Octopamine drives honeybee thermogenesis
Abstract
In times of environmental change species have two options to survive: they either relocate to a new habitat or they adapt to the altered environment. Adaptation requires physiological plasticity and provides a selection benefit. In this regard, the Western honeybee (Apis mellifera) protrudes with its thermoregulatory capabilities, which enables a nearly worldwide distribution. Especially in the cold, shivering thermogenesis enables foraging as well as proper brood development and thus survival. In this study, we present octopamine signaling as a neurochemical prerequisite for honeybee thermogenesis: we were able to induce hypothermia by depleting octopamine in the flight muscles. Additionally, we could restore the ability to increase body temperature by administering octopamine. Thus, we conclude that octopamine signaling in the flight muscles is necessary for thermogenesis. Moreover, we show that these effects are mediated by β octopamine receptors. The significance of our results is highlighted by the fact the respective receptor genes underlie enormous selective pressure due to adaptation to cold climates. Finally, octopamine signaling in the service of thermogenesis might be a key strategy to survive in a changing environment.
Editor's evaluation
This study is of broad interest to researchers in the field of entomology and physiology. These findings may shed light on at least one mechanism underlying selective advantages conferred to insect species on evolutionary timescales. Though the chemical signal, its source, and recipient tissues underlying thermogenesis are elucidated, hypotheses regarding their downstream effects remain to be substantiated.
https://doi.org/10.7554/eLife.74334.sa0Introduction
The Western honeybee (Apis mellifera) owns incredible thermoregulation strategies, which allow the colony to keep the brood area constantly at 34 °C (Simpson, 1961). Due to this special feature, honeybees are relatively independent of the ambient temperature (TA), which may contribute decisively to their almost worldwide distribution (Wallberg et al., 2014). In contrast to other ectotherms, honeybee thermoregulation includes thermogenesis. Here, primarily workerbees actively increase their thorax temperatures (TTHX, Kovac et al., 2009; Stabentheiner et al., 2010). This thermogenesis is of immense social importance, because it enables foraging at TA below 10 °C (Bujok et al., 2002; Stabentheiner et al., 2003) and a proper brood development (Himmer, 1932; Weiss, 1962; Tautz et al., 2003; Wang et al., 2016), reduces parasite infections (Starks et al., 2000; Campbell et al., 2010), and is a powerful defense mechanism against predatory hornets (Ken et al., 2005; Baracchi et al., 2010).
The individual heating pattern of workerbees consists of a wave-like rise and fall in TTHX (Kronenberg and Heller, 1982) and is realized exclusively by the activation of the indirect flight muscles, formed by the dorsoventral wing elevators (DV) and the dorsal-longitudinal wing depressors (DL), even if wing and thorax vibration are not visible (Esch et al., 1991; Esch and Goller, 1991). However, these muscles are utilized in various other behaviors, which includes flight (Esch et al., 1975; Esch, 1976), fanning (Simpson, 1961) and communication during the waggle dance (Esch, 1961; Wenner, 1962). In order to perform these various tasks, diverse contraction mechanisms exist which must be controlled differently (Esch and Goller, 1991). Some evidence indicates a crucial role of octopamine in the insect flight muscles (Blau and Wegener, 1994; Blau et al., 1994; Wegener, 1996; Duch et al., 1999). Unfortunately, it remains unknown whether octopamine is used as a neurochemical in honeybee flight muscles or whether an octopamine receptor gene is expressed in these tissues. However, DL and DV are under control of the mesometa-thoracic ganglion (MMTG, Markl, 1966) and the octopaminergic innervation of the flight muscles seems to be a conserved feature in insects (Duch et al., 1999; Schlurmann and Hausen, 2003; Pauls et al., 2018). It was further demonstrated that the brain octopamine concentration of workerbees is significantly decreased due to cold stress (Chen et al., 2008), which indicates the temperature sensitivity of the neuronal octopaminergic system. In this context, Wallberg et al., 2017 made the observation that honeybee β octopamine receptor genes (AmOARβ1-3/4) are subject to altitudinal adaptation processes in honeybees. Yet, the physiological significance of this result has not been investigated so far. One important parameter that decreases significantly with increasing altitude is TA. Consequently, honeybee thermogenesis is essential for colony survival, and the adaptive pressure on AmOARβ1-3/4 may indicate the involvement of octopamine in this process.
We hypothesize that honeybee thermogenesis relies on octopamine signaling and that β octopamine receptors are crucially involved in this process. We have investigated systematically the honeybee thoracic octopaminergic system. Moreover, we have tested our hypothesis and we can show that octopamine promotes thermogenesis by directly affecting the flight muscles.
Results
Honeybee flight muscles are innervated by octopaminergic neurons
First of all, we investigated whether octopamine can be a potential regulator of flight muscle functions in honeybees. Thus, we analyzed which monoamines are actually present in these tissues using high-performance liquid chromatography (HPLC) together with an electrochemical detector (ECD). We can detect octopamine and dopamine in both, DV and DL, whereas serotonin and tyramine are not detectable (Figure 1A, Figure 1—figure supplement 1A). We further compared the flight muscle octopamine concentration in differently aged workerbees. Newly emerged bees which cannot perform thermogenesis have the lowest octopamine concentration in DV and DL (Figure 1A–B) and the octopamine concentration increases with the age of the workerbee (Figure 1A–B). In contrast to octopamine, the concentrations of dopamine have a different time course in DV and DL (Figure 1—figure supplement 1B-C). We have further analyzed the MMTG. In addition to octopamine, serotonin, dopamine, and tyramine are also detectable, but no age-related differences can be observed for any of these monoamines (Figure 1C, Figure 1—figure supplement 1D-F).
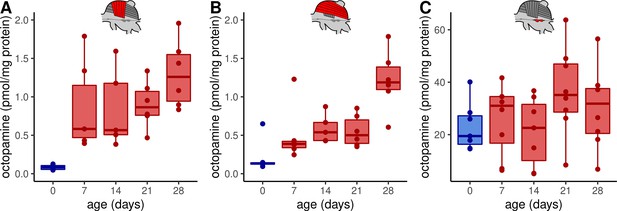
Octopamine concentrations in thoracic tissues across age.
Octopamine concentrations differ significantly between different workerbee age groups in DV (A) and DL (B) but not in the MMTG (C). blue = no active heating, red = active heating. Shown is median ± interquartile range (IQR). For statistics see Table 1.
Statistical analysis of HPLC analysis of the octopamine content.
ns = not significant.
