Molecular basis of the PIP2-dependent regulation of CaV2.2 channel and its modulation by CaV β subunits
Abstract
High-voltage-activated Ca2+ (CaV) channels that adjust Ca2+ influx upon membrane depolarization are differentially regulated by phosphatidylinositol 4,5-bisphosphate (PIP2) in an auxiliary CaV β subunit-dependent manner. However, the molecular mechanism by which the β subunits control the PIP2 sensitivity of CaV channels remains unclear. By engineering various α1B and β constructs in tsA-201 cells, we reported that at least two PIP2-binding sites, including the polybasic residues at the C-terminal end of I–II loop and the binding pocket in S4II domain, exist in the CaV2.2 channels. Moreover, they were distinctly engaged in the regulation of channel gating depending on the coupled CaV β2 subunits. The membrane-anchored β subunit abolished the PIP2 interaction of the phospholipid-binding site in the I–II loop, leading to lower PIP2 sensitivity of CaV2.2 channels. By contrast, PIP2 interacted with the basic residues in the S4II domain of CaV2.2 channels regardless of β2 isotype. Our data demonstrated that the anchoring properties of CaV β2 subunits to the plasma membrane determine the biophysical states of CaV2.2 channels by regulating PIP2 coupling to the nonspecific phospholipid-binding site in the I–II loop.
Editor's evaluation
This manuscript describes experiments using heterologous expression to achieve molecular dissection of the effects of PIP2 and CaVβ2 auxiliary subunits on CaV2.1 (P/Q-type) calcium channels. The experiments also probe interplay between lipid effects and other modulatory pathways. Understanding the functional regulation of this channel is important because CaV2.1 channels play significant roles in neuronal plasticity.
https://doi.org/10.7554/eLife.69500.sa0Introduction
Voltage-gated Ca2+ (CaV) channels that mediate Ca2+ influx upon membrane depolarization contribute to various physiological events, including synaptic transmission, hormone secretion, excitation–contraction coupling, and gene transcription (Berridge et al., 2000; Catterall, 2011; Clapham, 2007; Li et al., 2016). CaV channels can be divided into high-voltage-activated (HVA) and low-voltage-activated (LVA) channels based on their activation voltage threshold. The HVA Ca2+ channels, which consist of the CaV1 and CaV2 families, are multiprotein complexes with a pore-forming α1 subunit and auxiliary α2δ and β subunits. Diverse cellular factors regulate CaV channel activity (Felix, 2005; Huang and Zamponi, 2017).
Among the various intracellular regulatory signals of CaV channels, we focus on the membrane phospholipid phosphatidylinositol 4,5-bisphosphate (PIP2). Previous studies have shown that PIP2 activates several types of HVA CaV channels in recombinant systems and native tissue cells (Hille et al., 2015; Rodríguez-Menchaca et al., 2012; Suh and Hille, 2008; Wu et al., 2002; Xie et al., 2016). Dr-VSP, a voltage-sensing lipid phosphatase from zebrafish, can be used to examine the effects of PIP2 on CaV channels without the involvement of other downstream second messengers generated from Gq-coupled receptors (Murata et al., 2005; Okamura et al., 2009; Suh et al., 2010). In vitro experiments using Dr-VSP have shown that most HVA Ca2+ channels are suppressed by membrane PIP2 depletion without influencing LVA Ca2+ channels (Jeong et al., 2016; Suh et al., 2010). PIP2 induces two distinct and opposing regulatory effects on CaV2.1 channels (Wu et al., 2002). Thus, the CaV2.1 channel protein was suggested to contain two distinct PIP2-interaction sites with different binding affinity (Wu et al., 2002). A more recent study showed that four arginine residues within the C-terminal end of the I–II loop of L-type CaV1.2 channels are involved in nonspecific phospholipid interactions; therefore, the substitution of these basic residues for alanine decreases current inhibition via PIP2 breakdown and increases the open probability of CaV1.2 channels (Kaur et al., 2015). The precise PIP2-binding sites have not been fully determined in CaV channels yet.
Among the auxiliary subunits, CaV β subunits directly bind to an α-interacting domain (AID) within the N-terminal region of the I–II loop. They play key roles in regulating membrane trafficking and fine-tuning the gating of CaV channels (Buraei and Yang, 2010; Buraei and Yang, 2013). A single β subunit can be divided into five distinct regions: conserved src homology-3 (SH3) and guanylate kinase (GK) domains, a flexible HOOK region connecting the two domains, and variable N- and C-terminus. The GK domain contains an α-binding pocket (ABP), which is a site for interaction with the AID of the I–II loop (Buraei and Yang, 2010; Buraei and Yang, 2013; Chen et al., 2004; Opatowsky et al., 2004; Van Petegem et al., 2004). Additionally, the HOOK region, a flexible linker composed of around 70 amino acids, is important in determining the inactivation kinetics, current density, and PIP2 regulation of CaV2.2 channels via electrostatic interaction with the plasma membrane (PM) (Miranda-Laferte et al., 2012; Park et al., 2017; Park and Suh, 2017; Richards et al., 2007). Several studies have shown that subcellular localization of the β subunits is primarily involved in the modulation of CaV channel gating, including inactivation kinetics, current density, and PIP2 sensitivity (Keum et al., 2014; Kim et al., 2015a, Kim et al., 2016; Suh et al., 2012; Takahashi et al., 2003). For example, N-type CaV2.2 channels coexpressed with membrane-anchored β subunits, such as β2a or β2e, show relatively slower inactivation kinetics, higher current density, and lower PIP2 sensitivity than channels with the cytosolic β subunit, such as β2b, β2c, or β3 (Keum et al., 2014; Kim et al., 2015a, Kim et al., 2015b; Kim et al., 2016; Suh et al., 2012). However, the underlying mechanisms for the differential regulation of CaV2.2 channel gating depending on the subcellular localization of CaV β subunits has not been clearly resolved.
Previous studies have proposed a bidentate model where two palmitoyl chains of the CaV β2a subunit compete with the interaction of the two fatty acyl chains of PIP2. Subsequently, this dislodges the PIP2 molecule from its binding site on the N-type CaV2.2 channels, decreasing the requirement for PIP2 (Heneghan et al., 2009; Hille et al., 2015; Mitra-Ganguli et al., 2009; Roberts-Crowley and Rittenhouse, 2009). Using cryo-electron microscopy (cryo-EM), Dong et al., 2021 and Gao et al., 2021 have recently shown that human CaV2.2 channels possess a PIP2-binding pocket within the S4II domain of α1B subunit. PIP2 interaction to this site is required for a minor shift of the S4II domain to the I–II loop. The functional role of the PIP2-binding site in CaV2.2 channel gating and the modulatory effects of CaV β subunits on the PIP2 interaction are yet to be defined. In this study, we developed diverse engineered α1B and β constructs and found that the CaV2.2 channels were regulated by PIP2 through at least two distinct interacting sites, including a nonspecific phospholipid-binding motif in the distal I–II loop and the binding pocket in the S4II domain. Our results revealed that the PM-anchored β2a subunit selectively disrupted PIP2 interaction with the phospholipid-binding site in the I–II loop, leading to a channel state less sensitive to Dr-VSP-induced PIP2 depletion. However, the S4II-binding pocket of CaV2.2 channels interacted with PIP2 regardless of the coupled β2 isotype. The present study provides new insights into the reciprocal roles of the CaV β subunits and membrane PIP2 in HVA CaV channel regulation.
Results
N-terminal length of PM-tethering CaV β subunit is important in determining current inactivation and PIP2 sensitivity of CaV2.2 channels
We have previously reported that subcellular localization of the CaV β subunit plays an important role in determining the inactivation kinetics and PIP2 sensitivity of CaV2.2 channels (Keum et al., 2014; Kim et al., 2015a, Kim et al., 2016). By manipulating the β2 constructs, we further examined how CaV β subunits determine the gating properties of the CaV2.2 channel depending on their subcellular localization. First, we used a palmitoylation-resistant cytosolic mutant form of β2a, β2a(C3,4S), where two palmitoylation sites (C3 and C4) in the N-terminus of the β2a subunit were mutated to serine residues (Chien et al., 1996; Hurley et al., 2000; Olcese et al., 1994; Qin et al., 1998; Figure 1A and Figure 1—figure supplement 1A). Additionally, we constructed two more membrane-recruited β2c analogs by adding membrane-targeting Lyn11 (N-terminal G2-myristoylation and C3-palmitoylation modification sequence from Lyn kinase; Resh, 1994) or Lyn11 plus a flexible 48 amino acid linker (Lyn-48aa) to the N-terminus of β2c. When these CaV β constructs were expressed in cells without the pore-forming α1B, β2a(C3,4S) was distributed through the cytosol similar to β2c. By contrast, the engineered Lyn-β2c and Lyn-48aa-β2C were localized at the PM like the membrane-anchored β2a subunit (Figure 1B, C). However, in the presence of α1B and α2δ1, all the β2 constructs were mainly distributed at the PM, probably via binding to α1B subunits (Figure 1—figure supplement 1B, C). This suggested that amino acid mutation or chimeric modification of the β2 subunit does not affect the formation of the CaV2.2 channel multicomplex. Next, we tested the effects of the β2 constructs on current inactivation and PIP2 sensitivity of the CaV2.2 channels. PIP2 regulation of CaV2.2 channel gating was measured as the difference before and after a + 120 mV depolarizing pulse using Dr-VSP (see Figure 1—figure supplement 2A). Coexpression of β2a(C3,4S) accelerated current inactivation and increased the PIP2 sensitivity of CaV2.2 channels, such as those with the cytosolic β2c subunit. Expression of the chimeric Lyn-β2c slowed down the inactivation rate and decreased PIP2 sensitivity, like the channels with the PM-anchored β2a subunit (Figure 1D, E). Interestingly, cells co-transfected with the PM-tethered chimeric Lyn-48aa-β2c showed faster current inactivation and higher PIP2 sensitivity in CaV2.2 channels, which were similar to the responses of channels with the cytosolic β2c subunit. In control experiments without Dr-VSP, we confirmed that the current amplitudes of CaV2.2 channels with the developed β2 constructs were not significantly different before and after the depolarizing pulse (Figure 1—figure supplement 2A, B). Additionally, we verified that the effects of Dr-VSP were not due to relieving the Gβγ-mediated tonic inhibition from the CaV2.2 channels. As shown in Figure 1—figure supplement 2C, prepulse depolarization did not change the current amplitudes in cells intracellularly perfused with 1 mM of the G protein inhibitor GDP-β-S instead of GTP in the absence of Dr-VSP. Moreover, the CaV2.2 channels with GDP-β-S showed very similar PI(4,5)P2 sensitivities to those in experiments with GTP in cells expressing Dr-VSP (Figure 1—figure supplement 2D). This suggested that 0.1 mM GTP concentration in the pipette solution was not sufficient to trigger spontaneous G protein activation or suppress CaV2.2 channels through Gβγ binding.
