Multiplex CRISPR/Cas screen in regenerating haploid limbs of chimeric Axolotls
Abstract
Axolotls and other salamanders can regenerate entire limbs after amputation as adults, and much recent effort has sought to identify the molecular programs controlling this process. While targeted mutagenesis approaches like CRISPR/Cas9 now permit gene-level investigation of these mechanisms, genetic screening in the axolotl requires an extensive commitment of time and space. Previously, we quantified CRISPR/Cas9-generated mutations in the limbs of mosaic mutant axolotls before and after regeneration and found that the regenerated limb is a highfidelity replicate of the original limb (Flowers et al. 2017). Here, we circumvent aforementioned genetic screening limitations and present methods for a multiplex CRISPR/Cas9 haploid screen in chimeric axolotls (MuCHaChA), which is a novel platform for haploid genetic screening in animals to identify genes essential for limb regeneration.
Introduction
Salamanders are the only vertebrates known to regenerate complete limbs as adults. The axolotl, a species of salamander, can regenerate limbs, tails, and gills without scarring. Regeneration of these complex structures occurs through the formation of a blastema, a mass of proliferating dedifferentiated cells and pre-existing progenitor and stem cells (Currie et al., 2016; Kragl et al., 2009; Sandoval-Guzmán et al., 2014). Transcriptional profiling of the limb blastema has produced long lists of candidate genes that, to date, remain largely uncharacterized (Bryant et al., 2017; Campbell et al., 2011; Gerber et al., 2018; Knapp et al., 2013; Leigh et al., 2018; Monaghan et al., 2009; Voss et al., 2015).
The advent of CRISPR/Cas9 has made the axolotl a genetically tractable organism and the functional interrogation of these genes possible (Flowers et al., 2014; Fei et al., 2014). Although near-complete knockout F0 animals can be generated, they appear to be universally mosaic, harboring a variety of mutant alleles (Flowers et al., 2014). Such embryonically generated mutations both perturb the function of the targeted gene and uniquely label affected cell lineages with a traceable genetic barcode. Previously, we measured the fidelity of limb regeneration by using next-generation sequencing (NGS) to quantify the mutant allele frequencies of multiple genomic loci before and after limb regeneration in mosaic mutant axolotls (Flowers et al., 2017). We found that the majority of very low-frequency alleles reoccur in a regenerated limb at a frequency strikingly similar to that of the original limb. These data indicate that limb regeneration is a high-fidelity process in which the contributions of small cell populations to the original limb are replicated in the regenerated limb (Figure 1C,D).
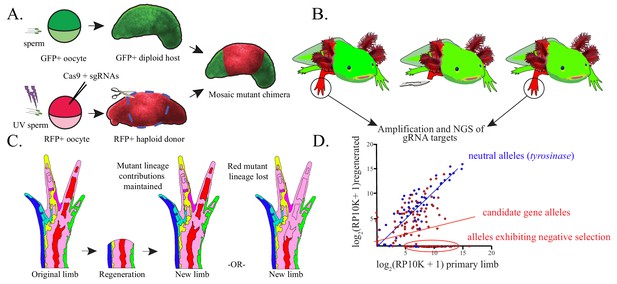
Haploid-diploid chimeric generation and lineage analysis.
(A) Schematic of haploid-diploid chimera generation. Gynogenetic haploids are generated by in vitro activation of unfertilized eggs with UV-enucleated sperm and subsequently mutagenized using CRISPR/Cas9. Chimeric embryos are generated by replacing the limb buds of GFP+ diploid embryos with corresponding tissue from mutagenized haploid donors. (B) DNA is extracted from original and fully regenerated haploid limbs of juvenile chimeric axolotls, target sequences are PCR amplified, and these products are subjected to NGS. (C) Schematic depicting the contribution of mutant cell lineages to the original and regenerated limb. Cell lineages mutant for non-essential candidate genes (light blue, dark blue, yellow, purple, red, green) may participate normally in regeneration and therefore contribute to the regenerated limb and original limb in a similar proportion. Cell lineages harboring deleterious mutant alleles deleterious (red, far right) are predicted to be reduced in regenerated limbs. (D) A hypothetical linear regression plot of the log2 of reads per ten thousand (RP10K+1) of mutant alleles before and after regeneration. Mutant alleles of a neutral gene, tyrosinase (blue), are faithfully preserved between original and regenerated limbs. Mutagenized genes essential for regeneration (red) will show a decrease in allele frequency or a complete loss of alleles in the regenerated limb.
Recent single-cell sequencing of the axolotl limb blastema demonstrated that cell identities converge at a transcriptional level during regeneration (Gerber et al., 2018). This suggests a shared genetic program across most blastemal cells. We anticipated that genetic perturbation of critical blastema-enriched genes would impair mutagenized cells’ ability to participate in the regenerative process. Negative selection screens are widely used to identify genes essential for cellular processes with CRISPR/Cas (Shalem et al., 2014; Wang et al., 2014; Yin and Chen, 2017). Screening can be improved by using haploid cells, which harbor a single copy of each gene, and thus require monoallelic inactivation to unveil loss-of-function phenotypes. We sought to determine whether we could detect negative selection of mutant alleles in regenerated haploid limbs of axolotls. (Figure 1C,D).
Results
We generated gynogenetic haploids through in vitro activation of eggs from white or transgenic RFP+ females using UV-enucleated sperm from a transgenic GFP+ male (Figure 1A,B). Haploidy was confirmed by karyotype (n = 14, 3/3 embryos, three squashes/embryo, Figure 2—figure supplement 1A), the universal appearance of the haploid syndrome embryonic phenotype (120/120 embryos, Figure 2—figure supplement 1B,C; Hronowski et al., 1979), and complete absence of paternally-derived GFP expression in donor embryos (156/156 GFP-, Figure 2—figure supplement 1B). Adult haploid axolotls are not viable, so we developed reliable whole limb bud grafting techniques to generate chimeric axolotls with haploid limbs (Figure 1A, Figure 2—figure supplement 1D). To find the optimal embryonic stage for limb bud grafting, we performed reciprocal grafts between stage-matched white and GFP+ diploid embryos across a range of developmental stages (Figure 2—source data 1). Diploid-diploid chimera (DDC) graft limbs were scored for the presence or absence of GFP+ host-derived cells using a fluorescent microscope. Embryos grafted at stage 23–25 produced normally developed limbs with a consistent host-derived neural GFP+ expression pattern (Figure 2B; Figure 2—source data 1). We adapted the DDC grafting protocol for haploids by substituting diploid tissue with that of haploid donors. We found that cleanly grafted haploid limbs develop fully, but are smaller and shorter than the opposing diploid limbs of the same animals (Figure 2A, Figure 2—figure supplement 2). Furthermore, haploid-diploid chimeras (HDCs) exhibited a neural-GFP expression pattern similar to DDCs (Figure 2B).