Analysis | Test | Groups (n) | Result | |
---|---|---|---|---|
HPLCoctopamineDVFigure 1A | Kruskal-Wallis test | χ2 = 15.772, df = 4, p = 0,0033 | ** | |
Dunns test | 0 days (6) vs. 7 days (6) | Z = –2.4593, padj = 0.1392 | ns | |
0 days (6) vs. 14 days (6) | Z = –2.8856, padj = 0.0391 | * | ||
0 days (6) vs. 21 days (6) | Z = –2.7217, padj = 0.065 | ns | ||
0 days (6) vs. 28 days (6) | Z = –3.7382, padj = 0.0017 | ** | ||
7 days (6) vs. 14 days (6) | Z = 0.4263, padj = 1.0 | ns | ||
7 days (6) vs. 21 days (6) | Z = 0.2623, padj = 1.0 | ns | ||
7 days (6) vs. 28 days (6) | Z = 1.2789, padj = 1.0 | ns | ||
14 days (6) vs. 21 days (6) | Z = 0.164, padj = 1.0 | ns | ||
14 days (6) vs. 28 days (6) | Z = –0.8526, padj = 1.0 | ns | ||
21 days (6) vs. 28 days (6) | Z = –1.0165, padj = 1.0 | ns | ||
HPLCoctopamineDLFigure 1B | Kruskal-Wallis test | χ2 = 16.292, df = 4, p = 0.0027 | ** | |
Dunns test | 0 days (6) vs. 7 days (6) | Z = –1.3117, padj = 1.0 | ns | |
0 days (6) vs. 14 days (6) | Z = –2.6561, padj = 0.0791 | * | ||
0 days (6) vs. 21 days (6) | Z = –1.9019, padj = 0.5718 | ns | ||
0 days (6) vs. 28 days (6) | Z = –3.8038, padj = 0.0014 | ** | ||
7 days (6) vs. 14 days (6) | Z = 1.3444, padj = 1.0 | ns | ||
7 days (6) vs. 21 days (6) | Z = 0.5902, padj = 1.0 | ns | ||
7 days (6) vs. 28 days (6) | Z = 2.4921, padj = 0.127 | ns | ||
14 days (6) vs. 21 days (6) | Z = 0.7542, padj = 1.0 | ns | ||
14 days (6) vs. 28 days (6) | Z = –1.1477, padj = 1.0 | ns | ||
21 days (6) vs. 28 days (6) | Z = –1.9019, padj = 0.5718 | ns | ||
HPLCoctopamineMMTGFigure 1C | Kruskal-Wallis test | χ2 = 5.4912, df = 4, p = 0.2405 | ns | |
groups (n): 0 days (7), 7 days (8), 14 days (7), 21 days (8), 28 days (8) | ||||
Nerves originating from the MMTG exclusively innervate the honeybee flight muscles (Markl, 1966; Pan, 1980). To answer whether octopamine in DV and DL can be delivered directly by octopaminergic neurons from the MMTG we used an octopamine specific antibody to analyze the octopamine distribution in these tissues. Octopamine-like immunoreactivity (OA-IR) is observable in four individual cell clusters, with most of the cell bodies being found at the ventral midline (Figure 2A–E). Some OA-IR positive cell bodies are also located at the dorsal midline (Figure 2D–E). Most MMTG leaving nerves show OA-IR (Figure 2G–I), as varicose fibers in IIN1 and a thicker axonal bundle in IIN3 demonstrate (Figure 2G1). Finally, finest OA-IR positive varicose structures can be found directly at muscle fibers (Figure 2J–K).
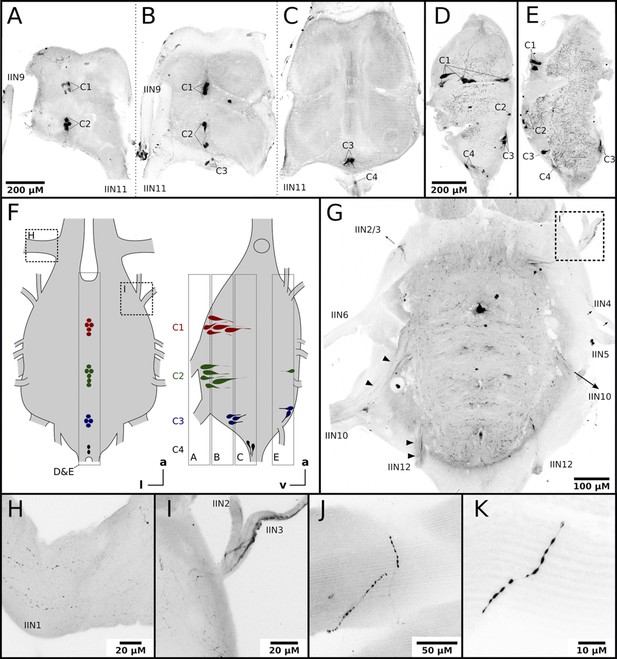
Honeybee flight muscles are innervated by octopaminergic neurons.
(A–E) Different cell clusters with OA-IR are observable. Consecutive frontal sections of the MMTG of the same workerbee (A–C) beginning with the most ventral section (A) showing clusters of OA-IR positive cells (C1–C4). Sagital sections (D–E) in the midline area of the MMTG of two individual bees display the same OA-IR positive cell clusters. (F) Schematic interpretation of the location of the cell clusters found in A-E. Additionally, the approximate location of frontal sections (A–C, G), the sagital sections (D–E), and the detailed images (H–I) are indicated by dashed boxes. (G) Dorsally located frontal section of the MMTG in showing several nerves which are leaving the ganglion. Strong OA-IR-positive fibers run into the nerves IIN3, IIN10, and IIN12 (arrowheads). (H) Within the nerve IIN1 fine varicose structures with OA-IR are observable. (I) An OA-IR-positive axon bundle runs through the nerve IIN3. (J–K) Flight muscle preparations reveal fine varicose structures with OA-IR closely attached to muscle fibers.
AmOARβ2 is expressed in the flight muscles
We next determined which octopamine receptor genes are expressed in the workerbee flight muscle. The honeybee genome harbors five different genes that code for octopamine receptors and two additional genes encoding tyramine receptors. The respective receptor proteins are functionally characterized (Blenau et al., 2000; Grohmann et al., 2003; Balfanz et al., 2014; Reim et al., 2017; Blenau et al., 2020). We observe strong signals for PCR products for AmOARα1 and AmOARβ2, weak DNA bands for AmOARβ1 and AmOARβ3/4, and no amplification product in the case of AmOARα2 and both tyramine receptor genes (AmTAR1 & AmTAR2; Figure 3A). In addition, PCR products indicate the expression for all known honeybee octopamine and tyramine receptor genes in neural tissues (brain, MMTG).
