensitivity, every trace was fitted to the Hill equation to generate its respective EC50 and H (Hill coefficient). The curves in the figures display the averaged current magnitudes normalized to their respective maximal values (I/Imax %). PNa+/PCl- values were calculated with the simplified equation derived from Goldman-Hodgkin-Katz equation:
where [Na+]o, [Cl-]o, [Na+]i, and [Cl-]i are extracellular and intracellular Na+ and Cl− concentrations, respectively.
Significant differences were determined with Student’s t-test and ANOVA unless otherwise stated. In all cases, data represent mean ± SEM, *p<0.05, **p<0.01, ***p<0.001, ****p<0.0001, n.s. p>0.05.
Data availability
All data generated or analysed during this study are included in the manuscript and supporting files.
References
-
Salting out the ionic selectivity of a wide channel: the asymmetry of OmpFBiophysical Journal 87:943–957.https://doi.org/10.1529/biophysj.104/043414
-
Phosphatidylinositol 4,5-bisphosphate, cholesterol, and fatty acids modulate the calcium-activated chloride channel TMEM16A (ANO1)Biochimica Et Biophysica Acta (BBA) - Molecular and Cell Biology of Lipids 1863:299–312.https://doi.org/10.1016/j.bbalip.2017.12.009
-
Known structures and unknown mechanisms of TMEM16 scramblases and channelsThe Journal of General Physiology 180:jgp.201711957.https://doi.org/10.1085/jgp.201711957
-
TMEM16F (Anoctamin 6), an anion channel of delayed Ca2+ activationThe Journal of General Physiology 141:585–600.https://doi.org/10.1085/jgp.201210861
-
Scramblase TMEM16F terminates T cell receptor signaling to restrict T cell exhaustionThe Journal of Experimental Medicine 213:2759–2772.https://doi.org/10.1084/jem.20160612
-
Independent activation of distinct pores in dimeric TMEM16A channelsThe Journal of General Physiology 148:393–404.https://doi.org/10.1085/jgp.201611651
-
Ligand-gated ion channels: mechanisms underlying ion selectivityProgress in Biophysics and Molecular Biology 86:161–204.https://doi.org/10.1016/j.pbiomolbio.2003.09.002
-
An inner activation gate controls TMEM16F phospholipid scramblingNature Communications 10:e1846.https://doi.org/10.1038/s41467-019-09778-7
-
Drosophila subdued is a moonlighting transmembrane protein 16 (TMEM16) that transports ions and phospholipidsJournal of Biological Chemistry 294:4529–4537.https://doi.org/10.1074/jbc.AC118.006530
-
The nhTMEM16 scramblase is also a nonselective ion channelBiophysical Journal 111:1919–1924.https://doi.org/10.1016/j.bpj.2016.09.032
-
Physical basis of apparent pore dilation of ATP-activated P2X receptor channelsNature Neuroscience 18:1577–1583.https://doi.org/10.1038/nn.4120
-
Independent activation of ion conduction pores in the double-barreled calcium-activated chloride channel TMEM16AThe Journal of General Physiology 148:375–392.https://doi.org/10.1085/jgp.201611650
-
Ca2+-dependent phospholipid scrambling by a reconstituted TMEM16 ion channelNature Communications 4:2367.https://doi.org/10.1038/ncomms3367
-
Comparison of ion transport determinants between a TMEM16 chloride channel and phospholipid scramblaseThe Journal of General Physiology 10:jgp.201812270.https://doi.org/10.1085/jgp.201812270
-
Structure and function of TMEM16 proteins (anoctamins)Physiological Reviews 94:419–459.https://doi.org/10.1152/physrev.00039.2011
-
Ion channel and lipid scramblase activity associated with expression of TMEM16F/ANO6 isoformsThe Journal of Physiology 593:3829–3848.https://doi.org/10.1113/JP270691
-
TMEM16F is a component of a Ca2+-activated cl- channel but not a volume-sensitive outwardly rectifying cl- channelAmerican Journal of Physiology. Cell Physiology 304:C748–C759.https://doi.org/10.1152/ajpcell.00228.2012
-
Role of membrane potential in the regulation of cell proliferation and differentiationStem Cell Reviews and Reports 5:231–246.https://doi.org/10.1007/s12015-009-9080-2
-
Calcium-dependent phospholipid scramblase activity of TMEM16 protein family membersJournal of Biological Chemistry 288:13305–13316.https://doi.org/10.1074/jbc.M113.457937
-
Anoctamins are a family of Ca2+-activated cl- channelsJournal of Cell Science 125:4991–4998.https://doi.org/10.1242/jcs.109553
-
Anoctamin 5/TMEM16E facilitates muscle precursor cell fusionThe Journal of General Physiology 150:1498–1509.https://doi.org/10.1085/jgp.201812097
-
Anoctamins/TMEM16 proteins: chloride channels flirting with lipids and extracellular vesiclesAnnual Review of Physiology 79:119–143.https://doi.org/10.1146/annurev-physiol-022516-034031
-
Calcium-calmodulin does not alter the anion permeability of the mouse TMEM16A calcium-activated chloride channelThe Journal of General Physiology 144:115–124.https://doi.org/10.1085/jgp.201411179
Article and author information
Author details
Funding
National Institute of Neurological Disorders and Stroke (R01NS069229)
- Lily Yeh Jan
Jane Coffin Childs Memorial Fund for Medical Research
- Tina W Han
Eunice Kennedy Shriver National Institute of Child Health and Human Development (F32HD089639)
- Mu He
Howard Hughes Medical Institute
- Yuh Nung Jan
- Lily Yeh Jan
The funders had no role in study design, data collection and interpretation, or the decision to submit the work for publication.
Acknowledgements
We thank Dr. Christian Peters (University of Illinois, Chicago, IL) and Dr. Huanghe Yang (Duke University, Durham, NC) for their critical reading of the manuscript and for helpful discussions. This study is supported in part by NIH Grants R01NS069229 (to LYJ), F32HD089639 (to MH) and by a grant from The Jane Coffin Childs Memorial Fund for Medical Research (to TWH). YNJ and LYJ are Howard Hughes Medical Institute investigators.
Copyright
© 2019, Ye et al.
This article is distributed under the terms of the Creative Commons Attribution License, which permits unrestricted use and redistribution provided that the original author and source are credited.
Metrics
-
- 3,053
- views
-
- 489
- downloads
-
- 27
- citations
Views, downloads and citations are aggregated across all versions of this paper published by eLife.
Citations by DOI
-
- 27
- citations for umbrella DOI https://doi.org/10.7554/eLife.45187