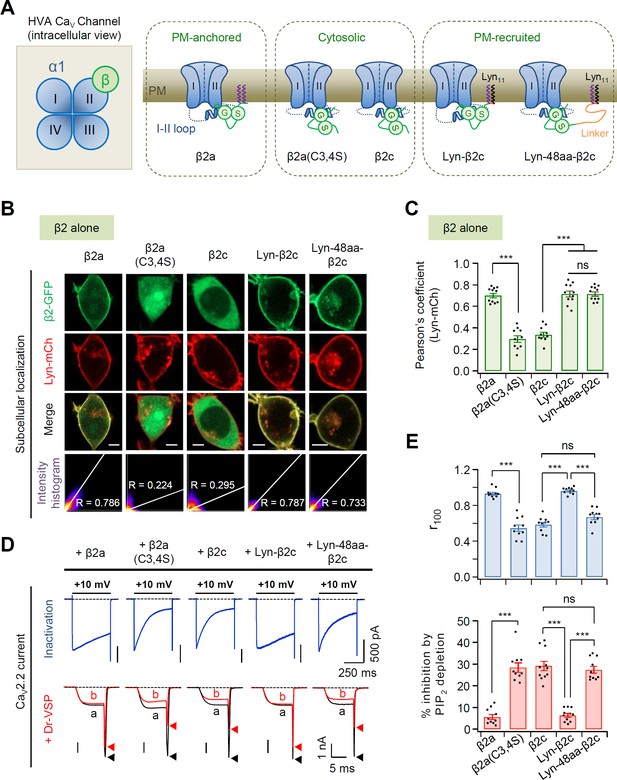
Current inactivation and PIP2 sensitivity in N-type CaV2.2 channels with different subtypes of the β2 subunit.
(A) Schematic diagram of high-voltage-activated (HVA) calcium channel complex viewed from the intracellular side (left). CaV β subunit is located beside the domain II of α1B in the cytosolic side while CaV α2δ subunit is mostly localized at the extracellular surface of the channel protein (Gao et al., 2021). Schematic model of CaV2.2 channels with plasma membrane (PM)-anchored β2a, cytosolic β2a(C3,4S) and β2c, or N-terminus engineered PM-recruited β2c (right). (B) Representative confocal images of tsA-201 cells expressing the PM marker Lyn-mCh and β2 isoforms or its derivatives fused to GFP without the α1 and α2δ1 subunits. Scale bar, 5 μm. The scatter plot shows a 2D intensity histogram of the red (Lyn-mCh) and green (β2-GFP) pixels in the confocal image. The value indicates the Pearson’s correlation coefficient (R) that is obtained by the Colocalization Threshold plugin of Fiji software (Image J). (C) Summary of Pearson’s coefficient between Lyn-mCh and the β2 construct (n = 10–11). (D) Current inactivation of CaV2.2 channels with β2 isoforms or its derivatives was measured during 500-ms test pulses to +10 mV (top). Current inhibition of CaV2.2 channels by Dr-VSP-mediated PIP2 depletion (bottom). The current traces before (a) and after (b) the strong depolarizing pulse to +120 mV were superimposed. Peak tail current is indicated by arrowheads (trace a, black head; trace b, red head). (E) Summary of current inactivation (top; n = 10–11) and inhibition (%) by PIP2 depletion (bottom; n = 10–11) in CaV2.2 channels with the β2 constructs. r100 indicates the fraction of current remaining after 100-ms depolarization to +10 mV (top). Dots indicate the individual data points for each cell. Data are mean ± standard error of the mean (SEM). ***p < 0.001, using one-way analysis of variance (ANOVA) followed by Tukey post hoc test.
-
Figure 1—source data 1
Current inactivation (r100) and current inhibition (%) by PIP2 depletion in N-type CaV2.2 channels with different subtypes of the β2 subunit.
- https://cdn.elifesciences.org/articles/69500/elife-69500-fig1-data1-v1.xlsx
We further examined the effects of the length of the flexible linker between Lyn and the β2c subunit on the inactivation kinetics and PIP2 sensitivity of CaV2.2 channels. As shown in Figure 1—figure supplement 3, when the inserted linkers were longer than 24 aa, current inactivation was faster and current inhibition by PIP2 depletion was stronger. Together, these data suggest that the N-terminal length of the PM-tethering CaV β subunit is critical in determining the inactivation kinetics and PIP2 sensitivity of CaV2.2 channels.
Proximal interaction of the fatty acyl chains with channel complex underlies the β subunit-dependent regulation of CaV2.2 channel gating
It has been previously reported that disruption of the SH3–GK interaction in the membrane-anchored β2a subunit accelerates the channel inactivation of CaV2.1 channels (Chen et al., 2009). The GK domain of the CaV β subunit interacts directly with the AID domain in the I–II loop of CaV α1 subunits (Buraei and Yang, 2010; Buraei and Yang, 2013; Chen et al., 2004; Opatowsky et al., 2004; Van Petegem et al., 2004); therefore, disruption of the SH3–GK interaction in the CaV β subunit may increase the length between the N-terminus and the GK–AID complex through the flexible HOOK region. To test the possible effects of increased N-terminal length from the AID–GK complex on CaV channel gating, we constructed mutant β2a subunits in which the SH3–GK intramolecular interaction was disrupted by mutating seven amino acids in the SH3 and GK domains to alanine residues (Figure 2A). Additionally, the N-terminus was deleted to abolish membrane targeting of the β2a subunit by itself, and Lyn11 was inserted to the N-terminus for membrane recruitment. Without α1B and α2δ1, both N-terminus-deleted (∆N)β2 WT and (∆N)β2 Mut, in which the SH3–GK interaction was disrupted, were expressed in the cytosol. Conversely, Lyn-(∆N)β2 WT and Lyn-(∆N)β2 Mut constructs were localized to the PM (Figure 2A, inset images). In CaV2.2 channels with the N-terminus-deleted mutant (∆N)β2 WT, the current exhibited fast inactivation and high PIP2 sensitivity (Figure 2B–D). These phenomena similarly appeared in channels with the (∆N)β2 Mut. In contrast, CaV2.2 channels with Lyn-(∆N)β2 WT exhibited slow inactivation and weak PIP2 sensitivity. However, the channels with Lyn-(∆N)β2 Mut exhibited fast inactivation and strong PIP2 sensitivity, like channels with cytosolic (∆N)β2 WT and (∆N)β2 Mut (Figure 2B–D). We also confirmed that disruption of the SH3–GK interaction did not shift the current–voltage (I–V) curve of CaV2.2 currents (Figure 2—figure supplement 1). These data suggested that the length from the N-terminal lipid anchor to the GK domain of β subunit is crucial in determining the inactivation rate and PIP2 sensitivity of CaV2.2 channels.
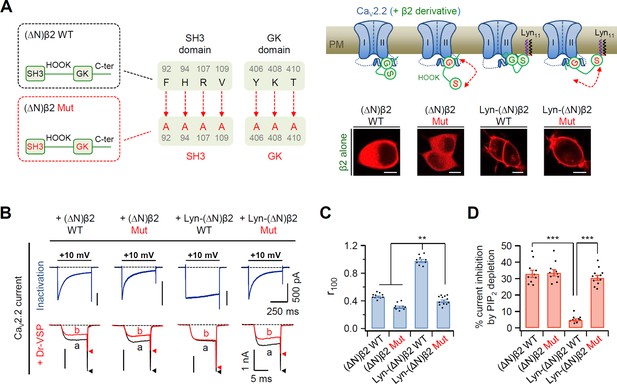
Disruption of SH3–GK interaction in the plasma membrane (PM)-recruited CaV β2 subunit leads to an increase in both current inactivation and PIP2 sensitivity of CaV2.2 channels.
(A) Left, a diagram showing how the SH3–GK intramolecular interaction is disrupted in β2 constructs (top). Phenylalanine 92, histidine 94, arginine 107, and valine 109 residues in the SH3 domain and tyrosine 406, lysine 408, and threonine 410 residues in the GK domain are replaced with alanine. Schematic model of CaV2.2 channels with engineered β2 constructs in which the SH3–GK intramolecular interaction is disrupted. Lyn-(∆N)β2: Lyn-labeled N-terminus-deleted β2 construct. Lyn-(∆N)β2 Mut: Lyn-(∆N)β2 construct with a disrupted SH3–GK intramolecular interaction. Inset: confocal images of tsA-201 cells expressing engineered β2 constructs labeled with mCherry without α1B and α2δ1 subunits. Scale bar, 5 μm. (B) Representative currents of CaV2.2 channels with engineered β2 constructs. The currents were measured during 500-ms test pulses to +10 mV (top). Current traces before (a) and after (b) a + 120-mV depolarizing pulse in cells expressing CaV2.2 channels with engineered β2 constructs and Dr-VSP (bottom). Peak tail current is indicated by arrowheads (trace a, black head; trace b, red head). (C) Summary of CaV2.2 current inactivation (n = 9–12). r100 indicates the fraction of current remaining after 100-ms depolarization to +10 mV. (D) Summary of CaV2.2 current inhibitions (%) by PIP2 depletion in Dr-VSP-expressing cells (n = 9–11). Dots indicate the individual data points for each cell. Data are mean ± standard error of the mean (SEM). **p < 0.01, ***p < 0.001, using one-way analysis of variance (ANOVA) followed by Tukey post hoc test.