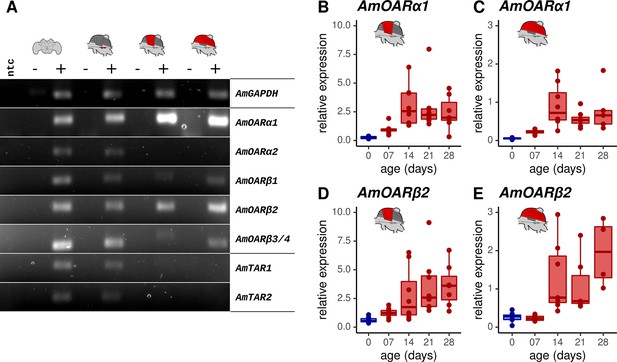
Octopamine receptor expression in the flight muscles.
(A) Brain, MMTG, DV, and DL were manually dissected from workerbees and underwent subsequent RNA isolation, cDNA synthesis and PCR analysis (+). The reverse transcriptase was omitted during cDNA synthesis for negative controls (-). RNase free water serves as no template (ntc) and AmGAPDH as loading control. (B–E) AmOARα1 and AmOARβ2 expression in DV and DL of differential aged workerbees with (red) or without (blue) the capability for thermogenesis. Data are represented as boxplots. Shown is median ± IQR. For statistic see Table 2.
-
Figure 3—source data 1
Labelled original files of the full raw unedited PCR gels.
- https://cdn.elifesciences.org/articles/74334/elife-74334-fig3-data1-v1.zip
-
Figure 3—source data 2
Unlabelled original files of the full raw unedited PCR gels.
- https://cdn.elifesciences.org/articles/74334/elife-74334-fig3-data2-v1.zip
Statistical analysis of the flight muscle gene expression analysis.
ns = not significant.
Analysis | Test | Groups (n) | Result | |
---|---|---|---|---|
qPCR AmOARα1 DV Figure 3D | Kruskal-Wallis test | χ2 = 25.734, df = 4, p = 0.00004 | *** | |
Dunns test | 0 days (8) vs. 7 days (8) | Z = –1.6253, padj = 1.0 | ns | |
0 days (8) vs. 14 days (8) | Z = –3.9776, padj = 0.0007 | *** | ||
0 days (8) vs. 21 days (8) | Z = –3.9135, padj = 0.0009 | *** | ||
0 days (8) vs. 28 days (8) | Z = –3.8493, padj = 0.0012 | ** | ||
7 days (8) vs. 14 days (8) | Z = –2.3523, padj = 0.1866 | ns | ||
7 days (8) vs. 21 days (8) | Z = –2.2882, padj = 0.2213 | ns | ||
7 days (8) vs. 28 days (8) | Z = –2.224, padj = 0.2615 | ns | ||
14 days (8) vs. 21 days (8) | Z = 0.0642, padj = 1.0 | ns | ||
14 days (8) vs. 28 days (8) | Z = 0.1283, padj = 1.0 | ns | ||
21 days (8) vs. 28 days (8) | Z = 0.0642, padj = 1.0 | ns | ||
qPCR AmOARα1 DL Figure 3C | Kruskal-Wallis test | χ2 = 28.163, df = 4, p = 0.00001 | *** | |
Dunns test | 0 days (8) vs. 7 days (8) | Z = –1.5661, padj = 1.0 | ns | |
0 days (8) vs. 14 days (8) | Z = –4.4373, padj = 0.0001 | *** | ||
0 days (8) vs. 21 days (7) | Z = –3.6548, padj = 0.0026 | ** | ||
0 days (8) vs. 28 days (5) | Z = –3.7128, padj = 0.002 | ** | ||
7 days (8) vs. 14 days (8) | Z = –2.8712, padj = 0.0409 | * | ||
7 days (8) vs. 21 days (7) | Z = –2.1418, padj = 0.322 | ns | ||
7 days (8) vs. 28 days (5) | Z = –2.3392, padj = 0193 | ns | ||
14 days (8) vs. 21 days (7) | Z = 0.6320, padj = 1.0 | ns | ||
14 days (8) vs. 28 days (5) | Z = 0.179, padj = 1.0 | ns | ||
21 days (7) vs. 28 days (5) | Z = –0.3844, padj = 1.0 | ns | ||
qPCR AmOARβ2 DV Figure 3D | Kruskal-Wallis test | χ2 = 24.54, df = 4, p = 0.00006 | *** | |
Dunns test | 0 days (8) vs. 7 days (8) | Z = –1.6894, padj = 0,911 | ns | |
0 days (8) vs. 14 days (8) | Z = –2.8228, padj = 0.0476 | * | ||
0 days (8) vs. 21 days (8) | Z = –3.8707, padj = 0.0011 | ** | ||
0 days (8) vs. 28 days (8) | Z = –4.3412, padj = 0.0001 | *** | ||
7 days (8) vs. 14 days (8) | Z = –1.1334, padj = 1.0 | ns | ||
7 days (8) vs. 21 days (8) | Z = –2.1813, padj = 0.292 | ns | ||
7 days (8) vs. 28 days (8) | Z = –2.6517, padj = 0.0801 | ns | ||
14 days (8) vs. 21 days (8) | Z = –1.0479, padj = 1.0 | ns | ||
14 days (8) vs. 28 days (8) | Z = –1.5183, padj = 1.0 | ns | ||
21 days (8) vs. 28 days (8) | Z = –0.4705, padj = 1.0 | ns | ||
qPCR AmOARβ2 DL Figure 3E | Kruskal-Wallis test | χ2 = 24.737, df = 4, p = 0.00006 | *** | |
Dunns test | 0 days (8) vs. 7 days (8) | Z = 0.5429, padj = 1.0 | ns | |
0 days (8) vs. 14 days (7) | Z = –2.9652, padj = 0.0302 | * | ||
0 days (8) vs. 21 days (6) | Z = –2.4814, padj = 0.130 | ns | ||
0 days (8) vs. 28 days (4) | Z = –3.1454, padj = 0.0166 | * | ||
7 days (8) vs. 14 days (7) | Z = –3.4897, padj = 0.0048 | ** | ||
7 days (8) vs. 21 days (6) | Z = –2.9841, padj = 0.0284 | * | ||
7 days (8) vs. 28 days (4) | Z = –3.5887, padj = 0.0033 | ** | ||
14 days (7) vs. 21 days (6) | Z = 0.3496, padj = 1.0 | ns | ||
14 days (7) vs. 28 days (4) | Z = –0.6246, padj = 1.0 | ns | ||
21 days (6) vs. 28 days (4) | Z = –0.9079, padj = 1.0 | ns |
We further determined the relative gene expression of the most promising candidates by quantitative Real Time PCR (qPCR, Figure 3B–E). AmOARα1 and AmOARβ2 expression can be observed in DV and DL in all age groups of workerbees. Here, relative expression increases with age, as shown by significant differences between newly emerged bees (0 days) and the three oldest groups.