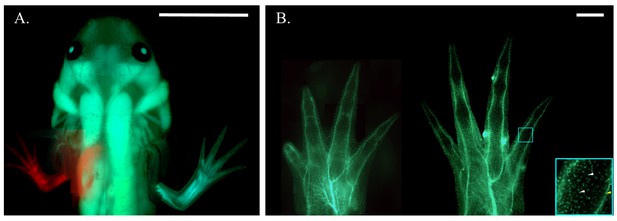
Haploid-diploid chimeric axolotl.
(A) Composite fluorescent image of a chimeric axolotl produced from a limb bud graft from an RFP+ haploid embryo to a GFP+ diploid host. Scale bar = 1 cm. (B) Composite fluorescent image of haploid (left) and diploid (right) limbs produced by embryonic limb bud grafting from a white donor embryo to a GFP+ diploid host. Both the GFP- haploid limb and GFP- diploid limb grafted to a GFP+ diploid host exhibit a GFP expression pattern that appears to be restricted to spinal nerves innervating the limb (yellow arrow) and individual sensory neurons and blood-derived cells (white arrows) stemming from the host body. Blue box is at 4x magnification (bottom right). Scale bars = 1 mm. Composite images were generated by manually compiling individual photos. Images have been adjusted with cropping, contrast, color correction, and gamma correction.
-
Figure 2—source data 1
The number of diploid white to diploid GFP+ grafts that were performed to determine the optimal embryonic stage for limb bud grafting.
- https://cdn.elifesciences.org/articles/48511/elife-48511-fig2-data1-v1.xlsx
Next, we tested the regenerative capacity of HDC and DDC graft limbs. We amputated HDC and DDC limbs and found that both fully regenerate and retain their neural GFP expression pattern (2/2 HDC limbs, 2/2 DDC limbs). While the gross morphology of regenerated haploid limbs is identical to that of the original limbs, haploid limb regeneration is slightly delayed relative to diploid limb regeneration (Figure 2—figure supplement 2). To quantify the fidelity of haploid limb regeneration, we generated HDCs using haploid donors mutagenized at one of two genomic loci non-essential for regeneration, tyrosinase and methyltransferase-like, for which we had previously observed faithful recapitulation of mutant allele frequencies between original and regenerated diploid limbs. NGS of targeted loci in 12 HDCs mutagenized with one of two highly active guide RNAs (gRNAs) revealed 92 total alleles with a mean mutation frequency of 3.46% per allele in the primary limbs (SE = + /- 1.19%). NGS of these targeted sites in DNA from regenerated limbs revealed that the log score of the normalized read numbers for each allele in the primary limbs predicts the log score of the normalized read numbers in the secondary limbs (R2 = 0.544, p<0.0001, Figure 3A,B, Figure 3—figure supplement 1), which is similar to observations made with these same targets in diploid mosaic limbs (Flowers et al., 2017). Thus, with respect to morphology and cell lineage contributions, haploid limb regeneration is similar to that of diploid limb regeneration.
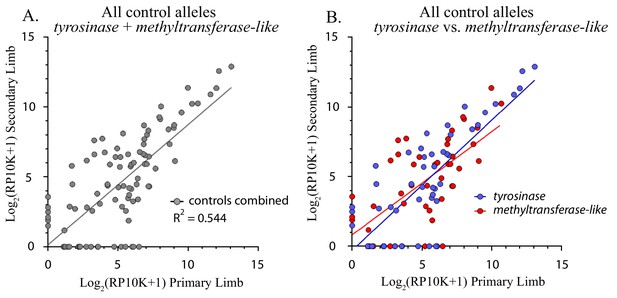
Control alleles.
(A) Comparison of all alleles generated in the controls (methyltransferase plus tyrosinase) in the original and regenerated haploid limbs of 12 animals. The log scores of the reads per ten thousand (RP10K) of every allele in the original limb are significantly correlated with those of the secondary limb (R2 = 0.544, p-value<0.0001). (B) Linear regression comparing the log scores of RP10K for alleles depicted in 3A, but separated by gene (methyltransferase-like in red and tyrosinase in blue). The slopes of the regression lines are not significantly different for the two genes (methyltransferase-like m = 0.740, tyrosinase m = 0.935, p-value=0.238, ANCOVA).
The majority of tyrosinase and methyltransferase-like alleles (76.1%, 70/92) are detected in both the first and second haploid limbs. Most mutant alleles occur at a low frequency, comprising fewer than 1.6% of the total reads for a given haploid limb (81.5%, 75/92, Table 1). The majority of low-frequency alleles are detected in both primary and secondary limbs (70.7%, 53/75) and undergo less than a two-fold change in frequency after regeneration (69.3%, 52/75, Table 1, Figure 3—figure supplement 2A–D). Collectively, these results support the notion that, as in diploids, haploid limb regeneration is a high-fidelity process in which the majority of small cell lineages contribute to the regenerated limb in a manner similar to their contributions to the original developed limb.
The numbers of all alleles in the first limbs of controls, all targets, fetuin-b, all targets excluding fetuin-b, catalase, and all targets excluding catalase that are sorted by mutation frequency and log of fold change.