-
Figure 2—source data 1
Current inactivation (r100) and current inhibition (%) by PIP2 depletion in N-type CaV2.2 channels with the engineered β2 construct.
- https://cdn.elifesciences.org/articles/69500/elife-69500-fig2-data1-v1.xlsx
To further examine the functional role of length between lipid anchor and GK domain on CaV channel gating in live cells, we developed new chimeric β2 constructs by applying the rapamycin-induced dimerizing system FK506-binding protein (FKBP) and FKBP–rapamycin-binding (FRB) protein (Banaszynski et al., 2005; Inoue et al., 2005; Suh et al., 2006). As shown in Figure 3A, FKBP and FRB proteins irreversibly assembled to form a ternary complex upon application of rapamycin, which led to shortening of the length between the lipid anchor Lyn11 and GK–AID domains. We fused a Förster resonance energy transfer (FRET) probe YFP to the C-terminus of all β2 chimera to investigate whether the FKBP domain was really translocated to the PM to make a Lyn11-FRB and FKBP complex closely after rapamycin addition (Figure 3A, right diagram). In experiments with the β chimera without the FKBP domain (Control: Lyn-FRB-HOOK-GK), both FRETr and the current amplitude of CaV2.2 channels were not changed by rapamycin addition (Figure 3B). Consistently, rapamycin treatment did not affect current inactivation and the PIP2 sensitivity of CaV2.2 channels in these cells (Figure 3C–F). In contrast, in CaV2.2 channels with Lyn-FRB-HOOK-GK-FKBP (RF), rapamycin treatment irreversibly enhanced the FRETr signal and increased the current amplitude of CaV2.2 channels (Figure 3B, middle and Figure 3—figure supplement 1). Moreover, rapamycin treatment reduced the current inactivation and PIP2 sensitivity of CaV2.2 channels (Figure 3C–F). However, in CaV2.2 channels with Lyn-FRB-HOOK-GK-Linker-FKBP (RCF), where a 194-aa linker was inserted between GK and FKBP, rapamycin enhanced the FRETr signal without causing significant changes in the current amplitude (Figure 3B, right and Figure 3—figure supplement 1). The effects of rapamycin on inactivation kinetics and PIP2 sensitivity were much weaker in CaV2.2 channels with RCF when compared with those in channels with RF (Figure 3C–F). This suggested that rapamycin-induced dimerization may be insufficient to shorten the length between the lipid anchor and isolated GK domain of β subunit in channels with RCF.
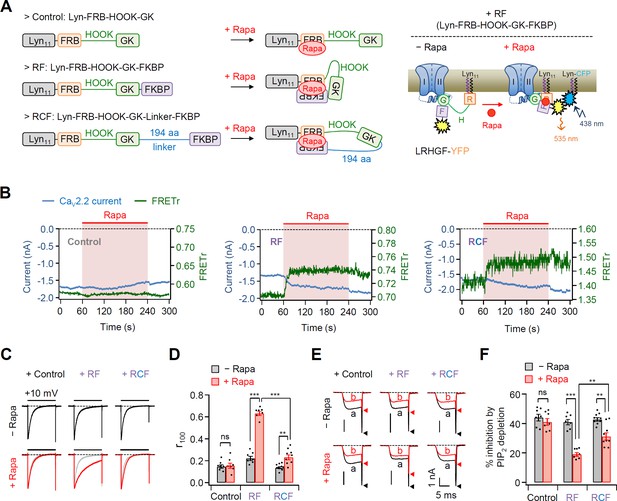
Effects of the real-time translocation of the GK domain to the plasma membrane (PM) on CaV2.2 channel gating.
(A) Left, a schematic diagram showing rapamycin-induced translocatable β2 chimeric constructs. Translocatable β2 chimeric constructs were invented by fusing FRB or FKBP to the N- and C-termini of the GK domain, respectively. The new constructs were tagged with Lyn11 (RF or Lyn-FRB-Hook-GK-FKBP) to be tethered to the PM. Rapamycin (Rapa) addition triggers the formation of a tripartite FRB–rapamycin–FKBP complex, resulting in the movement of the FKBP domain to the PM (right). For Förster resonance energy transfer (FRET) imaging, chimeric β constructs labeled with YFP in the C-terminus and PM-targeting Lyn-CFP were coexpressed. Right, schematic model of CaV2.2 channels with RF before and after rapamycin application. Rapamycin induces the formation of the tripartite complex, resulting in a shift of the FKBP domain to the PM and an enhanced FRET signal. (B) Time courses of CaV2.2 currents (blue traces) and FRET ratio (green traces) were measured simultaneously in single cells expressing CaV2.2 channels with Cont (left), RF (middle), or RCF (right) and the membrane marker Lyn-CFP. (C) Current inactivation of CaV2.2 channels with Cont (left), RF (middle), and RCF (right) was measured during 500-ms test pulses to +10 mV before (black traces) and after (red traces) rapamycin addition. (D) Summary of inactivation of CaV2.2 currents before (black bars) and after (red bars) rapamycin application (n = 7–9). The fraction of the current remaining after 100-ms depolarization (r100) to +10 mV. (E) Current inhibition of Dr-VSP-mediated PIP2 depletion on CaV2.2 channels with Cont (left), RF (middle), and RCF (right) before and after rapamycin addition. The traces before (a) and after (b) the depolarizing pulse to +120 mV were superimposed. Peak tail current is indicated by arrowheads (trace a, black head; trace b, red head). (F) Summary of Dr-VSP-induced CaV2.2 current inhibition before (black bars) and after (red bars) rapamycin addition (n = 7–9). Dots indicate the individual data points for each cell. Data are mean ± standard error of the mean (SEM). **p < 0.01, ***p < 0.001, using two-way analysis of variance (ANOVA) followed by Sidak post hoc test.
-
Figure 3—source data 1
Time courses of CaV2.2 currents and Förster resonance energy transfer (FRET) ratio.
- https://cdn.elifesciences.org/articles/69500/elife-69500-fig3-data1-v1.xlsx
-
Figure 3—source data 2
Current inactivation (r100) and current inhibition (%) by PIP2 depletion in CaV2.2 channels with rapamycin-induced translocatable β2 chimeric constructs before and after rapamycin.
- https://cdn.elifesciences.org/articles/69500/elife-69500-fig3-data2-v1.xlsx
Next, we measured the effects of the N-terminal length of PM-tethered β subunit on CaV2.2 channel activity by inserting flexible linkers of various lengths between Lyn11 and the GK domain of β2 (Figure 4A). The inserted linkers were unstructured flexible peptides (see Figure 4—figure supplement 1); therefore, the length of the linkers was calculated using the worm-like chain (WLC) model (see Methods). Our results showed that both the current inactivation and PIP2 sensitivity of CaV2.2 channels became gradually stronger as the inserted flexible linkers became longer (Figure 4B–D). Consistently, the current activation was gradually accelerated by the increase in linker length (Figure 4—figure supplement 2). However, no additional difference was detected in channels with the membrane-tethered Lyn-43aa-GK subunit when compared with the cytosolic GK subunit. This indicated that the GK domain with the length of the inserted 43-aa linker is sufficient to act like the cytosolic CaV β subunit (Figure 4B–D). Interestingly, the PIP2 sensitivity and inactivation kinetics of CaV channels were differentially regulated by the length between the lipid anchor and the GK domain: the channels with Lyn-43aa-GK showed faster inactivation than the channels with Lyn-22aa-GK, whereas the PIP2 sensitivity of the two channels was not significantly different (Figure 4B–E). Additionally, our data analysis indicated that the biophysical gating properties of CaV2.2 channels with a membrane-anchored β2a subunit were similar to those of channels with Lyn-9aa-GK. Furthermore, the gating properties of CaV2.2 channels coupled with cytosolic β2c were similar to those of channels with Lyn-20aa-GK (Figure 4E).
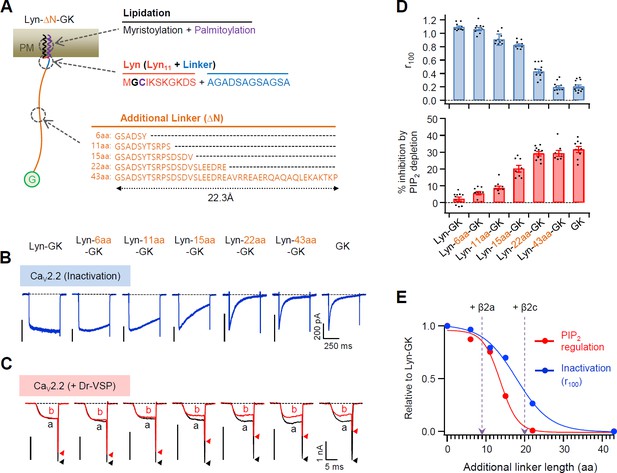
Flexible linker length between Lyn and the GK domain of the β subunit performs a key role in determining both the current inactivation and the PIP2 sensitivity of CaV2.2 channels.
(A) Schematic diagram of diverse flexible linkers (∆N) inserted between Lyn and GK (G) domain. The length of each linker is calculated by the worm-like chain (WLC) model (see Methods). Amino acid sequences of Lyn (Lyn11 plus 12 aa linker) and the additional linkers are listed. (B) Current inactivation of CaV2.2 channels with diverse CaV β-GK derivatives was measured during 500 ms test pulses to +10 mV. (C) Effects of Dr-VSP-mediated PIP2 depletion on CaV2.2 channels with GK domain derivatives. Peak tail current is indicated by arrowheads (trace a, black head; trace b, red head). (D) Summary of current inactivation (blue bars; n = 9–12) and inhibition (%) by PIP2 depletion (red bars; n = 8–10) in CaV2.2 channels with CaV β GK derivatives. Data are mean ± standard error of the mean (SEM). Dots indicate the individual data points for each cell. (E) Normalized mean current inactivation and mean current inhibition by PIP2 depletion versus additional linker length (aa) of CaV β GK derivatives measured in CaV2.2 channels. The normalized current regulation in cells expressing CaV2.2 with β2a and β2c is indicated with dashed arrows.