Octopamine is mandatory for honeybee thermogenesis
To investigate the consequences of octopamine missing in the flight muscles, we fed workerbees with reserpine. This drug has the ability to deplete vesicles on monoaminergic synapses (Plummer et al., 1954; Cheung and Parmar, 2020). The octopamine concentrations in DV and DL are significantly decreased due to our treatment (Figure 4A–B). In contrast, the dopamine concentration in the flight muscle seems not to be affected (Figure 4—figure supplement 1). The same is true for the concentrations of octopamine (Figure 4C) and of the other monoamines in the MMTG (Figure 4—figure supplement 1).
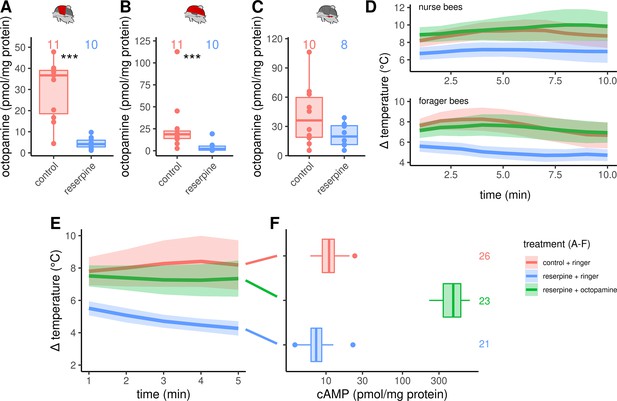
Octopaminergic control of honeybee thermogenesis.
(A–C) Octopamine concentrations are decreased in DV and DL but not MMTG of reserpinezed workerbees. Data are represented as boxplots. Shown is median ± IQR. Mann-Whitney U test, DV(A): W = 105, z = –3.70, p < 0.001; DL(B): W = 102, z = –3.37, p < 0.001; MMTG(C): W = 56, z = –0.94, p = 0.1728. (D) Reserpinezed bees show hypothermia when compared with control. An injection of octopamine into the flight muscle helps the bees to recover, as no differences are observable between the control group and the recovered bees. The solid line represents the mean difference between TTHX and TA and the shaded area represents the 95% confidence interval. For statistic see Table 3. (E) Similar experiment as in (D) but bees were frozen in liquid N2 after 5 min for cAMP quantification. For statistic see Table 3. (F) The tissue cAMP concentrations in the flight muscles differ significantly due the treatment (Kruskal-Wallis test, X2 = 52.636, df = 2, p < 0.001). Reserpinezed bees has the lowest tissue cAMP concentrations in the flight muscles when compared with controls (Dunns test, Z = 2.6383, padj = 0.025) and recovered bees (Z = 7.117, padj= < 0.001). Controls also differ from the recovered bees (Z = –4.7998, padj <0.001). Data are represented as boxplots. Shown is median ± IQR.
Statistical analysis of the thermogenesis dependent on the pharmacological treatment.
c = control, r = reserpine, ATS = ANOVA type statistic, ns = not significant.
Experiment | Groups (n) | ATS | Df | p | |
---|---|---|---|---|---|
ReserpineNurse beesFigure 4D | 9.3635 | 1.9854 | 0.00009 | *** | |
c + ringer (21) vs. r + ringer(23) | 13.9618 | 1.0 | 0.0002 | *** | |
c + ringer (21) vs. r + octopamine (23) | 0.0952 | 1.0 | 0.7577 | ns | |
r + ringer(23) vs. r + octopamine (23) | 14.2223 | 1.0 | 0.0002 | *** | |
ReserpineForager beesFigure 4D | 14.5704 | 1.9437 | 0.0000006 | *** | |
c + ringer (29) vs. r + ringer(28) | 126.5492 | 1.0000 | 0.0000003 | *** | |
c + ringer (29) vs. r + octopamine (29) | 0.0753 | 1.0 | 0.7838 | ns | |
r + ringer(28) vs. r + octopamine (29) | 21.1833 | 1.0000 | 0.000004 | *** | |
ReserpinecAMPQuantificationFigure 4E | 22.8759 | 1.8981 | 0,0000000003 | *** | |
c + ringer (26) vs. r + ringer(21) | 39.9913 | 1.0000 | 0.0000000003 | *** | |
c + ringer (26) vs. r + octopamine (23) | 0.1155 | 1.0 | 0.734 | ns | |
r + ringer(21) vs. r + octopamine (23) | 37.3015 | 1.0000 | 0.000000001 | *** | |
MianserinNurse beesForager beesFigure 5A | |||||
control (30) vs. mianserin (30) | 9.2737 | 1.0000 | 0.0023 | ** | |
control (30) vs. mianserin (30) | 8.4638 | 1.0000 | 0.0036 | ** | |
YohimbineNurse beesForager beesFigure 5B | |||||
control (30) vs. yohimbine (30) | 0.8011 | 1.0000 | 0.3708 | ns | |
control (32) vs. yohimbine (33) | 0.0584 | 1.0000 | 0.8091 | ns | |
AlprenololNurse beesForager beesFigure 5C | |||||
control (30) vs. alprenolol (30) | 7.5516 | 1.0000 | 0.0059 | ** | |
control (34) vs. alprenolol (33) | 10.9721 | 1.0000 | 0.0009 | *** | |
CarvedilolNurse beesForager bees | |||||
control (30) vs. carvedilol (30) | 0.1235 | 1.0000 | 0.7252 | ns | |
control (36) vs. carvedilol (34) | 0.2650 | 1.0000 | 0.6067 | ns | |
MetoprololNurse beesForager bees | |||||
control (30) vs. metoprolol (30) | 0.1031 | 1.0000 | 0.7481 | ns | |
control (36) vs. metoprolol (36) | 0.2029 | 1.0000 | 0.6524 | ns | |
Rp-8-CPT-cAMPSNurse beesForager beesFigure 6A | |||||
control (25) vs. Rp-8-CPT-cAMPS (23) | 4.062 | 1.0000 | 0.044 | * | |
control (15) vs. Rp-8-CPT-cAMPS (14) | 27.7439 | 1.0000 | 0.0000001 | *** | |
The reserpine feeding additionally causes hypothermia in both, nurse bees and forager bees (Figure 4D, Table 3). A preliminary screen with serotonin, dopamine, octopamine and tyramine revealed, that octopamine may reverse the reserpine effect (Figure 4—figure supplement 3). We were able to show that this octopamine effect is robust. We reversed the reserpine-induced hypothermia by injecting octopamine directly into the flight muscles (Figure 4, Table 3).