Controls | All targets | |||||||
---|---|---|---|---|---|---|---|---|
Allele Frequency | Log of fold change | Allele Frequency | Log of fold change | |||||
(Low) Frequency < 1.6% | < 2 | > 2 | (Low) Frequency < 1.6% | < 2 | > 2 | |||
Alleles Lost | 22 | 5 | 17 | Alleles Lost | 60 | 24 | 36 | |
Alleles Preserved | 53 | 35 | 18 | Alleles Preserved | 94 | 71 | 23 | |
Sum | 75 | 40 | 35 | Sum | 154 | 95 | 59 | |
Allele Frequency | Log of fold change | Allele Frequency | Log of fold change | |||||
Frequency > 1.6% | < 2 | > 2 | Frequency > 1.6% | < 2 | > 2 | |||
Alleles Lost | 0 | 0 | 0 | Alleles Lost | 2 | 0 | 2 | |
Alleles Preserved | 17 | 15 | 2 | Alleles Preserved | 20 | 13 | 7 | |
Sum | 17 | 15 | 2 | Sum | 22 | 13 | 9 | |
Total alleles: 92 | Total alleles: 176 | |||||||
fetuin-b | All targets except fetuin-b | |||||||
Allele Frequency | Log of fold change | Allele Frequency | Log of fold change | |||||
(Low) Frequency < 1.6% | < 2 | > 2 | (Low) Frequency < 1.6% | < 2 | > 2 | |||
Alleles Lost | 20 | 9 | 11 | Alleles Lost | 40 | 15 | 25 | |
Alleles Preserved | 25 | 21 | 4 | Alleles Preserved | 69 | 50 | 19 | |
Sum | 45 | 30 | 15 | Sum | 109 | 65 | 44 | |
Allele Frequency | Log of fold change | Allele Frequency | Log of fold change | |||||
Frequency > 1.6% | < 2 | > 2 | Frequency > 1.6% | < 2 | > 2 | |||
Alleles Lost | 2 | 0 | 2 | Alleles Lost | 0 | 0 | 0 | |
Alleles Preserved | 1 | 0 | 1 | Alleles Preserved | 19 | 13 | 6 | |
Sum | 3 | 0 | 3 | Sum | 19 | 13 | 6 | |
Total alleles: 48 | Total alleles: 128 | |||||||
catalase | All other targets except catalase | |||||||
Allele Frequency | Log of fold change | Allele Frequency | Log of fold change | |||||
(Low) Frequency < 1.6% | < 2 | > 2 | (Low) Frequency < 1.6% | < 2 | > 2 | |||
Alleles Lost | 6 | 1 | 5 | Alleles Lost | 54 | 23 | 31 | |
Alleles Preserved | 1 | 1 | 0 | Alleles Preserved | 93 | 70 | 23 | |
Sum | 7 | 2 | 5 | Sum | 147 | 93 | 54 | |
Allele Frequency | Log of fold change | Allele Frequency | Log of fold change | |||||
Frequency > 1.6% | < 2 | > 2 | Frequency > 1.6% | < 2 | > 2 | |||
Alleles Lost | 0 | 0 | 0 | Alleles Lost | 2 | 0 | 2 | |
Alleles Preserved | 1 | 0 | 1 | Alleles Preserved | 19 | 13 | 6 | |
Sum | 1 | 0 | 1 | Sum | 21 | 13 | 8 | |
Total alleles: 8 | Total alleles: 168 |
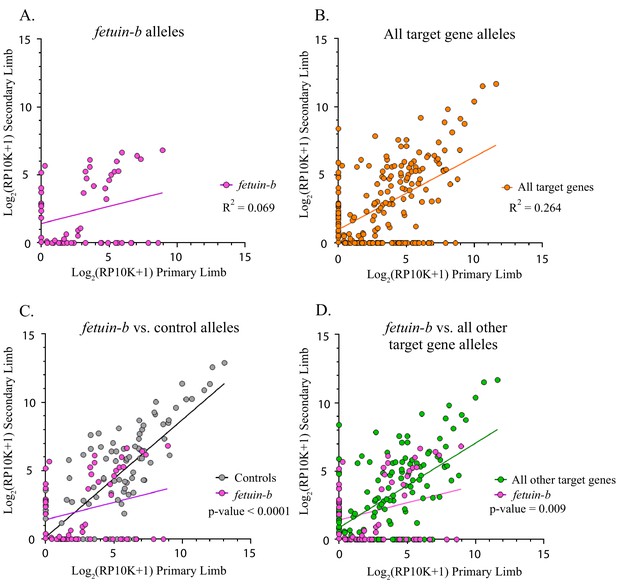
Fetuin-b alleles compared to all other target gene and control alleles.
(A) Linear regression plot of the log2(RP10K) score for all alleles of fetuin-b detected in the first and regenerated haploid limbs of 11 animals. The log scores of alleles in the primary limb poorly predict the log scores of alleles in the secondary limb. (R2 = 0.069, p-value=0.046). (B) Linear regression plot of the log2(RP10K) score for all alleles of all targets detected in the primary and regenerated limb (R2 = 0.264, p<0.0001). (C) Comparison of linear regression plots of fetuin-b (pink) with controls (gray). The slopes of the regression lines are significantly different (fetuin-b m = 0.254, controls m = 0.861, p-value<0.0001, ANCOVA). (D) Comparison of linear regression plots of fetuin-b (pink) with all other targets (green). The slopes of the regression lines are significantly different (fetuin-b m = 0.254, all other targets m = 0.619, p-value=0.009, ANCOVA).
-
Figure 4—source data 1
Raw number of reads, normalized reads, and log2(RP10K) score for all mutant alleles of every targeted gene in each mutant limb in this study.
- https://cdn.elifesciences.org/articles/48511/elife-48511-fig4-data1-v1.xlsx
We found two genes, fetuin-b and catalase, that exhibited signs of negative selection, showing both a loss of mutant alleles and a decline in the contribution of mutant alleles from primary to secondary limbs (Table 1). We compared the linear regression line slopes of all mutant alleles between primary and secondary limbs for each target gene with those of the inessential controls (methyltransferase-like and tyrosinase) and found that fetuin-b (fetub) was significantly different (n = 48 mutant alleles, fetub m = 0.254, controls m = 0.861, p<0.0001, Figure 4A,C). Further comparison of fetub with all other target genes combined reveals that the slope of the linear regression of fetub is lower than that of all other target genes combined (fetub m = 0.254, All other target genes m = 0.619, p=0.009, ANCOVA, Figure 4D). Linear regression analysis of fetub reveals that the log scores of the normalized read numbers for each allele in the second limb poorly predict the log scores of the normalized read numbers in the primary limb (R2 = 0.069, p=0.046, Figure 4A). Alleles of fetub detected in the primary limb are more likely to be absent in the secondary limb (45.8%, 22/48) than alleles detected in controls (23.9%, 22/92) and this difference is significant (χ2 = 7.03, p=0.008). Fetub alleles (45.8%, 22/48) are more likely to be absent from the second limb than alleles of all other targets combined (31.3%, 40/128), but this effect is not significant, except when the other outlier, catalase, is excluded (χ2 = 3.25, p=0.071 and χ2 = 5.23, p=0.022, respectively).