-
Figure 4—source data 1
Current inactivation (r100) and current inhibition (%) by PIP2 depletion in CaV2.2 channels with the engineered β2 GK derivatives.
- https://cdn.elifesciences.org/articles/69500/elife-69500-fig4-data1-v1.xlsx
Previous studies have reported that subcellular localization of the CaV β subunit is important in determining the current density of CaV channels, where CaV channels with the membrane-anchored β subunit show relatively higher current density than channels with the cytosolic β subunit (Suh et al., 2012). In line with this, we found that the current density of CaV2.2 channels with β2a was significantly higher than that of channels with β2c (Figure 4—figure supplement 3A–C). Therefore, we tested whether the current density of CaV channels was dependent on the N-terminal length. CaV2.2 channels showed slightly decreased current density that was dependent on the expansion of the flexible linker length between Lyn and the GK domain alone (Figure 4—figure supplement 4A, C). This phenomenon was observed in channels with the whole β2c subunit with Lyn (Figure 4—figure supplement 3D–F). We tested whether the length between N-terminal lipid anchor and GK domain affected the voltage-dependent gating of CaV channels. Voltage-dependent activation of CaV2.2 channels with Lyn-linker-GK derivatives showed a greater shift to positive voltage as the inserted flexible linkers increased in length (Figure 4—figure supplement 4B, D). This suggested that incremental increases in linker length lead to a decreased voltage sensitivity. There was no difference in the current density and voltage-dependent activation between CaV channels with the Lyn-43aa-GK and GK subunit. Together, these results suggested that differential regulation of CaV2.2 channel gating by β subunits is mainly determined by the anchoring properties of the β subunits to PM.
Polybasic motif at the C-terminal end of I–II loop plays an important role in the PIP2 regulation of CaV2.2 channels
How does the N-terminal length of the PM-tethering CaV β subunit regulate CaV channel gating? Recently, Kaur et al., 2015 have reported that a polybasic motif consisting of four basic amino acids within the C-terminal end of the I–II loop of L-type CaV1.2 channels interacts with membrane phospholipids, including PIP2. Additionally, the putative PIP2-binding site is conserved in the I–II loop of N-type CaV2.2 channels (Figure 5—figure supplement 1). We examined whether the polybasic motif affects the PIP2 sensitivity of CaV2.2 channels. First, we eliminated the potential phospholipid-binding motif from the CaV2.2 channel I–II loop by mutating the four polybasic residues to alanine (4A α1B) (Figure 5A). In CaV2.2 channels with the β2a subunit, the inactivation kinetics of the current did not differ between WT α1B and 4A α1B (Figure 5B, C, left). However, in CaV2.2 with β2c, the inactivation rate was slower in 4A α1B channels (Figure 5B, C, right). The effects of PIP2 depletion on current amplitude were also measured in these channels. In control experiments without Dr-VSP, the current of WT or 4A-mutant CaV2.2 channels did not significantly differ before and after a + 120-mV depolarizing pulse in cells with either β2a or β2c subunits (Figure 5D). By contrast, PIP2 depletion by Dr-VSP activation similarly inhibited the CaV current by approximately 5% in cells expressing either WT or 4A CaV2.2 channels with a PM-anchored β2a subunit (Figure 5E, left). This indicated the presence of another PIP2-binding site in the α1B subunit other than this polybasic motif in I–II loop. On the other hand, the PIP2 sensitivity in channels with β2c was dramatically reduced in 4A channels, indicating that the polybasic motif in the I–II loop plays a key role in PIP2 regulation of CaV2.2 channels with the cytosolic β subunit (Figure 5D, E). However, in cells expressing 4A CaV2.2 channels with β2c, we observed another ~5% current inhibition by PIP2 depletion. This was similar to the CaV2.2 channels with β2a.
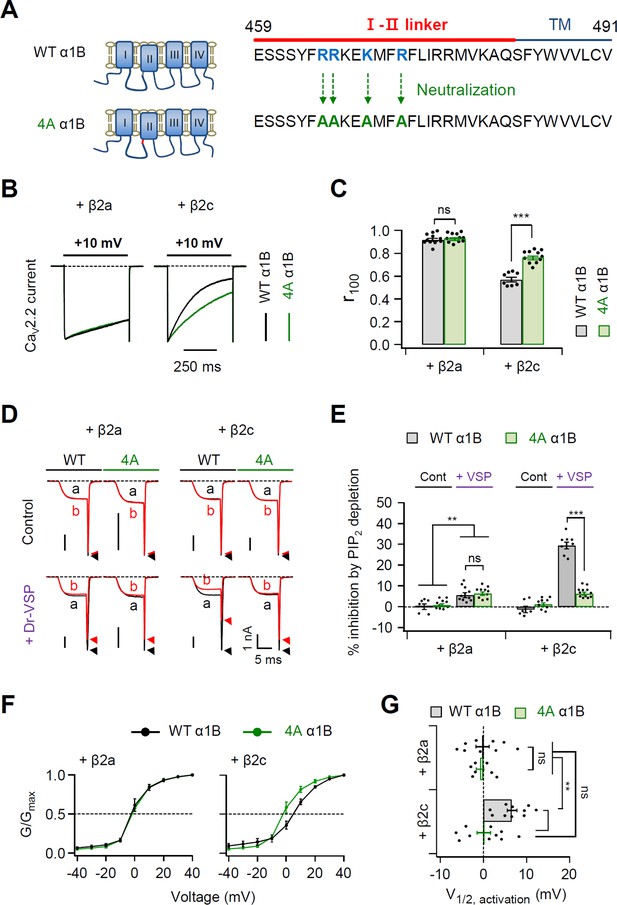
Polybasic motif at the C-terminal end of the I–II loop influences determination of steady-state activation, current inactivation, and PIP2 sensitivity of CaV2.2 channels.
(A) Schematic diagram of phospholipid-binding residue-neutralizing mutations within the C-terminal end of the I–II loop in the α1B subunit. The phospholipid-binding residues (R465, R466, K469, and R472) highlighted in blue were mutated to alanine (4A). (B) Current inactivation was measured during 500-ms test pulses to +10 mV in cells expressing α1B WT (black traces) and 4A mutants (green traces) with β2a (left) or β2c (right) subunits. (C) Summary of current inactivation of CaV2.2 WT (black bars) and 4A (red bars) with β2 subunits (n = 8–11). r100 indicates the fraction of current remaining after 100-ms depolarization to +10 mV. (D) Current inhibition by Dr-VSP-mediated PIP2 depletion in cells expressing CaV2.2 WT and 4A with the β2a (left) or β2c subunit (right). CaV2.2 currents before (a) and after (b) the depolarizing pulse to +120 mV are superimposed in control (top) and Dr-VSP-expressing (bottom) cells. Peak tail current is indicated by arrowheads (trace a, black head; trace b, red head). (E) Summary of current inhibition (%) of CaV2.2 WT (black bars) and 4A (red bars) by PIP2 depletion in control (n = 10) and Dr-VSP-transfected cells (n = 8–12). (F) The voltage dependence of normalized steady-state activation (G/Gmax) for α1B WT (black) and 4A mutants (green) with β2a (left) or β2c (right) subunits. Tail currents elicited between −40 and +40 mV in 10 mV steps, from a holding potential of −80 mV were normalized to the largest tail current in each series of test pulse. The curves were fitted by a Boltzmann function. Dashed line indicates the V1/2 of normalized steady-state activation. (G) Summary of the V1/2 of normalized steady-state activation in cells expressing α1B WT (black bars) and 4A mutants (green bars) with β2a (upper) or β2c (bottom) subunits (n = 7–10). Dots indicate the individual data points for each cell. Data are mean ± standard error of the mean (SEM). **p < 0.01, ***p < 0.001, using two-way analysis of variance (ANOVA) followed by Sidak post hoc test.
-
Figure 5—source data 1
Current inactivation (r100) and current inhibition (%) by PIP2 depletion in cells expressing α1B WT and 4A mutants with β2a and β2c.
- https://cdn.elifesciences.org/articles/69500/elife-69500-fig5-data1-v1.xlsx
Next, we investigated whether the polybasic motif affects the PIP2 sensitivity of CaV2.2 channels with Lyn-β2c and Lyn-48aa-β2c (Figure 5—figure supplement 2). Similar to β2a, we did not detect any significant differences in current inactivation and PIP2 sensitivity between WT and 4A mutant CaV2.2 with Lyn-β2c (Figure 5—figure supplement 2). Conversely, WT CaV2.2 channels with Lyn-48aa-β2c exhibited faster inactivation and higher PIP2 sensitivity, which was similar to the responses of CaV2.2 channels with cytosolic β2c. However, in cells expressing 4A mutant CaV2.2 channels with Lyn-48aa-β2c, the current inactivation was slowed and the PIP2 sensitivity was decreased to ~5% (Figure 5—figure supplement 2). The PIP2 sensitivities of 4A CaV2.2 channels with Lyn-β2c and Lyn-48aa-β2c did not significantly differ and were similar to that of WT channels with Lyn-β2c. Consistent with the data in Figure 5, these results suggested that the polybasic motif within the I–II loop interacts with membrane PIP2 in CaV2.2 channels with β2c-like Lyn-48aa-β2c, but not with β2a-like Lyn-β2c subunits. On the other hand, in channels with the β2a subunit, there was no significant difference in the voltage-dependent activation between WT α1B and 4A α1B (Figure 5F, G). However, the activation of 4A α1B with the β2c subunit was significantly shifted toward the hyperpolarization direction when compared with WT α1B channels with β2c (Figure 5F, G). In addition, the activation curve of 4A α1B with β2c was similar to the curves of WT and 4A α1B with β2a (Figure 5F, G). Together, our data suggested that two different PIP2-interacting sites with differential PIP2 sensitivities exist in CaV2.2 channels. More importantly, our data indicate that PIP2 interacts with the polybasic motif when CaV2.2 is expressed with cytosolic β subunits but not when expressed with lipidated membrane-anchored β subunit.