As stated above, we hypothesize that β octopamine receptors are crucially involved in honeybee thermogenesis. Via Gαs proteins, these receptors are positively coupled to membrane-bound adenylyl cyclases (mAC), which leads to an increase of the intracellular adenosine 3’,5’-cyclic mono-phosphate (cAMP) concentration upon receptor activation (Balfanz et al., 2014). To control our hypothesis, we have repeated the reserpine experiment reported above. The reserpine induced hypothermia as well as the octopamine reversion of this effect are again clearly observable (Figure 4E, Table 3). We stopped thermography after 5 min and the bees were immediately flash-frozen to subsequently quantify the tissue cAMP concentrations of their flight muscles. The tissue cAMP concentration is significant lower in reserpinized bees when compared with control (Figure 4D). Furthermore, octopamine injection into the flight muscles of reserpinized bees leads to a strong increase of the tissue cAMP concentration (Figure 4D). The tissue guanosine 3’,5’-cyclic monophosphate (cGMP) concentrations seem not to be affected by our treatment (Figure 4—figure supplement 4). Further cyclic nucleotides in the flight muscles were either below the lower limit of quantification (cytidine 3’,5’-cyclic monophosphate, cCMP) or were not detectable at all.
Octopamine receptor antagonists also induce hypothermia
Next, we aimed to confirm the described effects of octopamine on honeybee thermogenesis and also to further narrow down the responsible receptor subtypes. Therefore, we injected different pharmacological substances directly into the flight muscles and analyzed their effect on thermogenesis. These substances antagonize various octopamine, tyramine, or adrenergic receptors. All antagonists either lead to hypothermia in both, nurse bees and forager bees, or they are not effective at all. The non-selective but potent octopamine receptor antagonist mianserin leads to hypothermia (Figure 5A, Table 3), while the effective tyramine receptor and octopamine receptor antagonist yohimbine does not (Figure 5B, Table 3). Finally, alprenolol causes hypothermia too (Figure 5C, Table 3), whereas carvedilol and metoprolol did not have an observable effect on thermogenesis (Table 3).
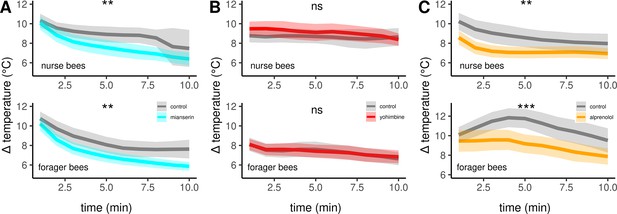
The effects of different antagonists on workerbee thermogenesis.
Mianserin (A) and alprenolol (C) cause hypothermia in workerbees but not yohimbine (B). The solid line represents the mean difference between TTHX and TA and the shaded area represents the 95% confidence interval. For statistic see Table 3.
Downstream metabolic pathway analyses points to glycolysis
In a final experiment series, we investigated the signaling pathway downstream of octopamine receptors in more detail. Up this point, our results indicate the activation of β octopamine receptors, leading to an increase in cAMP concentration. This second messenger has the potential to activate protein kinase A (PKA). To test whether PKA is directly involved in the cellular pathway that enables thermogenesis, we used Rp-8-CPT-cAMPS which is a potent, metabolically stable and membrane-permeable inhibitor of PKA (Dostmann et al., 1990; Gjertsen et al., 1995). Rp-8-CPT-cAMPS negatively effects thermogenesis in both, nurse bees and forager bees (Figure 6A). Furthermore, we wanted to know whether octopamine release, which most likely activates PKA, could stimulate glycolysis. To test this hypothesis, we quantified pyruvate concentration in DL muscles after octopamine stimulation. Pyruvate is formed in the final step of glycolysis and its metabolites are further catabolized in the tricarboxylic acid cycle (Zhang et al., 2019). Pyruvate concentrations increase significantly after octopamine stimulation (Figure 6B). Finally, we observed that the AmGAPDH gene shows increased expression triggered by cold stress (Figure 6C). This gene encodes glyceraldehyde 3-phosphate dehydrogenase which converts glyceraldehyde 3-phosphate to D-glycerate 1,3-bisphosphate during glycolysis. A similar increase in AmGAPDH expression can be observed when the bees were treated with an octopamine injection in to the flight muscles instead of cold stress. (Figure 6D).
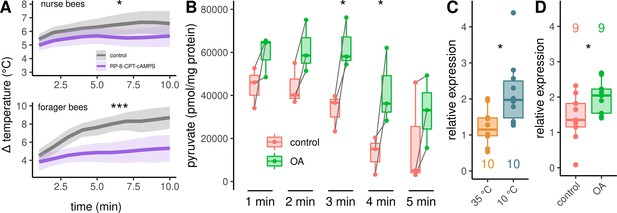
Analysis of the downstream pathway.
(A) The PKA inhibitor Rp-8-CPT-cAMPS causes hypothermia in nurse bees and forager bees. The solid line represents the mean difference between TTHX and TA and the shaded area represents the 95% confidence interval. For statistic see Table 3. (B) DL muscles were separated into two mirror-similar parts and treated differently. Bath application of octopamine (B) leads to an difference in the mean pyruvate concentration when compared with control (two-way RM ANOVA, F(1)=38.28, p < 0.001). The simple main effect of treatment becomes significant after 3 and 4 min (Sidaks multiple comparisons test, 3 min: p = 0.016, 4 min: p = 0.017). Shown is median ± IQR. Data points of the same individual are connected by gray lines. (C–D) AmGAPDH expression in DV and DL is upregulated due to cold stress (C, Mann-Whitney U test, W = 83, z = –2.24, p = 0.01261). This result can be mimicked by an injection of octopamine directly into the flight muscles (D, Mann-Whitney U test, W = 18, z = –1.68, p = 0.04694). Shown is median ± IQR.