Similarly, the slope of the linear regression of catalase alleles differed from control genes (n = 8 mutant alleles, catalase m = 0.018, controls m = 0.861, p=0.005, ANCOVA, Figure 5A,C). The slope of the linear regression of catalase did not differ from that of all other target genes combined, except when fetub was excluded (catalase m = 0.018, all other target genes m = 0.550, p=0.073, all other target genes excluding fetub m = 0.645, p=0.029, ANCOVA, Figure 5B,D). A significantly greater proportion of catalase alleles are lost (75.5%, 6/8) than both those of controls and all other targets combined (χ2 = 9.53, p=0.002 and χ2 = 5.81, p=0.016, respectively).
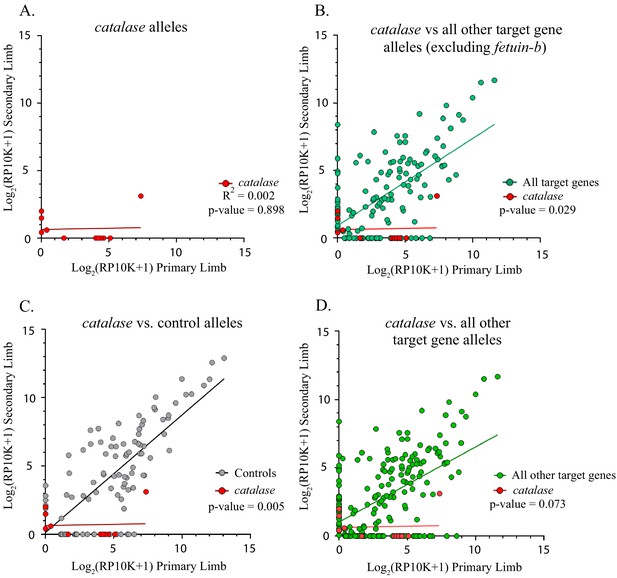
Catalase alleles compared to all other target gene and control alleles.
(A) Linear regression plot of the log2(RP10K) score for all alleles of catalase detected in the first and regenerated haploid limbs of three animals. The log scores of alleles in the primary limbs do not predict the log scores of alleles in the secondary limbs. (R2 = 0.002, p-value=0.898). (B) Comparison of linear regression plots of catalase (red) with all other targets excluding fetuin-b (teal). The slopes of the regression lines are significantly different (catalase m = 0.018, all other targets excluding fetuin-b m = 0.645, p-value=0.029, ANCOVA). (C) Comparison of linear regression plots of catalase (red) with controls (gray). The slopes of the regression lines are significantly different (catalase m = 0.018, controls m = 0.861, p-value=0.005). (D) Comparison of linear regression plots of catalase (red) with all other targets (green). The slopes of the regression lines are not significantly different (catalase m = 0.018, all other targets m = 0.550, p-value=0.073, ANCOVA).
To increase the total number of catalase and fetub mutants analyzed, we next addressed whether loss of these genes perturbs regeneration at a whole organismal level. We produced early embryonic mutants for catalase, fetub, and tyrosinase by injecting gRNAs against each with Cas9 protein into zygotes. At stage 44, we amputated the posterior 2 mm of the tails of each larva and monitored its regeneration. We extracted DNA from the amputated tails and confirmed the high-level mutagenesis of fetub and catalase by fluorescent PCR fragment analysis (fetub, n = 12, mean = 7.3% wildtype alleles, SD = + /- 5.2%;. catalase, n = 16, mean = 3.0% wildtype alleles, SD = + /- 5.8%, Figure 6—source data 1). fetub and catalase mutants did not display regeneration growth defects compared to tyrosinase mutants at early time points, but the total regenerative outgrowth of both fetub and catalase mutant tails were reduced compared to tyrosinase mutants at 18 days post-amputation (n = 16 tyrosinase mutants, p=0.002, fetub; p=0.012, catalase; Welch’s t-test, one-tailed; Figure 6A–C), with the reduction in regeneration also evident at 14 days post-amputation in catalase mutant tails (p=0. 025, Welch’s t-test, one-tailed, Figure 6A). These data indicate that, while catalase and fetub are not essential for the onset of regeneration, the process of regeneration is slower in the tails of catalase and fetub mutants. These findings are consistent with the apparent loss of catalase and fetub mutant cells within the context of regenerating mosaic mutant haploid limbs and suggest a broader role for these genes in the regeneration of multiple tissues and structures. As cell competition in developing tissues can result in the elimination of cells lacking genes controlling the rate of growth at a whole organismal level (Johnston et al., 1999; Morata and Ripoll, 1975), these findings support the validity of this assay as a means to identify genes critical for proper regeneration.
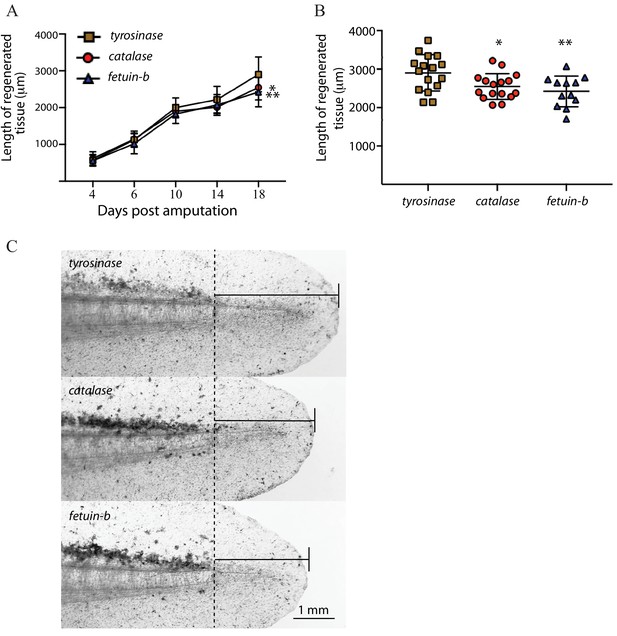
Larval tail regeneration in tyrosinase, catalase, and fetuin-b mutants.