Finally, we determined whether other arginine residues in the distal region of polybasic motif also affected the PIP2 sensitivity of CaV2.2 channels (Figure 5—figure supplement 3). For this, two arginine residues (R476 and R477) near the polybasic motif were replaced with alanine (α1B R476,477A) (Figure 5—figure supplement 3A). We also constructed a α1B R465,466A by mutating only two arginine residues (R465 and R466) in the polybasic motif (R465, R466, K469, and R472) (Figure 5—figure supplement 3A). In CaV2.2 channels with the β2a subunit, we did not detect any significant differences in current inactivation and PIP2 sensitivity among WT α1B, α1B R465,466A, and α1B R476,477A (Figure 5—figure supplement 3B–E). However, in CaV2.2 with β2c, the inactivation rate was slower and the PIP2 sensitivity was weaker in both α1B R465,466A and α1B R476,477A compared to WT α1B (Figure 5—figure supplement 3B–E).
Differential modulation of CaV2.2 channels by muscarinic receptor stimulation in cells expressing PM-anchored or cytosolic β subunit
To examine whether the polybasic motif influenced the Gq-coupled modulation of CaV2.2 channels, we applied the muscarinic acetylcholine receptor agonist, oxotremorine-M (Oxo-M), to cells co-transfected with the M1 muscarinic receptor (M1R) (Figure 6). Since the M1R stimulation suppressed CaV2.2 channels through both Gβγ binding to channels and PIP2 depletion (Keum et al., 2014), we then used a Gβγ-insensitive chimeric CaV2.2 channel construct, α1C-1B, to examine the effect of PIP2 depletion alone on channel regulation (Figure 6). In this chimera construct, the N-terminus of CaV2.2 (α1B), which contains one of the Gβγ interaction sites, is replaced by the N-terminus of CaV1.2 (α1C) (Agler et al., 2005). M1R activation inhibited the current by approximately 5% in cells expressing either α1C-1B WT or 4A channels with β2a subunit, which were similar to the responses of regulation by Dr-VSP-mediated PIP2 depletion in those channels (Figure 6B, C). However, consistent with the results for Dr-VSP-induced channel modulation, current suppression was much weaker in α1C-1B 4A channels with β2c than in α1C-1B WT with β2c (Figure 6B, C). We confirmed that the current suppression by M1R activation were not recovered in both α1C-1B WT and α1C-1B 4A channels by a prepulse regardless of the coupled β2 isotypes (Figure 6D, E). We additionally used Gi-coupled M2 muscarinic receptor (M2R) to further examine whether the polybasic motif in I–II loop affects the Gβγ-mediated modulation of CaV2.2 channels (Figure 6—figure supplement 1). M2R activation inhibited the currents evoked by a + 10-mV test pulse without significant difference between WT and 4A α1B with β2a or β2c (Figure 6—figure supplement 1B, C). M2R activation commonly slowed down the activation kinetics of CaV2.2 currents (Figure 6—figure supplement 1D). We have previously reported that subcellular localization of the CaV β subunit plays important roles in determining the Gβγ-dependent inhibition of CaV2.2 channels; membrane-anchored β2a subunit changes CaV2.2 channels are more sensitive to Gβγ-mediated voltage-dependent inhibition, whereas cytosolic β2b and β3 subunit changes channels are less sensitive to Gβγ-mediated voltage-dependent inhibition (Keum et al., 2014). In CaV2.2 channels with the β2a subunit, the recoveries from Gβγ-mediated inhibition did not significantly differ between WT α1B and 4A α1B (Figure 6—figure supplement 1E, F). However, in CaV2.2 with the β2c subunit, there was less recovery from Gβγ-mediated inhibition in α1B WT than in α1B 4A (Figure 6—figure supplement 1E, F). Recovery from M2R-mediated inhibition in 4A α1B with β2c was similar to the values of WT and 4A α1B with β2a (Figure 6—figure supplement 1E, F).
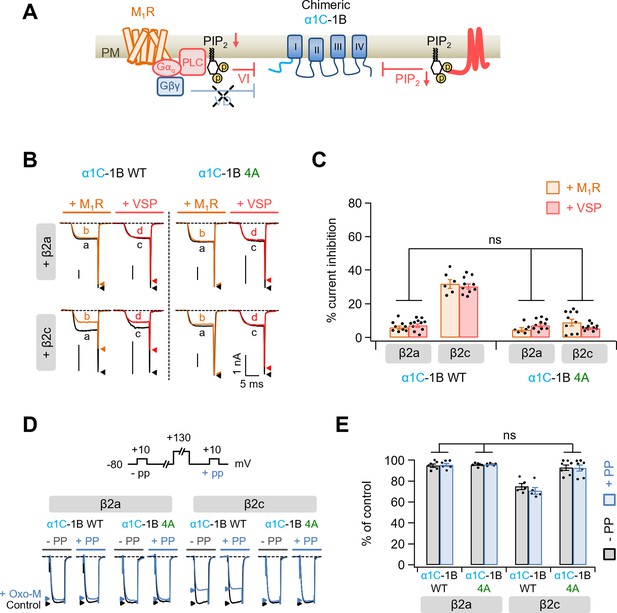
Modulation by M1 muscarinic stimulation and Dr-VSP activation in Gβγ-insensitive chimeric α1C-1B CaV2.2 channel.
(A) Schematic diagram showing the inhibitory signaling from M1 muscarinic acetylcholine receptor (M1R) and Dr-VSP to Gβγ-insensitive chimeric α1C-1B channel. VI, voltage-independent inhibition; VD, voltage-dependent inhibition. (B) Current traces before (a, black) and during (b, orange) the 10 µM Oxo-M application or before (c, black) and after (d, red) the Dr-VSP activation in cells expressing the α1C-1B WT and α1C-1B 4A with β2a or β2c subunits. Peak tail current is indicated by arrowheads (trace a, black head; trace b, orange head; trace c, black head; trace d, red head). (C) Summary of current inhibition (%) of α1C-1B WT and α1C-1B 4A by M1R stimulation (orange bars) or Dr-VSP activation (red bars) in cells with β2a or β2c subunits (n = 6–11). (D) Current traces before (control, black) and during the Oxo-M application (+Oxo-M, blue) were superimposed. Cells were given a test pulse (−PP) and then depolarized to +130 mV, followed by the second test pulse after 20 ms (+PP). Peak current is indicated by arrowheads (control, black head; +Oxo-M, blue head). (E) Summary of the prepulse experiments in before and Oxo-M perfused cells with α1C-1B WT and α1C-1B 4A with β2a or β2c subunits (n = 5–7). The current amplitude after Oxo-M application is given as percentage of the initial control. Dots indicate the individual data points for each cell. Data are mean ± standard error of the mean (SEM).
-
Figure 6—source data 1
Current inhibition (%) of α1C-1B WT and 4A mutants by M1R or Dr-VSP activation in cells expressing with β2a and β2c.
Summary of the prepulse experiments in before and Oxo-M perfused cells with α1C-1B WT and 4A mutants with β2a or β2c subunits.
- https://cdn.elifesciences.org/articles/69500/elife-69500-fig6-data1-v1.xlsx
PIP2-binding site in S4II domain is important in maintaining the CaV2.2 channel activity regardless of the coupled β2 isotype
Recently, the cryo-electron microscopic structure of human CaV2.2 complex composed of α1B, α2δ1, and β3 subunits was revealed at a resolution of 3.0 Å (Dong et al., 2021; Gao et al., 2021). These studies have shown that the 5-phosphate group of membrane PIP2 interacts with two basic residues (R584 and K587) within S4II domain of α1B. We examined whether the two basic residues affect the PIP2 sensitivity of CaV2.2 channels. First, we constructed neutralized mutant α1B subunits in which the two basic residues in S4II were replaced by alanine residues (α1B RA/KA) (Figure 7A). In CaV2.2 channels with β2a, the inactivation kinetics of the current were not changed in α1B and α1B RA/KA, regardless of the 4A mutation (Figure 7B, C). In CaV2.2 with β2c, WT α1B RA/KA showed faster inactivation than WT α1B, whereas 4A α1B RA/KA showed much slower but similar inactivation to those of α1B and α1B RA/KA with β2a (Figure 7B, C). Additionally, the effects of PIP2 depletion on current amplitude were measured in these mutant channels. Mutation of the two basic residues in S4II completely abolished the Dr-VSP-mediated current inhibition in cells expressing WT α1B RA/KA or 4A α1B RA/KA with the β2a subunit, while there was approximately 5% inhibition in cells expressing WT and 4A α1B with β2a (Figure 7D, E). Importantly, PIP2 depletion significantly inhibited the currents in cells expressing WT α1B RA/KA and β2c, whereas 4A α1B RA/KA exhibited no current inhibition, like α1B RA/KA with β2a (Figure 7D, E). Since the mutation of two basic residues changes the gating charges of S4II voltage-sensor domain, we additionally tested if other charge residues within the S4II similarly affects PIP2 sensitivity of CaV2.2 channels. We eliminated two adjacent arginine residues (R578 and R581) in S4II by replacing with alanine (α1B R578,581A) (Figure 7—figure supplement 1A). In both WT α1B and α1B R578,581A channels with β2a or β2c, there was no significant changes in the current inhibition by PIP2 depletion (Figure 7—figure supplement 1B, C), suggesting that the R578 and R581 charge residues near the PIP2-binding pocket were not involved in the PIP2 interaction. Next, we examined the functional role of the PIP2-binding site within S4II in the voltage-dependent activation of CaV2.2 channels. Regardless of β2 isotype, the activation curves were significantly shifted toward the depolarization direction in both WT α1B RA/KA and 4A α1B RA/KA (Figure 7F, G). Together, our results suggest that the two basic residues within the S4II domain consistently interact with PIP2 regardless of the coupled β2 isotype. Additionally, PIP2-binding to the S4II-binding pocket is important in maintaining stable CaV2.2 channel gating.