Discussion
In this study, we hypothesized that octopamine has a critical role in the shivering thermogenesis of honeybees. An important prerequisite is that this monoamine can be used as a neurochemical substance at the flight muscles, which seems to be a conserved feature in insects (Duch et al., 1999; Schlurmann and Hausen, 2003; Pauls et al., 2018). We can demonstrate that octopamine is present in workerbee flight muscles by independent analysis methods (HPLC-ECD, antibody labeling). This is most likely delivered via flight muscle innervating neurons from the MMTG. Here, we can detect four octopaminergic cell clusters. Those are known to derive from a single median neuroblast at the posterior border of each segment of the developing neuroectoderm and are then displaced during the fusion of ganglia to the dorsal or the ventral surface (Bräunig and Pflüger, 2001). We postulate that the octopaminergic cells in each cluster we found are descendants of individual neuroblasts of their neuromere. The honeybee MMTG is formed by fusion of four neuromers (mesothorax, methathorax, first and second abdominal ganglia; Markl, 1966). Furthermore, the MMTG nerves IIN1 and IIN3 innervate DV and DL, respectively (Markl, 1966; Pan, 1980), while some of their neuronal structures contain octopamine. Finally, they reach DV and DL as octopaminergic varicosities suggest. We conclude, that octopaminergic neurons from the MMTG directly innervate the flight muscles and therefore influence thermogenesis.
If this is true, octopamine should be detectable at comparable concentrations in the flight muscles of workerbees capable of thermogenesis. Indeed, we found no differences in bees with ages ranging from 7 days up to 4 weeks. They are all similarly engaged in active heat production independent of their actual task within the colony (Stabentheiner et al., 2010). In contrast, newly emerged bees, which are not capable of heat production (Harrison, 1987; Stabentheiner et al., 2010), have significant lower flight muscle octopamine concentrations. It remains uncertain whether there is a causal relationship between the low octopamine concentrations and the absence of thermogenesis in newly emerged bees or whether this observation is merely a correlation. Several factors could be responsible, such as incomplete differentiation of flight muscle tissues (Roberts and Elekonich, 2005; Correa-Fernandez and Cruz-Landim, 2010).
We determined AmOARα1 and AmOARβ2 as the predominant octopamine receptor genes expressed in the flight muscle, and their expression is detectable across age. The relative expression of both genes is higher in older bees, but at the same time a huge inter-individual variation is detectable. This might reflect differential demands to muscle activity in the context of the age-dependent task allocation and its neurochemical control. Workerbees perform very different tasks as a function of their age (Seeley, 1995). Yet, they are all similarly engaged in heat production if they are older than two days (Stabentheiner et al., 2010). Besides flight and thermogenesis another important function of the flight muscles is fanning for cooling purposes (Hess, 1926; Hazelhoff, 1954; Simpson, 1961) and octopamine is known to increase the probability of fanning when fed to workerbees together with tyramine (Cook et al., 2017). The two genes AmOARα1 and AmOARβ2 encode the octopamine receptor proteins AmOARα1 (Grohmann et al., 2003) and AmOARβ2 (Balfanz et al., 2014), respectively. We assume, that both receptors can receive and forward the signal mediated by an octopamine release at the flight muscles. Until now, we did not know in which situations this occurs and what specific role the corresponding receptors might have in this process.
Our reserpine experiments solve this problem, because it makes octopamine no longer usable at the flight muscle. As direct consequence, we observe hypothermia. Moreover, if we supply the system with octopamine again we can restore heat generation. We conclude that octopamine signaling is necessary for honeybee thermogenesis. This interpretation is supported by the fact that the potent octopamine receptor antagonist mianserin (Grohmann et al., 2003; Balfanz et al., 2014; Blenau et al., 2020) causes hypothermia, too. Moreover, our cAMP quantification result suggests that at least one β octopamine receptor subtype mediates the octopamine signal in the service of thermogenesis. The decreased octopamine availability in the flight muscles of reserpinezed bees likely causes the loss of octopamine release if necessary. In the end, this results in a reduction of octopamine receptor activation events. In the case of β octopamine receptors, consequently, no cAMP is produced. Indeed, we observe a decrease in tissue cAMP concentrations in combination with reserpine induced hypothermia. Octopamine-induced reversal of this effect is accompanied by a tremendous increase in tissue cAMP concentrations. Unfortunately, honeybee cAMP concentrations from muscle tissues are not available, but our results are consistent with analysis in locust flight muscle (Baines et al., 1990; Lange and Nykamp, 1996). Furthermore, the lack of an octopamine effect on cGMP concentrations and the absence of the other cyclic nucleotides clearly suggests that mACs mediate the observed octopamine effects. Hasan et al., 2014 could show that mAC activation leads to exclusive cAMP increase. Our results strongly suggests that β octopamine receptor activation is necessary for honeybee thermogenesis, since these receptors are known to be positively coupled mACs (Balfanz et al., 2014). Our explanation again receives support by pharmacological thermography. Due to the lack of subtype-specific octopamine receptor antagonists, we made use of well-established adrenoceptor antagonists. Deuterostome adrenoceptor and arthropoda octopamine receptors are very closely related (Roeder, 2005; Spindler et al., 2013; Fuchs et al., 2014; Hochman, 2015; Roeder, 2020), which also applies to receptor subtypes as supported by phylogenetic analyses (Qi et al., 2017). The reserpine and mianserin effects described above can be mimicked by alprenolol (Figure 5G). This antagonist is active at both, β1 and β2 adrenoceptors (Åblad et al., 1973; Åblad et al., 1972). Therefore, it represents a putative antagonist of AmOARβ1 and AmOARβ2 and was already used in insects in other studies (Belzunces et al., 1996; Cossío-Bayúgar et al., 2012). Contrastly, carvedilol and metoprolol did not cause any effect. Carvedilol antagonizes preferably α1 and β1 adrenoceptors (Hansson and Himmelmann, 1998), whereas metoprolol antagonizes βone adrenoceptors in the human heart (Benfield et al., 1986). We assume that both substances antagonize the corresponding octopamine receptors. Several studies show that metoprolol is effective in species belonging to all major protostome phyla (Dzialowski et al., 2006; Spindler et al., 2013; Jungmann et al., 2017; Buchberger et al., 2018). However, an expansion of the pharmacological profiles of honeybee octopamine receptors (Grohmann et al., 2003; Balfanz et al., 2014; Blenau et al., 2020) is needed to confirm whether the compounds we used actually antagonize the desired receptor proteins. Combining the information stated above with our results that alprenolol causes hypothermia but not yohimbine, which does not antagonize honeybee β octopamine receptors (Balfanz et al., 2014; Kovac et al., 2009), further supports the hypothesis that at least one β octopamine receptor subtype is crucially involved in honeybee thermogenesis. Since AmOARβ2 is predominantly expressed in the flight muscles (when compared with AmOARβ1 and AmOARβ3/4), AmOARβ2 is the most promising candidate. This assumption is supported by studies in mammals showing the predominant expression of the βtwo adrenergic receptor in skeletal muscle tissue, which is a similar receptor subtype (Liggett et al., 1988; Kim et al., 1991).