(A) Regenerative outgrowth of tail in high-level tyrosinase, catalase, and fetuin-b F0 mutants. While no significant difference is detected at early time points, both fetuin-b and catalase mutants display tail reduced tail regeneration compared to tyrosinase mutants at later time points (catalase vs tyrosinase, Day 4, p=0.205, Day 6, p=0.400, Day 10, p=0.111. Day 14, p=0.026, Day 18, p=0.011; fetuin-b vs tyrosinase, Day 4, p=0.450, Day 6, p=0.129, Day 10, p=0.047, Day 14, p=0.109, Day 18, 0 = 0.002, Welch’s t-test). Bars indicate standard deviation. (B) Plots of lengths of regenerate in individual tyrosinase, catalase, and fetuin-b F0 mutants at 18 days post-amputation; **=fetuin b, p=0.002, *=catalase, p=0.011. (C) Brightfield images of individual tyrosinase, catalase, and fetuin-b F0 mutants at 18 days post-amputation (dpa) showing median amount of tail regeneration at 18 dpa. Dotted line indicates the amputation plane.
-
Figure 6—source data 1
Regenerative outgrowth measurements and genotyping data for tyrosinase, catalase, and fetuin-b F0 mutants.
- https://cdn.elifesciences.org/articles/48511/elife-48511-fig6-data1-v1.xlsx
Discussion
Collectively, our data suggests that cells lacking the limb blastema-enriched genes, fetub and catalase, have a reduced capacity to contribute to the regenerating limb. Catalase is an enzyme that plays a conserved role in protecting cells from oxidative damage by catalyzing the decomposition of hydrogen peroxide, a reactive oxygen species (ROS). Despite their potentially harmful effects, ROS are critical for normal tail, fin, and heart regeneration to proceed in xenopus and zebrafish (Love et al., 2013; Gauron et al., 2013; Han et al., 2014). However, prolonged ROS-exposure and ROS-induced cellular senescence impair tissue regeneration (Saxena et al., 2019). Overexpression of catalase impedes heart regeneration after infarction in zebrafish, and chemical inhibition of Catalase may transiently delay tail regeneration in xenopus larva, suggesting that ROS levels must be carefully regulated during regeneration (Han et al., 2014; von HAHN, 1959).
Fetuin-B and its paralogue Fetuin-A, are highly expressed in the liver, where they are secreted into the blood plasma, and are also expressed in the chondrocytes and muscle cells of developing limb buds in mouse, rat, and sheep (Terkelsen et al., 1998; Saunders et al., 1994; Dziegielewska et al., 1996; Denecke et al., 2003). Mammalian Fetuins belong to the cystatin superfamily of proteins, which include many protease inhibitors, yet these two proteins appear to have differing biochemical activities (Denecke et al., 2003; Karmilin et al., 2019). Fetuin-A is expressed in the growth plate chondrocytes of young mice and is required for proper long bone development, and Fetuin-A knockout mice exhibit severely foreshortened femora due to growth plate deformations and displaced distal epiphyses (Seto et al., 2012). Fetub, knockout mice, however, do not display these defects, and instead show female infertility (Dietzel et al., 2013); and mammalian Fetuin-B, unlike Fetuin-A, appears to function as a specific inhibitor of meprin and ovastacin metalloproteinases (Karmilin et al., 2019). Extracellular matrix remodeling by metalloproteinases is crucial for a variety of processes, including regeneration. Together, our results suggest that locally expressed Fetub is an important regulator of regeneration in the axolotl.
Both genes for which mutant cells exhibited negative selection in this assay are not developmentally essential in mice; however, transcriptional profiling of axolotl limb blastemas across the time course of regeneration indicates that a considerable portion of blastema-enriched genes are known to participate in limb development or cell survival in other organisms (Monaghan et al., 2012; Knapp et al., 2013; Stewart et al., 2013). We anticipate that mosaic loss-of-function of many genes enriched in both the limb bud and limb blastema may result in a depletion of mutant cell lineages prior to limb formation. Thus, mutant alleles of genes required for both limb development and regeneration may not exhibit negative selection in this assay. Exclusion of mutant alleles prior to limb formation may potentially be investigated by comparing allele frequencies from targeted loci in non-grafted tissues in donor haploid embryos to those found within developed limbs arising from tissue grafts from the same embryos. The identification and validation of catalase and fetub in this assay suggests that this method is particularly useful for identifying genes that are not essential for limb development but critical for proper regeneration.
Recent single-cell analyses of blastema cells across the time course of regeneration indicate that connective-tissue-derived blastema cells transcriptionally converge to express a shared set of genes distinct from that expressed in developing limb buds; however, at later stages of regeneration these blastema cells recapitulate transcriptional programs found in developing limbs (Gerber et al., 2018). These findings indicate that the genes controlling early blastema formation do not substantially overlap with those required for proper limb development. There are several known examples demonstrating dissociation between the genetic control of limb of development and regeneration in the axolotl. The long-characterized recessive short-toes allele in axolotls produces animals that develop limbs but display a progressive decline in regenerative capacity (Del Rio-Tsonis et al., 1992; Gassner and Tassava, 1997). Similarly, loss of sox2 in axolotls produces an early spinal cord regeneration defect without perturbing spinal cord development (Fei et al., 2014). Nonetheless, because of the potential confounding effects that may arise in this assay when targeting genes essential for limb development, we largely excluded blastema-enriched genes expected to be required for limb development from the set of targeted genes in this study.
Although this negative selection assay in mosaic haploid mutant limbs led to the identification and subsequent confirmation of catalase and fetub as critical regeneration genes, we failed to detect significant evidence of negative selection of mutant alleles for other assessed candidate genes. For several of these targeted genes, there were insufficient mutant alleles to provide evidence of negative selection (Figure 4—figure supplement 1). The absence of significant negative selection for these targets should not be regarded as evidence that these genes are not critical for limb regeneration. As we conducted multiplex mutagenesis to permit analysis of multiple targets in limbs of an individual animal, we reduced the mass of injected gRNAs from that used in earlier CRISPR mutagenesis studies (Flowers et al., 2017) to prevent confounding effects caused by mutating multiple targets in single-cell lineages. While 16/25 gRNAs used in this study produced mutant alleles in the limb of at least one animal analyzed, we expect that in future studies injecting greater quantities of gRNA will produce more mutant alleles without confounding results.
Here, we provide a novel screening platform that couples targeted mutagenesis and lineage tracing to identify novel regulators of regeneration. This method relies upon the ability to generate chimeric animals that possess mutagenized haploid limbs. We find that the development and regeneration of these haploid limbs is comparable to that of diploid limbs. Using this approach, we find that catalase and fetuin-b are required for cells to participate in limb regeneration and for proper tail regeneration. As the axolotl possesses an impressive capacity to regenerate many parts of its body, future work should explore whether haploid chimeric approaches may be applied to the study the regeneration of these structures. To our knowledge, this is the first example of a true in vivo haploid selection screen conducted in a complex structure of a vertebrate.