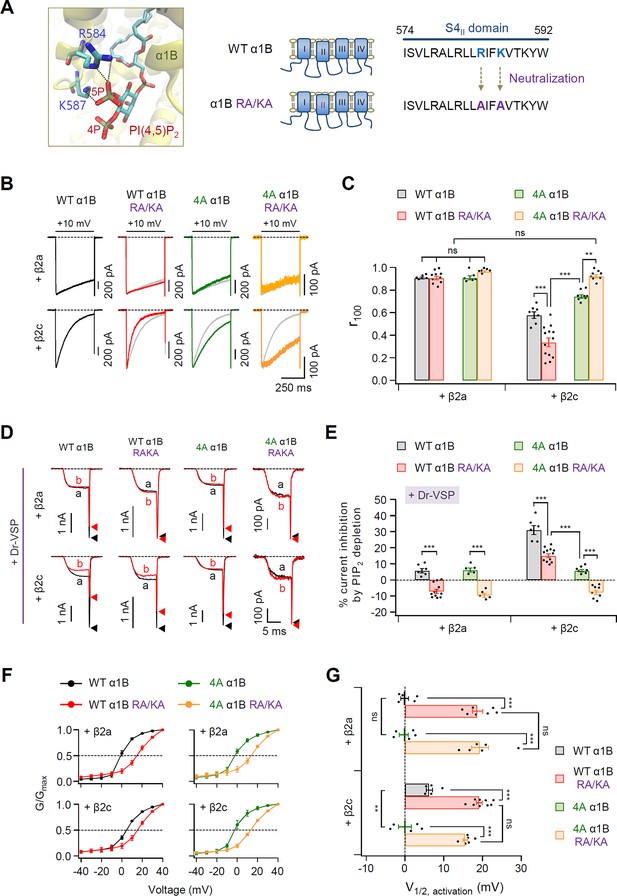
PIP2-binding residues within the S4II domain plays an important role in determining steady-state activation and PIP2 sensitivity of CaV2.2 channels.
(A) Distance analysis of PIP2-binding site in the S4II domain of α1B subunit. Two amino acids (R584 and K587) interacting with the 5-phosphate of PIP2 were neutralized to alanine residues (RA/KA). (B) Current inactivation was measured during 500-ms test pulses to +10 mV in cells expressing WT α1B (black traces), WT α1B RA/KA (red traces), 4A α1B (green traces), and 4A α1B RA/KA (orange traces) with β2a (upper) or β2c (bottom). Gray traces present the curve of WT α1B for comparison. (C) Summary of current inactivation of CaV2.2 channel in cells expressing indicated α1B with β2a (n = 5–10) or β2c (n = 7–13). The r100 indicates the fraction of current remaining after 100-ms depolarization to +10 mV. (D) Current inhibition by Dr-VSP-mediated PIP2 depletion in cells expressing WT α1B, WT α1B RA/KA, 4A α1B, and 4A α1B RA/KA with β2a (upper) or β2c (bottom) subunits. CaV2.2 currents before (a) and after (b) the depolarizing pulse to +120 mV are superimposed in Dr-VSP-expressing cells. Peak tail current is indicated by arrowheads (trace a, black head; trace b, red head). (E) Summary of the CaV2.2 current inhibition (%) by PIP2 depletion in cells expressing indicated α1B with β2a (n = 6–12) or β2c (n = 5–11). (F) The voltage dependence of normalized steady-state activation (G/Gmax) for WT α1B (black), WT α1B RA/KA (red), 4A α1B (green), and 4A α1B RA/KA (orange) with β2a (left) or β2c (right). Tail currents elicited between −40 and +40 mV in 10 mV steps, from a holding potential of −80 mV were normalized to the largest tail current in each series of test pulse. The curves were fitted by a Boltzmann function. Dashed line indicates the V1/2 of normalized steady-state activation. (G) Summary of the V1/2 of normalized steady-state activation in F (n = 5–9). Dots indicate the individual data points for each cell. Data are mean ± standard error of the mean (SEM). **p < 0.01, ***P<0.001, using two-way analysis of variance (ANOVA) followed by Sidak post-hoc test.
-
Figure 7—source data 1
Current inactivation (r100), current inhibition (%) by PIP2 depletion and the V1/2 of normalized steady-state activation in cells expressing WT α1B, WT α1B RA/KA, 4A α1B, and 4A α1B RA/KA with β2a or β2c.
- https://cdn.elifesciences.org/articles/69500/elife-69500-fig7-data1-v1.xlsx
Discussion
This study has expanded our understanding of the inter-regulatory actions of the CaV β subunit and membrane PIP2 on CaV channel gating properties, including inactivation kinetics, current density, and voltage dependency. Our data predict that CaV2.2 channels complexed with any β isotype can interact with membrane PIP2 through the binding pocket in the S4II domain (Figure 8). However, in CaV2.2 channels with cytosolic β2c, there seems to be another interaction with PIP2 through the nonspecific phospholipid-binding site at the distal end of the α1B I–II loop. This leads to the channel becoming highly sensitive to Dr-VSP-mediated PIP2 depletion (Figure 8, lower panel). In channels with β2a, the membrane anchoring of the subunit may interfere with the interaction between the phospholipid-binding site and PIP2. This converts the channels to a less PIP2-sensitive state (Figure 8, upper panel). Additionally, the neutralization of polybasic residues in the I–II loop to alanine abolished PIP2 binding on the phospholipid-binding site regardless of β isotype, which led to the less PIP2-sensitive state (Figure 8, 4A α1B). By contrast, the neutralization of two basic residues in the S4II-binding pocket slightly reduced PIP2 sensitivity in channels with cytosolic β2c subunits and completely abolished the response in channels with a β2a subunit (Figure 8, α1B RA/KA). Taken together, these data showed that when PIP2 molecules were depleted at the VSDII PIP2 and polybasic phospholipid-binding sites or both sites were mutated to neutralized amino acid residues, the channels move to a nonconducting state (Figure 8, 4A α1B RA/KA).
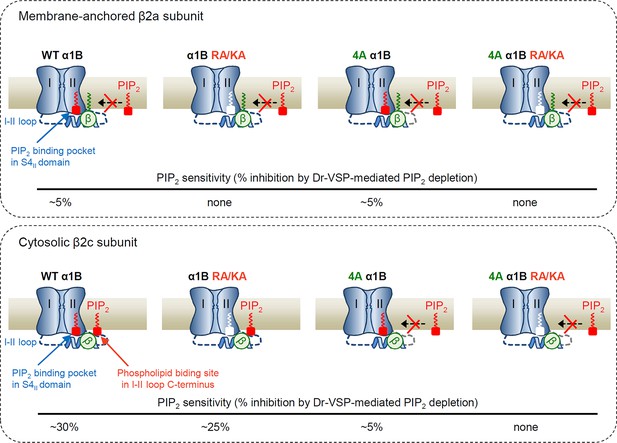
Schematic model showing the differential regulation of CaV2.2 channels with membrane-anchored and cytosolic β subunits by PIP2.
The channel possesses two distinct PIP2-interacting sites: the PIP2-binding pocket in the S4II domain and the nonspecific phospholipid-biding site in the I–II loop C-terminus. When the CaV2.2 channel is coupled with membrane-anchored β2a (upper panel), the proximal interaction of N-terminus of β2a with plasma membrane (PM) via its lipid anchor eliminates the binding of PIP2 to the polybasic phospholipid site on I–II loop, leading to the state less sensitive to PIP2 (upper left). In this condition, mutation of the PIP2-interacting phospholipid site in the I–II loop does not change the PIP2 sensitivity (upper 4A α1B). In contrast, when the CaV2.2 channel is coupled with cytosolic β2c (lower panel), there is no interaction of β subunit with the PM, leading to the higher PIP2-sensitive state through the association of the polybasic phospholipid-binding site with acid phospholipids in the PM (lower left). In 4A mutant channels, PIP2 interaction with the phospholipid-binding site is abolished, changing the channels to a state that shows only PIP2 binding to the binding pocket in S4II domain. PIP2 depletion in the PM or mutations of both PIP2-interacting sites alter channels to the nonconducting state by shifting the voltage-dependent activation to the depolarization direction (lower right). The approximate PIP2 sensitivity of each channel state in response to Dr-VSP activation is indicated as % inhibition at the bottom of each panel.
CaV β subunits regulate bidentate PIP2 binding to CaV2.2 channels
Previous studies have proposed a bidentate model for the PIP2 modulation of N-type CaV2.2 channel regulation (Heneghan et al., 2009; Hille et al., 2015; Mitra-Ganguli et al., 2009; Roberts-Crowley and Rittenhouse, 2009). In this model, lipidation on the N-terminus of CaV β subunits disrupts the hydrophobic interaction between the two fatty acyl chains of PIP2 and CaV2.2 channels, and thus reduces current inhibition by PIP2 depletion. For example, β2a subunits interact with the PM through two palmitoyl fatty acyl chains in the N-terminus, leading to competition in binding to CaV channels with the fatty side chains of PIP2. This competition removes PIP2 from the channel-binding site. Thus, CaV channels with β2a are uncoupled from the membrane PIP2 and show lower PIP2 sensitivity to PIP2 depletion. By contrast, non-lipidated β3 subunits expressed in the cytosol do not interrupt the interaction between the fatty acyl chains of PIP2 and CaV2.2 channels, and show high PIP2 sensitivity of channels (Heneghan et al., 2009; Hille et al., 2015; Suh et al., 2012). Consistently, we found that when the β3 subunits were anchored to the PM by adding the lipidation signal of Lyn to the N-terminus, the engineered Lyn-β3 construct decreased the PIP2 sensitivity of CaV2.2 channels, similar to β2a. The Lyn11 domain incorporates into the PM through the G2-myristoylated and C3-palmitoylated lipid anchors; therefore, Lyn-β3 mimics β2a in competing with PIP2 for the hydrophobic CaV2.2 channel interaction. Conversely, the lipid anchor of Lyn-48aa-β2c may be localized far from the channel complex because of its long N-terminal flexible linker, suggesting that these mutant subunits cannot disrupt the hydrophobic interaction between PIP2 and channels.