Our PCR analysis further revealed the prevalent expression of AmOARα1. However, yohimbine does not cause hypothermia. This substance was shown to bind and antagonize αone octopamine receptors receptors in a wide range of insects (Bischof and Enan, 2004; Enan, 2005; Ohtani et al., 2006; Huang et al., 2010). Thus, we hypothesize that this receptor is not in the service of thermogenesis.
Tyramine is also capable to reverse the reserpine induced hypothermia. However, we could observe neither tyramine nor any tyramine receptor gene expression in the flight muscles. One might argue that the tyramine effect is mediated via tyramine receptors that are expressed in the MMTG. In that case, the potent tyramine receptor antagonist yohimbine (Ohta et al., 2003; Fussnecker et al., 2006; Reim et al., 2017) should have an effect on thermogenesis, but this is not the case. Based on our results and the fact that tyramine is able to activate octopamine receptors (Grohmann et al., 2003; Balfanz et al., 2014; Blenau et al., 2020), we classify this tyramine effect as artificial and physiologically not relevant.
The data of our study supports the hypothesis that octopaminergic signaling in the flight muscle is necessary for honeybee thermogenesis. Most likely, this monoamine acts directly at the indirect flight muscles via the activation of β octopamine receptors. We speculate, that their role is to boost glycolysis (see scheme Figure 7). Cold stress will induce an octopamine release directly at the flight muscles. The subsequent β octopamine receptor mediated generation of cAMP will activate proteinkinase A (PKA, Müller, 2000). That PKA is in service of thermogenesis is supported by our experiments in which bees are hypothermic as a result of PKA inhibition. PKA in turn might phosphorylates and activates phosphofructokinase 2 (PFK-2), which is the enzyme that produces fructose-2,6-bisphosphate (F2,6P2, Rider et al., 2004). F2,6P2 is an activity increasing modulator of phosphofructokinase 1 (PFK-1, Hue and Rider, 1987; Bartrons et al., 2018). The PFK-1 mediated phosphorylation of fructose-6-phosphate (F6P) to fructose-1,6-bisphosphate (F1,6P2) is a key step in glycolysis, at its end ATP is provided (Fothergill-Gilmore and Michels, 1993). Finally, heat is generated by the hydrolysis of ATP at the actomyosin complex (Zhang and Feng, 2016). Our pyruvate quantification results support this hypothesis. We can detect higher quantities of the glycolysis final product after octopamine stimulation. Another possibility is that PKA is involved in the activation of certain transcription factors. As a consequence, the expression of genes of important glycolysis enzymes may be enhanced. Here, we provide the AmGAPDH gene as one example whose gene product is essential in glycolysis (Burke et al., 1996). Its expression can be increased by both cold stress and octopamine injection. The alternative futile cycle (Newsholme et al., 1972), which is based on high fructose-1,6-bisphosphatase (FbPase) activity in certain bumblebee species, must be doubted, at least for honeybees. Honeybees and many other bumblebee species have comparable low FbPase activity (Newsholme and Crabtree, 1970; Staples et al., 2004) and FbPase-PFK cycling rates are not sufficient for heat production (Clark et al., 1973; Kammer and Heinrich, 1978; Newsholme and Crabtree, 1976). Our hypothetical cascade is supported by the results of other studies. F2,6P2 levels increase in locust flight muscles due to octopamine stimulation (Blau and Wegener, 1994; Blau et al., 1994) and by this controls the rate of carbohydrate oxidation in flight muscles (Wegener, 1996). In mammals, adrenaline stimulates increasing F2,6P2 levels and thus glycolysis (Narabayashi et al., 1985). This effect is achieved by β adrenoreceptor activation followed by stimulation of PKA (Rider et al., 2004). Chronic exercise causes stereotypical adaptations in several tissues of Drosophila melanogaster, which requires the activation of octopaminergic neurons (Sujkowski et al., 2017). In muscles, those effects are dependent on the activation of β octopamine receptors (Sujkowski et al., 2020). If cold stress becomes chronic, such as in cold climate at high altitude or during winter, there will probably be a similar pattern in honeybees. It is conceivable that the octopaminergic system in the flight muscles is permanently active to enable persistent heat production. If this system is compromised, it will endanger the survival of the colony due to the lost of individually performed heating, which enables foraging, breeding, and diverse defense mechanisms (Himmer, 1932; Weiss, 1962; Starks et al., 2000; Bujok et al., 2002; Stabentheiner et al., 2003; Tautz et al., 2003; Ken et al., 2005; Baracchi et al., 2010; Campbell et al., 2010; Wang et al., 2016). This may explain the enormous selective pressure on β octopamine receptor genes (Wallberg et al., 2017). Issues to be addressed are how the octopaminergic system responds to cold stress. But also heat stress, and in this context adaptations to warm climate in the course of climate change can become very important. With our important contribution to the understanding of thermogenesis in honeybees we provide a solid basis to analyze these issues.
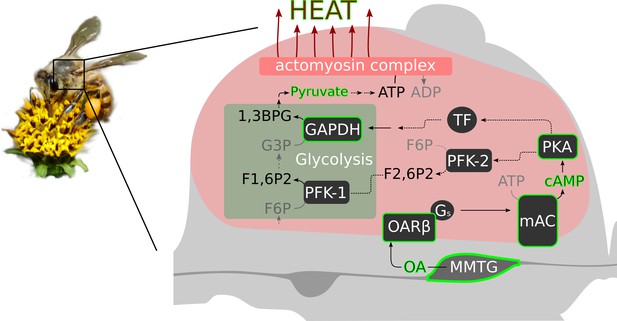
Octopamine and honeybee thermogenesis.
The scheme summarizes our findings, with the solid lines and green borders representing interpretations supported by our results and the dashed lines representing hypothetical pathways. Muscle innervating neurons in the MMTG release octopamine (OA) directly to the flight muscles. By this, AmOARβ two receptors are activated which in turn activate the membrane-bound adenylyl cyclase (mAC) via Gs proteins. The resulting increase in the intracellular cAMP concentration leads to the activation of Proteinkinase A (PKA) which phosphorylates and by this activates phosphofructokinase 2 (PFK-2). Consequently, this enzyme produces fructose-2,6-bisphosphate (F2,6P2) which increases the activity of phosphofructokinase 1 (PFK-1). An alternative pathway is the PKA mediated activation of transcription factors (TF) which might enhance expression of GAPDH which encodes glyceraldehyde 3-phosphate dehydrogenase (GAPDH). This enzyme converts glyceraldehyde 3-phosphate (G3P) into 1,3-bisphosphoglyceric acid (1,3BPG). All together, this increases the glycolysis rate so that a greater amount of pyruvate is available for ATP production. Finally, heat is generated in the actomyosin complex under ATP consumption.