Materials and methods
Reagent type (species) or resource | Designation | Source or reference | Identifiers | Additional information |
---|---|---|---|---|
Genetic reagent (Ambystoma mexicanum) | cagg:egfp | Ambystoma Genetic Stock Center (Sobkow et al., 2006) | AGSC Cat# 110A, RRID:AGSC_110A | |
Genetic reagent (Ambystoma mexicanum) | cagg:nls-mcherry | Ambystoma Genetic Stock Center (Kragl et al., 2009) | AGSC Cat# 112A, RRID:AGSC_112A | |
Chemical compound, drug | MS-222 | Western Chemical | ANADA #200–226 | |
Chemical compound, drug | Human chorionic gonadotropin (Chorulon,) | Merck Animal Health | NADA 140–927 | |
Gene (Ambystoma mexicanum) | msx2 | Axolotl transcriptome assembly 3.4 | AMEXTC_0340000067092 | |
Gene (Ambystoma mexicanum) | prmt1 | Axolotl transcriptome assembly 3.4 | AMEXTC_0340000062704 | |
Gene (Ambystoma mexicanum) | myl6 | Axolotl transcriptome assembly 3.4 | AMEXTC_0340000067862 | |
Gene (Ambystoma mexicanum) | fetub | Axolotl transcriptome assembly 3.4 | AMEXTC_0340000227254 | |
Gene (Ambystoma mexicanum) | hoxc8 | Axolotl transcriptome assembly 3.4 | AMEXTC_0340000065333 | |
Gene (Ambystoma mexicanum) | akap8l | Axolotl transcriptome assembly 3.4 | AMEXTC_0340000192860 | |
Gene (Ambystoma mexicanum) | hrnrpa0 | Axolotl transcriptome assembly 3.4 | AMEXTC_0340000081837 | |
Gene (Ambystoma mexicanum) | hsd17b10 | Axolotl transcriptome assembly 3.4 | AMEXTC_0340000257015 | |
Gene (Ambystoma mexicanum) | hoxb9 | Axolotl transcriptome assembly 3.4 | AMEXTC_0340000035333 | |
Gene (Ambystoma mexicanum) | tyrosinase | Axolotl transcriptome assembly 3.4 | AMEXTC_0340000179254 | |
Gene (Ambystoma mexicanum) | etv4 | Axolotl transcriptome assembly 3.4 | AMEXTC_0340000233035 | |
Gene (Ambystoma mexicanum) | cacng1 | Axolotl transcriptome assembly 3.4 | AMEXTC_0340000081988 | |
Gene (Ambystoma mexicanum) | catalase | Axolotl transcriptome assembly 3.4 | AMEXTC_0340000186723 | |
Gene (Ambystoma mexicanum) | hoxb13 | Axolotl transcriptome assembly 3.4 | AMEXTC_0340000007929 | |
Gene (Ambystoma mexicanum) | zic5 | Axolotl transcriptome assembly 3.4 | AMEXTC_0340000057641 | |
Gene (Ambystoma mexicanum) | ecm1 | Axolotl transcriptome assembly 3.4 | AMEXTC_0340000123229 | |
Gene (Ambystoma mexicanum) | cornifelin | Axolotl transcriptome assembly 3.4 | AMEXTC_0340000173184 | |
Gene (Ambystoma mexicanum) | dsg-like | Axolotl transcriptome assembly 3.4 | AMEXTC_0340000056512 | |
Gene (Ambystoma mexicanum) | enpp2 | Axolotl transcriptome assembly 3.4 | AMEXTC_0340000217071 | |
Gene (Ambystoma mexicanum) | fabp2 | Axolotl transcriptome assembly 3.4 | AMEXTC_0340000084459 | |
Gene (Ambystoma mexicanum) | pmp2 | Axolotl transcriptome assembly 3.4 | AMEXTC_0340000238807 | |
Gene (Ambystoma mexicanum) | kcne1 | Axolotl transcriptome assembly 3.4 | AMEXTC_0340000121776 | |
Gene (Ambystoma mexicanum) | krt6a | Axolotl transcriptome assembly 3.4 | AMEXTC_0340000060835 | |
Gene (Ambystoma mexicanum) | rcc1 | Axolotl transcriptome assembly 3.4 | AMEXTC_0340000210022 | |
Recombinant DNA reagent | MLM3613 | (Hwang et al., 2013) | RRID: Addgene plasmid 42251 | Cas9 expression vector |
Peptide, recombinant protein | Cas9 | PNABio | Cat. #: CP04-500 | |
Commercial assay or kit | mMessage mMachine Kit | ThermoFisher | Cat. #: Am1345 | |
Commercial assay or kit | MAXIscript SP6/T7 Transcription Kit | ThermoFisher | Cat. #: Am1322 | |
Chemical compound, drug | MS-222 | Sigma Aldrich | SML1656 | |
Software, algorithm | Geneious Software | Biomatters | RRID:SCR_010519 |
Animals
All animal experiments were carried out on Ambystoma mexicanum (axolotls) in facilities at Yale University. Experimental procedures were approved by the Yale University IACUC (2017–10557) and were in accordance with all federal policies and guidelines governing the use of vertebrate animals. All axolotls used in this study were produced by natural mating or in vitro fertilization and housed in our facility. They were fed artemia, blood worms, and fish pellets. The parental cagg:egfp and cagg:nls-mcherry transgenic animals were originally obtained from the Ambystoma Genetic Stock Center (Kragl et al., 2009; Sobkow et al., 2006).
Haploid generation
Request a detailed protocolGametes were collected in a manner similar to that previously described (Mansour et al., 2011), and haploids were generated using in vitro activation methods (Trottier and Armstrong, 1976). RFP+ or white mutant females were anesthetized in 1 g/L MS-222 (Tricaine-S, Western Chemical) and injected with 1500 units of human chorionic gonadotropin (Chorulon, Merck Animal Health) in the dorsal musculature above the hind limbs. Females were stored at 8°C to 10°C for 2 days until they began laying unfertilized eggs. Gametes were then collected from a GFP+ male by gentle pelvic squeezing using a P1000 micropipette. Sperm viability and concentration was assessed using an inverted microscope. Sperm was diluted to 80,000 motile cells/mL in sterile 0.1x MMR and spread on a sterile petri dish to form a 1-mm deep film. Eggs were extracted from the female after full anesthesia in a similar manner, without the use of a pipette. To enucleate, sperm were placed 4 cm from the bulbs of a 254 nm CL-1000 Ultraviolet Crosslinker (UVP) and irradiated with 800,000 uJ/mm2 of UV energy. Each unfertilized egg was then coated with 0.25 to 0.5 µL of enucleated sperm and allowed to sit at room temperature for 30 min. Eggs were then flooded with sterile 0.1x MMR. Haploid embryos were individually housed and maintained from 10°C to 18°C. Haploids were inspected for GFP expression 3 to 4 days after in vitro activation.