Our results provide advance information about the bidentate model. First, we confirmed that two distinct PIP2-interacting sites were preserved in the CaV2.2 channel: the binding pocket in VSDII and phospholipid-binding site in the I–II loop. Our data are consistent with that the 5-phosphate group of membrane PIP2 interacts with the two basic residues within the S4II domain of CaV2.2 channels regardless of β2 isotype. The additional interaction of PIP2 with the nonspecific phospholipid biding site in the distal I–II loop of CaV channels was mainly observed in CaV2.2 channels with the cytosolic CaV β2c subunit. Our data indicate that PIP2-binding to the I–II loop phospholipid-binding site is selectively disrupted by the lipid anchor of membrane-anchored β2a. The hydrophobic interaction of the palmitoyl or myristoyl groups of CaV β2a or Lyn-β constructs with channel complex may be the cause of PIP2 release from the lower-affinity I–II loop phospholipid-binding site (Roberts-Crowley and Rittenhouse, 2009). When PIP2 interacts with the VSDII PIP2-binding site of CaV2.2 channels complexed with β2a, the PIP2 sensitivity of the channels dramatically decreased to approximately 5%. Our results suggested that this minimal PIP2 sensitivity specifically caused by PIP2 degradation on VSDII-binding pocket by Dr-VSP activation.
This work suggests that the PIP2 sensitivity of the CaV2.2 channel is mainly affected by the length between the lipid anchor and GK domain of the CaV β subunit. Although both Lyn-β2c and Lyn-48aa-β2c are localized at the PM, the PIP2 sensitivity and inactivation kinetics of CaV2.2 channels are significantly different from each other: CaV2.2 channels with Lyn-β2c subunits exhibited relatively slower inactivation kinetics and lower PIP2 sensitivity, similar to channels with the membrane-anchored β2a subunit. By contrast, CaV2.2 channels with Lyn-48aa-β2c subunits exhibited faster inactivation kinetics and higher PIP2 sensitivity, similar to channels with the cytosolic β2c subunit. Similarly, disruption of the SH3-GK interaction in the membrane-anchored β2a subunit accelerated current inactivation and increased the current inhibition by PIP2 depletion. Moreover, real-time translocation of the lipid anchor, Lyn11, to the channel complex by rapamycin-inducible dimerization systems slowed the inactivation and decreased the PIP2 sensitivity of CaV2.2 channels. Inversely, incremental increases in flexible linker length between the lipid anchor and GK domain of CaV β2 subunits gradually accelerated the inactivation kinetics and increased the PIP2 sensitivity of CaV2.2 channels. However, the mechanism by which the physical distance from the PM lipid to GK domain of the CaV β subunit affects the PIP2 sensitivity of the CaV2.2 channel is not fully understood yet. Another possibility is that torsional rigidity of the linker domain may be different depending on the length and thus differently restrict the cytoplasmic movement of CaV β subunit as well as the gating of CaV2.2 channels.
Colecraft et al. have reported that chemically induced anchoring of intracellular loops of the channels to the PM can modulate the gating of the HVA Ca2+ channel (Subramanyam and Colecraft, 2015; Yang et al., 2013). They have shown that PdBu-induced translocation to the PM of chimeric β3-C1PKCγ, which is assembled by fusing the C1 domain of PKCγ to the C-terminus of the β3 subunit, leads to the inhibition of the CaV2.2 current. Conversely, the C1PKCγ-β3 subunit, which is assembled by adding C1PKCγ to the N-terminus of the β3 subunit, has no effect on the current (Yang et al., 2013). These studies suggest that the polarity of the PM-targeting domain may play an important role in determining the CaV2.2 channel gating; however, the molecular basis of the differential regulation mechanism remain unclear. On the basis of our results, we speculate that the C1PKCγ-β3 form may be insufficient to disrupt the interaction with between phospholipid-binding site and PIP2 in CaV2.2 channels because the length from the C1PKCγ and the GK domain of the β3 subunit is 175 aa. This could be too long to interfere the interaction between PIP2 and CaV2.2 channels.
Recently, Gao et al., 2021 have shown that two basic gating charge residues (R584 and K587) within the S4II domain of human CaV2.2 channel interact with the 5-phosphate group of membrane PIP2. In our present work, we found that mutation of the two residues (RA/KA) in the S4II domain completely blocked the Dr-VSP-induced current suppression in channels with β2a and shifted the voltage-dependent activation curve toward the depolarization direction regardless of CaV β2 isotype. The cryo-EM structure does not show the nonspecific PIP2-binding site in the channels probably because it is located in the flexible I–II loop. We hypothesize that the polybasic residues in the I–II loop tether to the anionic phospholipids through the electrostatic interaction and this dipole–dipole interaction may contribute to the low-affinity phospholipid-binding site (Yeon et al., 2018). In contrast, the VSDII PIP2-binding site forms a pocket-like structure inside the S4II domain and covered by the AID domain in the cytosolic side (Dong et al., 2021; Gao et al., 2021), which could stabilize the domain in a high-affinity PIP2 interacting site. Thus, it is possible that the PIP2 molecule inside the VSDII PIP2-binding pocket is relatively less accessible to the degradation by phospholipase C or Dr-VSP, leading to the lower PIP2 sensitivity in CaV2.2 channels.
In conclusion, our findings provide new insights on the regulatory mechanism of CaV2.2 channel gating by CaV β subunits. Our recent study has reported that when intracellular Ca2+ is increased by depolarizing the cells or activating Gq-coupled receptors, the high intracellular Ca2+ concentration induces a dissociation of the N-terminus of the CaV β2e subunit from the PM. This increases both the inactivation kinetics and PIP2 sensitivity of CaV2.2 channels (Kim et al., 2016). The N-terminus of the β2e subunit is anchored to the PM via electrostatic interaction with the anionic phospholipids of these PM. These studies suggest that dissociation of the β2e subunit from the membrane leads to an interaction between the I–II loop phospholipid-binding site and PIP2, which changes the gating properties of CaV channels in physiological conditions. The interaction of CaV α1B with β subunits can be dynamically exchanged by other free β isoforms in intact cells (Yeon et al., 2018); therefore, the displacement of cytosolic β subunits by membrane-tethered β subunits on CaV channels will abolish the interaction with between PIP2 and the I–II loop phospholipid-binding site via lipid anchor of membrane-tethered β subunits, which alters the CaV channel gating properties. Further studies are needed to investigate whether the conformational shift of the I–II loop to the membrane or cytosolic face by endogenous β subunit combinations determines CaV channel gating in neurons and other excitable cells.
Materials and methods
Cell culture and transfection
Request a detailed protocolHuman embryonic kidney tsA-201 cells (large T-antigen transformed HEK293 cells; RRID:CVCL_2737) were a kind gift from Dr Bertil Hille at University of Washington. The identity of this cell line has been authenticated by STR analysis and has recurrently tested negative for mycoplasma contamination using PCR (Cosmogenetech, Daejeon, South Korea). Cells were maintained in Dulbecco modified Eagle medium (Invitrogen, CA) supplemented with 10% fetal bovine serum (Invitrogen, CA) and 0.2% penicillin/streptomycin (Invitrogen, CA) in 100 mm culture dishes at 37°C with 5% CO2. The cells were transiently transfected with Lipofectamine 2000 (Invitrogen, CA) when the confluency of the cells reached 50–70%. For assessment of CaV channel expression, the cells were co-transfected with α1 of CaV, α2δ1, and various β2 chimera constructs in a 1:1:1 molar ratio. The transfected cells were plated onto a coverslip chip coated with poly-l-lysine (0.1 mg/ml, Sigma-Aldrich, MO) 24–36 hr after transfection. Plated cells were used for electrophysiological and confocal experiment within 24 hr after plating, as described previously (Park et al., 2017).
Plasmids
The following plasmids were used: The calcium channel subunits α1B of rat CaV2.2e[37b] (GenBank Sequence accession number AF055477) and rat α2δ1 (AF286488) were from Diane Lipscombe, Brown University, Providence, RI. Chimeric α1C-1B was generously donated by David T. Yue, Johns Hopkins University, Baltimore, MD. Mouse cDNAs of β2a and β2c were generously donated by Veit Flockerzi, Saarland University, Homburg, Germany. The Dr-VSP (AB308476) was obtained from Yasushi Okamura, Osaka University, Osaka, Japan.
Molecular cloning
Request a detailed protocolCloning of β2a-GFP, β2a(C3,4S)-GFP, and β2c-GFP was performed as previously described (Park et al., 2017). For the generation of various β2 chimera constructs, we used the one-step sequence- and ligation-independent cloning (SLIC) as a time-saving and cost-effective cloning strategy (Jeong et al., 2012). First, pEGFP-N1, pEYFP-N1, and mCherry-N1 vectors (Clontech) were linearized by KpnI restriction enzyme digestion. The cDNAs encoding β2a, β2c, Lyn, FRB, or FKBP were amplified by PCR using primers with an 18-bp homologous sequence attached to each end of the linearized vector. Primers used for β2 chimera constructs are listed in Supplementary file 1. Second, the linearized vector and PCR fragments were blended and incubated at room temperature for 2.5 min with T4 DNA polymerase (NEB, The Netherlands). Third, the DNA mixture was kept on ice for 10 min, after which competent Escherichia coli cells were transformed directly. For the deletion and point mutation of GK-SH3 interaction sites of the β2 subunit and the potential PIP2-interaction sites of α1B, first, the α1B or β2 subunits were amplified by inverse PCR using nPfu-special DNA polymerase (Enzynomics, Daejeon, South Korea). Second, the PCR product was 5′-phosphorylated by T4 polynucleotide kinase (Enzynomics, Daejeon, South Korea) and plasmid DNA was digested by Dpn I (Agilent Technologies, Santa Clara, CA). Finally, the PCR product was ligated by T4 DNA ligase (NEB, The Netherlands). The primers used for mutagenesis are listed in Supplementary file 2. All the chimera and mutant constructs were verified by DNA sequencing (Macrogen, South Korea).