Karyotype analysis
Request a detailed protocolStage 35 embryos selected for karyotype analysis were staged according to the Schreckenberg and Jacobson staging series (Schreckenberg and Jacobson, 1975). Embryos were prepared for karyotype analysis by incubating them in 0.25% colchicine in 0.1x MMR for 48 hr at 18°. Embryos were then washed twice with dissociation solution (0.1x MMR without Ca2+ or Mg2+) with 0.25% Colchicine. Using fine forceps, the ventral half of each embryo was removed and individually transferred to a microcentrifuge tube containing 1 mL of dissociation solution and incubated at room temperature for 5 min. Using a Pasteur pipette, cells were loosened by gentle agitation and allowed to settle. All but 50 µL of the dissociation solution was removed without disturbing the cells and replaced with 950 L of 60% acetic acid in water, gently mixed, and allowed to stand for 5 min. The fixed cells were pipetted onto positively charged slides, which were briefly flamed to dry before a cover slip was added. Without disturbing the coverslip, a 50-lb lead brick with a paper towel cushion was placed on the slide to squash the cells. After 5 min, the brick was removed, the slides frozen on dry ice, and the coverslips were pried off with a scalpel. The samples were then stained with Hoechst 33342, covered, and sealed with clear nail polish.
Genome editing
View detailed protocolTarget gRNA sequences are listed in the Supplementary file 1. Axolotl matings and microinjections were carried out as previously described (Flowers and Crews, 2015) with the following modifications for haploid mutagenesis: Haploids were allowed to develop for 7 hr until they reached a two-cell stage. For multiplex mutagenesis, each blastomere was injected with an equal volume of injection mix for a total of 1000 pg of cas9 mRNA and 5 pg per gRNA (five total). Each gRNA was injected in two separate pools of gRNAs into embryos from two separate matings. Control embryos were injected as described, but with 50 pg of gRNA. The data described represents the results from ten independent in vitro fertilization, injection, and grafting experiments to produce experimental animals, and three additional experiments to produce control animals.
For tail regeneration experiments, embryos were produced by a single mating and injected with 1250 pg of gRNA coupled with 1250 pg of Cas9 protein (PNA Bio) as described previously within 2 hr of being laid (Fei et al., 2018). Successful mutagenesis of tyrosinase was assessed by loss of pigmentation. Mutations of catalase and fetuin-b were confirmed by fragment analysis PCR (Figure 6—source data 1).
Limb field grafting
Request a detailed protocolStage-matched haploid embryos and GFP+ diploid hosts (stages 21–25) were freed from their vitelline membranes and washed with sterile 0.1x MMR with antibiotics and stored overnight at 4°C. The embryos were then transferred to refrigerated, sterile agar plates with holding grooves and maintained at 8°C on a chilled-stage dissecting microscope. Two sets of Dumostar forceps (Dumont #55, Fine Science Tools) were used to replace the limb bud fields from the GFP hosts with the corresponding tissue from haploid donors. Tissue grafts were held in place for one hour using a small rectangular glass shard from a crushed cover slip. The glass shards were then carefully removed, and the embryos were gently transferred to individual housing at 12°C for 24 hr. Afterwards, the embryos were transferred to new sterile 0.1x MMR with antibiotics and maintained at 16°C to 18°C. 0.1x MMR was replaced every day until the tadpoles began to feed. After 2 to 3 months of development, limbs were inspected for purity and quality using a fluorescent dissecting microscope.
Amputations
Request a detailed protocolAmputations were performed through the mid-zeugopod as previously described (Flowers et al., 2017). Animals were anesthetized in 1 g/L MS-222 (Tricaine-S, Western Chemical). For each gRNA target pool, an additional amputation was performed on a non-mutant animal to serve as a non-mutant control for sequencing. Limbs were frozen at −20° C until all primary and secondary limbs were collected.
Tail amputations were carried out in stage 44 larvae (Nye et al., 2003). Larvae were maintained at 19.5°C in 0.1x MMR. Embryos were monitored and imaged with a Zeiss stereomicroscope using brightfield imaging.
Genomic DNA preparation
Request a detailed protocolGenomic DNA was extracted from entire amputated limbs using the DNeasy Blood and Tissue Collection Kit (Qiagen) according to the manufacturer’s protocol with the following modifications: limbs were suspended in a 3x volume and completely digested with vortexing in Proteinase K for 6 to 8 hr at 56°C. Before adding AL buffer and ethanol, the digest was split into three separate tubes of equal volume for each limb and treated as a separate sample. After the addition of AL buffer and ethanol, the sample was vortexed and frozen at −20°C overnight. After thawing, the purification process was completed according to the manufacturer’s instructions. For tail amputations, DNA was extracted from 2 mm tissue samples using the QiaAmp DNA Kit (Qiagen) according to the manufacturer’s instructions.
Target amplification and genotyping
Request a detailed protocolAll PCRs for primary and secondary limbs were performed at the same time using Phusion High-Fidelty DNA Polymerase (NEB). Target sequences were labeled using a three-cycle labeling step with a unique molecular identifier (UMI), purified, and amplified with final 30-cycle PCR using the purified UMI-labeled product as a template. The final PCR product was purified to remove all primers. For the three-cycle UMI labeling step, 500 ng of genomic DNA was used as template in a 100 µL reaction. For each 30-cycle PCR, an indexed universal adaptor forward primer and a gene-specific reverse primer was used. From 5’ to 3’, each UMI consists of a universal adaptor sequence, a 10N randomized barcode, and 18 to 28 bases of gene specific sequence. Each universal adaptor primer possesses a unique 4 to 6 bp 5’ indexing sequence. For each target, this process was carried out for a no-gDNA control. All products were purified using 1.0x Ampure XP magnetic beads (Agencourt). Products were prepared for sequencing using the TruSeq Nano DNA Library Prep Kit and indexed with the Unique Dual Indexes for TruSeq (Illumina). All universal and gene specific primer sequences are listed in Supplementary file 1.
The mutation rate in individual larvae for tail regeneration experiments was determined using fragment analysis of fluorescent PCR products as described previously (Flowers and Crews, 2015) and analyzed using GeneMapper software. (Thermo Fisher Scientific). Animals in which PCR fragments corresponding to the expected size of wildtype PCR products represented more than 15% of the total intensity of the sum of all PCR products were excluded from analysis.
Amplicon library QC
Request a detailed protocolTo identify and size the various amplicon products in each sample, libraries were analyzed on the Agilent TapeStation 4200 (Agilent Technologies) using the DNA D1000 High Sensitivity assay. Target peaks were identified within the range of 150 bp – 500 bp and samples were then processed for upper and lower automated size selection using the Pippin Prep 2% Agarose, dye-free cassette (Sage Science). Size selected eluates were purified using the Qiagen PCR purification kit (Qiagen) and re-analyzed on the Agilent Tapestation DNA D1000 High Sensitivity assay to confirm the removal of products less than 150 bp and greater than 500 bp. Amplicon libraries were then quantified and normalized using the dsDNA High Sensitivity Assay for Qubit 3.0 (Life Technologies).
Illumina MiSeq sequencing
Request a detailed protocolThe sample library pools were prepared for Illumina MiSeq sequencing following the denaturing and dilution protocol set forth by the manufacturer (Illumina). Prior to MiSeq v2 500 cycle sequencing, the prepared pool was first quality checked on a MiSeq Reagent kit v2 Nano to ensure proper representation of each sample (biased pooling percentage). Deep sequencing on the MiSeq Reagent kit v2 500 cycle was performed with paired end 250 bp reads across five sequencing runs.
Sequence analysis
Request a detailed protocolAlleles were quantified as previously described (Flowers et al., 2017). UMI sequences were extracted from each sequence read after allele assignment, and duplicate sequences were eliminated using the ‘eliminate duplicate reads’ function in Geneious R11. Based upon the input molecular weight of DNA used in our initial PCRs, we estimated that the sequence reads for each gene for each limb represents a sample of approximately 10,000 cells. We produced normalization coefficients by dividing 10,000 by the total number of unique assigned reads for each target for each animal. The number of unique reads for each allele was multiplied by this coefficient to produce a reads per 10 k (RP10K) value. To obtain coherent log scores, one was added to each value prior to log calculations. All allele sequences, raw allele counts, normalized read numbers, and log values for every animal for each gene can be found in Figure 4—source data 1.
Statistical analyses
Request a detailed protocolThe log scores of normalized sequence reads for all alleles of control genes and experimental genes were compared between primary and secondary limbs. Linear regressions were created for each target and control gene, and the slope of each experimental gene regression was compared both to that of all control genes and that of all other experimental genes by one-way ANCOVA in GraphPad Prism software. As two genes were found to differ significantly from control genes, the alleles for those genes were excluded from the set of experimental genes, and the slope of each experimental gene regression was compared to the trimmed set of all other experimental genes.
Data availability
All data generated or analyzed during this study are included in the manuscript or supporting files.
References
-
Gene expression profile of the regeneration epithelium during axolotl limb regenerationDevelopmental Dynamics 240:1826–1840.https://doi.org/10.1002/dvdy.22669
-
Fetuin-B, a liver-derived plasma protein is essential for fertilizationDevelopmental Cell 25:106–112.https://doi.org/10.1016/j.devcel.2013.03.001
-
The expression of fetuin in the development and maturation of the hemopoietic and immune systemsHistochemistry and Cell Biology 106:319–330.https://doi.org/10.1007/BF02473242
-
Generating and identifying axolotls with targeted mutations using Cas9 RNA-guided nucleaseMethods in Molecular Biology 1290:279–295.https://doi.org/10.1007/978-1-4939-2495-0_22
-
Development and survival of haploids of the mexican axolotl,Ambystoma mexicanumJournal of Experimental Zoology 209:41–47.https://doi.org/10.1002/jez.1402090105
-
Efficient genome editing in zebrafish using a CRISPR-Cas systemNature Biotechnology 31:227–229.https://doi.org/10.1038/nbt.2501
-
Minutes: mutants of Drosophila autonomously affecting cell division rateDevelopmental Biology 42:211–221.https://doi.org/10.1016/0012-1606(75)90330-9
-
Extending the table of stages of normal development of the axolotl: limb developmentDevelopmental Dynamics : An Official Publication of the American Association of Anatomists 226:555–560.https://doi.org/10.1002/dvdy.10237
-
Expression and distribution of fetuin in the developing sheep fetusHistochemistry 102:457–475.https://doi.org/10.1007/BF00269578
-
Normal stages of development of the axolotl. Ambystoma mexicanumDevelopmental Biology 42:391–399.https://doi.org/10.1016/0012-1606(75)90343-7
-
Rat fetuin: distribution of protein and mRNA in embryonic and neonatal rat tissuesAnatomy and Embryology 197:125–133.https://doi.org/10.1007/s004290050124
-
Simple meets single: the application of CRISPR/Cas9 in haploid embryonic stem cellsStem Cells International 2017:1–6.https://doi.org/10.1155/2017/2601746
Article and author information
Author details
Funding
Connecticut Innovations (Seed Grant 15RMA-YALE-09)
- Grant Parker Flowers
Eunice Kennedy Shriver National Institute of Child Health and Human Development (Individual Postdoctoral Fellowship F32HD086942)
- Grant Parker Flowers
Connecticut Innovations (Established Investigator Award 15-RMB-YALE-01)
- Craig M Crews
National Institute of General Medical Sciences (Predoctoral Training Fellowship T32GM007499)
- Lucas D Sanor
The funders had no role in study design, data collection and interpretation, or the decision to submit the work for publication.
Ethics
Animal experimentation: Experimental procedures were approved by the Yale University IACUC (2017-10557) and were in accordance with all federal policies and guidelines governing the use of vertebrate animals.
Copyright
© 2020, Sanor et al.
This article is distributed under the terms of the Creative Commons Attribution License, which permits unrestricted use and redistribution provided that the original author and source are credited.
Metrics
-
- 14,460
- views
-
- 888
- downloads
-
- 13
- citations
Views, downloads and citations are aggregated across all versions of this paper published by eLife.
Citations by DOI
-
- 13
- citations for umbrella DOI https://doi.org/10.7554/eLife.48511