Electrophysiology
Request a detailed protocolThe whole-cell configuration of the patch-clamp technique was used to record Ba2+ currents using HEKA EPC-10 patch-clamp amplifier with pulse software (HEKA Elektronik). Electrodes pulled from glass micropipette capillaries (Sutter Instrument) had resistances of 2–4 MΩ. The whole-cell access resistance was of 2–6 MΩ, and series resistance errors were compensated by 60%. For all recordings, cells were maintained at −80 mV. The external solution contained 10 mM BaCl2, 150 mM NaCl, 1 mM MgCl2, 10 mM HEPES, and 8 mM glucose, adjusted to pH 7.4 with NaOH and an osmolarity of 321–350 mOsm. The internal solution of the pipette consisted of 175 mM CsCl2, 5 mM MgCl2, 5 mM HEPES, 0.1 mM 1,2-bis(2-aminophenocy)ethane N,N,N′,N′-tetraacetic acid (BAPTA), 3 mM Na2ATP, and 0.1 mM Na3GTP, adjusted to pH 7.4 with CsOH and an osmolarity of 321–350 mOsm.
Confocal imaging
Request a detailed protocolAll imaging examinations were performed with an LSM 700 confocal microscope (Carl Zeiss AG) at room temperature (22–25℃). The external solution for confocal imaging contained 160 mM NaCl, 2.5 mM KCl, 2 mM CaCl2, 1 mM MgCl2, 10 mM HEPES, and 8 mM glucose, adjusted to pH 7.4 with NaOH and an osmolarity of 321–350 mOsm. For live-cell imaging, images were obtained by scanning cells with a ×40 (water) apochromatic objective lens at 1024 × 1024 pixels using digital zoom. Analysis of line scanning of fluorescence images was performed using the ‘profile’ tool in Zen 2012 lite imaging software (Carl Zeiss Microimaging). To analyze colocalization, we performed quantitative colocalization analysis using Fiji software with the Colocalization Threshold plugin to determine the Pearson’s correlation coefficient (R). Pixel intensities were presented as 2D intensity histograms with a linear regression line and as bar graphs with mean R values. All images were transferred from LSM4 to JPEG format.
Förster resonance energy transfer
Request a detailed protocolFRET experiments were performed using a monochromator (Polychrome V; TILL Photonics) with a ×40, NA 0.95 dry immersion objective lens (Olympus). Regular pulses of indigo light (438 ± 12 nm) excited the fluorescent proteins. Emission was separated into short (460–500 nm) and long (520–550 nm) wavelengths by appropriate filters and then acquired by two photomultipliers. Donor and acceptor signals obtained by photometry (TILL Photonics) were transferred to the data acquisition board (PCI-6221; National Instruments). Signal acquisition and real-time calculation of the FRET ratio were conducted by a custom program. The FRET ratio was calculated as follows:
CFPC is the CFP emission detected by the short-wavelength photomultiplier, and YFPC is the YFP emission detected by the long-wavelength photomultiplier, as described previously (Keum et al., 2014).
Calculation of distance with a WLC model
Request a detailed protocolThe Lyn-Linker-(additional Link) structure was suggested as an unstructured structure from the IUPRed Web-server (http://iupred.elte.hu/) (Dosztányi et al., 2005) to predict disorder tendency. To calculate the distance between the GK domain and the inner surface of the PM, the WLC model was used. This model is usually used to describe the behavior of polymers that are semi-flexible: quite stiff with successive segments pointing in roughly the same direction, and with persistence length within a few orders of magnitude of the polymer length. This model is also used to describe unstructured proteins like this linker structure (Zhou, 2001). In the WLC, the mean square end-to-end distance is written as:
where P is the polymer’s characteristic persistence length and is the maximum length. We used P = 0.6 and as (N − 1)*3.8, where N is number of amino acids in the unstructured protein (Lapidus et al., 2002). We then removed three amino acids in Lyn(MGC), which is directly connected to the membrane via palmitoylation and myristoylation. The root mean square end-to-end distance , which can be suggested as the average distance, was calculated. was 32.7 Å for six additional linkers, 36.0 Å for 11 aa, 38.4 Å for 15 aa, 42.4 Å for 22 aa, 52.5 Å for 43 aa, and 28.2 Å for no additional linker.
Statistical analysis
Request a detailed protocolPatch clamp data acquisition and analysis used Pulse/Pulse Fit 8.11 software with the EPC-10 patch clamp amplifier (HEKA Elektronik). Further data processing was performed with Igor Pro 6.2 (WaveMetrics, Inc), Excel office 365 (Microsoft), and GraphPad Prism 7.0 (GraphPad Software, Inc). All quantitative data were presented as mean ± standard error of the mean values. Comparisons between groups were analyzed by Student’s two-tailed unpaired t-test. Comparisons among more than two groups were analyzed using one-way analysis of variance (ANOVA) followed by Tukey post hoc test. Comparisons among more than two groups with two independent variables were analyzed using two-way ANOVA followed by Sidak post hoc test. Differences were considered significant at the *p < 0.05, **p < 0.01, and ***p < 0.001, as appropriate.
Data availability
All data generated or analyzed during this study are included in the manuscript and supporting files.
References
-
Characterization of the FKBP·rapamycin·FRB ternary complexJournal of the American Chemical Society 127:4715–4721.https://doi.org/10.1021/ja043277y
-
The versatility and universality of calcium signallingNature Reviews. Molecular Cell Biology 1:11–21.https://doi.org/10.1038/35036035
-
The ß subunit of voltage-gated ca2+ channelsPhysiological Reviews 90:1461–1506.https://doi.org/10.1152/physrev.00057.2009
-
Structure and function of the β subunit of voltage-gated ca2+ channelsBiochimica et Biophysica Acta 1828:1530–1540.https://doi.org/10.1016/j.bbamem.2012.08.028
-
Voltage-Gated calcium channelsCold Spring Harbor Perspectives in Biology 3:a003947.https://doi.org/10.1101/cshperspect.a003947
-
Identification of palmitoylation sites within the L-type calcium channel beta2a subunit and effects on channel functionThe Journal of Biological Chemistry 271:26465–26468.https://doi.org/10.1074/jbc.271.43.26465
-
Molecular regulation of voltage-gated Ca2+ channelsJournal of Receptor and Signal Transduction Research 25:57–71.https://doi.org/10.1081/rrs-200068102
-
The Ca2+ channel β subunit determines whether stimulation of Gq-coupled receptors enhances or inhibits N currentJournal of General Physiology 134:369–384.https://doi.org/10.1085/jgp.200910203
-
Phosphoinositides regulate ion channelsBiochimica et Biophysica Acta (BBA) - Molecular and Cell Biology of Lipids 1851:844–856.https://doi.org/10.1016/j.bbalip.2014.09.010
-
Regulation of voltage gated calcium channels by GPCRs and post-translational modificationCurrent Opinion in Pharmacology 32:1–8.https://doi.org/10.1016/j.coph.2016.10.001
-
One-Step sequence- and ligation-independent cloning as a rapid and versatile cloning method for functional genomics studiesApplied and Environmental Microbiology 78:5440–5443.https://doi.org/10.1128/AEM.00844-12
-
Dual regulation of R-type CaV2.3 channels by M1 muscarinic receptorsMolecules and Cells 39:322–329.https://doi.org/10.14348/molcells.2016.2292
-
A polybasic plasma membrane binding motif in the I-II linker stabilizes voltage-gated Cav1.2 calcium channel functionThe Journal of Biological Chemistry 290:21086–21100.https://doi.org/10.1074/jbc.M115.645671
-
Voltage-Dependent regulation of CaV2.2 channels by Gq-coupled receptor is facilitated by membrane-localized β subunitThe Journal of General Physiology 144:297–309.https://doi.org/10.1085/jgp.201411245
-
Dynamic phospholipid interaction of β2e subunit regulates the gating of voltage-gated ca2+ channelsThe Journal of General Physiology 145:529–541.https://doi.org/10.1085/jgp.201411349
-
Effects of chain stiffness on the dynamics of loop formation in polypeptides. Appendix: Testing a 1-dimensional diffusion model for peptide dynamicsThe Journal of Physical Chemistry B 106:11628–11640.https://doi.org/10.1021/jp020829v
-
A short polybasic segment between the two conserved domains of the β2a-subunit modulates the rate of inactivation of R-type calcium channelThe Journal of Biological Chemistry 287:32588–32597.https://doi.org/10.1074/jbc.M112.362509
-
Voltage-Sensing phosphatase: actions and potentialsThe Journal of Physiology 587:513–520.https://doi.org/10.1113/jphysiol.2008.163097
-
The hook region of voltage-gated Ca2+ channel β subunits senses and transmits PIP2 signals to the gateThe Journal of General Physiology 149:261–276.https://doi.org/10.1085/jgp.201611677
-
Arachidonic acid inhibition of L-type calcium (cav1.3b) channels varies with accessory cavbeta subunitsThe Journal of General Physiology 133:387–403.https://doi.org/10.1085/jgp.200810047
-
Dual regulation of voltage-sensitive ion channels by PIP (2)Frontiers in Pharmacology 3:170.https://doi.org/10.3389/fphar.2012.00170
-
Ion channel engineering: perspectives and strategiesJournal of Molecular Biology 427:190–204.https://doi.org/10.1016/j.jmb.2014.09.001
-
Pip2 is a necessary cofactor for ion channel function: how and why?Annual Review of Biophysics 37:175–195.https://doi.org/10.1146/annurev.biophys.37.032807.125859
-
Bio-inspired voltage-dependent calcium channel blockersNature Communications 4:14950–14957.https://doi.org/10.1038/ncomms3540
-
Loops in proteins can be modeled as worm-like chainsThe Journal of Physical Chemistry B 105:6763–6766.https://doi.org/10.1021/jp011355n
Article and author information
Author details
Funding
National Research Foundation of Korea (2020R1A6A3A01100500)
- Cheon-Gyu Park
National Research Foundation of Korea (2019R1A2B5B01070546)
- Byung-Chang Suh
National Research Foundation of Korea (2020R1A4A1019436)
- Byung-Chang Suh
The funders had no role in study design, data collection, and interpretation, or the decision to submit the work for publication.
Acknowledgements
We thank many laboratories for providing the plasmids.
Copyright
© 2022, Park et al.
This article is distributed under the terms of the Creative Commons Attribution License, which permits unrestricted use and redistribution provided that the original author and source are credited.
Metrics
-
- 1,296
- views
-
- 162
- downloads
-
- 1
- citation
Views, downloads and citations are aggregated across all versions of this paper published by eLife.