Materials and methods
Animals
Honeybee workers (Apis mellifera carnica) were collected from colonies of the department next to the Biocenter at the University of Würzburg, Germany. We declared bees that returned to the hive with pollen loads on their hind legs as forager bees. As nurse bees, we defined bees, that were sitting on a brood comb and were actively heating (thorax temperature, TTHX32 °C). TTHX was monitored with a portable thermographic camera (FLIR E6, FLIR, Wilsonville, USA). Pollen forager were collected for the gene expression analysis from the same hives and were immediately flash-frozen in liquid nitrogen and stored at –80 °C. For the age-series analysis (monoamine quantification, gene expression analysis), a queen was caged on a brood comb for 3 days. Shortly before the bees started to emerge, we transferred the brood comb into an incubator (34 °C, RH = 65 %). The first group (0-day-old bees) consisted of newly hatched bees and were collected directly from the brood comb. The remaining newly hatched bees were color-marked and then inserted into a standard hive. Those bees were collected from the hive after 7, 14, 21, and 28 days, respectively. For the AmGAPDH expression analysis, 7-day-old age-marked bees were collected from a hive and distributed equally into two identical cages. For the cold stress experiment, one cage was placed in an incubator at 10 °C for 120 min, while the other served as a control (120 min, 34 °C). For the octopamine injection experiment, bees of the control group receive an injection of saline solution (270 mM sodium chloride, 3.2 mM potassium chloride, 1.2 mM calcium chloride, 10 mM magnesium chloride, 10 mM 3-(N-morpholino) propanesulfonic acid, pH = 7.4; Erber and Kloppenburg, 1995) into their flight muscles. The test group was injected with octopamine (0.01 M in saline). Subsequently, both groups were incubated for 120 min at 34 °C. All collected bees (expression analysis, monoamine quantification) were immediately flash-frozen in liquid nitrogen and subsequently stored at –80 °C.
Immunohistochemistry
Request a detailed protocolFor octopamine immunolabeling, we used a polyclonal rabbit anti-octopamine antibody (IS1033, ImmuSmol, Bordeaux, France) together with the STAINperfect immunostaining kit A (SP-A-1000, ImmuSmol, Bordeaux, France). We have analyzed ten individual MMTGs in three independent experiments for frontal sections and additionally three individual MMTGs for sagital sections. Four individual DVs and DLs, respectively, were analyzed in two independent experiments. Due to non optimal tissue permeability, we have slightly adopted the manufacturers protocol for whole mount preparations to perform analysis with vibratom sections. In brief, tissues (MMTG, flight muscles) were micro-dissected and subsequently fixed in fixation buffer for 3 hr at 4 °C while shaking. Afterwards, the fixed tissues were washed five times for 30 min with Wash Solution 1, embedded in 5% (w/v) agarose and were cut into 100-µm-thick sections. Then, the tissue sections were treated consecutively: 1 hr in Permeabilization Solution at RT followed by two times Wash Solution 1 for 3 min, 1 hr in Stabilization Solution followed by three times Wash Solution 1 for 3 min, and 1 hr in Saturation Solution at RT. Afterwards, the Saturation Solution was replaced by the primary antibody (1:500, in Antibody Diluent) and the tissue sections were incubated at 4 °C while shaking for at least for 72 hr. After five times washing cycles with Wash Solution 2 for 30 min at RT the secondary antibody (1:200 in Antibody Diluent, goat anti-rabbit Alexa Fluor 568; Molecular Probes, Eugene, USA) was applied for 24 hr (4 °C). After the final washing with Wash Solution two and Wash Solution 3 (both 3 times for 30 min at RT) the slices were mounted in 80% Glycerol (in Wash Solution 3) on microscope slides. Preparations were imaged by confocal laser scanning microscopy using a Leica TCS SP2 AOBS (Leica Microsystems AG, Wetzlar, Germany). HC PL APO objective lenses (10 x/0.4 NA imm; 20 x/ 0.7 NA imm and 63 x/1.20 NA imm) with additional digital zoom were used for image acquisition. ImageJ (1.53 c, Schindelin et al., 2012) was used to process images (maximum intensity projection, optimization for brightness and contrast) and Inkscape (1.1, Inkscape Developer Team, 2021) was used to arrange images into figures. MMTG nerve terminology is based on the nomenclature used by Markl, 1966.
Monoamine quantification
Request a detailed protocolThe DV and DL were dissected under liquid nitrogen. Afterwards, we thawed the remaining thoracic tissue in ice-cold ethanol to immediately dissect the MMTG. The separated tissues were kept at –80 °C until extraction. For high-performance liquid chromatography (HPLC) analysis of the monoamines we used a slightly modified protocol as described by Cook et al., 2017. For extraction, 120 µL (DV, DL) or 60 µL (MMTG) of extraction solution (10.0 pg/µL 3,4-dihydroxy-benzylamine (DHBA) in 0.2 M perchloric acid) was added in the first step. After a short centrifugation (21,130 g, 2 min, 0 °C) the tissues were disintegrated via sonication (10 min, 0 °C), followed by an incubation (20 min, 0 °C). After a final centrifugation (21,130 g, 14 min, 0 °C), the supernatant was analyzed via HPLC-ECD (Thermo Fisher Scientific, Waltham, USA) and the pellet was stored at –80 °C for protein quantification. A 3 µm reverse phase column (BDS-Hypersil-C18, 150 × 3 mm, pore size 130 Å, Thermo Fisher Scientific, Waltham, USA) and an ECD-3000RS configuration with two coulometric cells (6011RS ultra-analytical cell, Thermo Fisher Scientific, Waltham, USA) were connected to a biocompatible Dionex Ultimate 3,000 UHPLC focused (Thermo Fisher Scientific, Waltham, USA). The mobile phase contained 15% (v/v) methanol, 15% (v/v) acetonitrile, 85 mM sodium phosphate monobasic, 1.75 mM sodium dodecyl sulfate, 0.5 mM sodium citrate and ultrapure water. Phosphoric acid was used for accurate pH adjustment (pH 5.6 ± 0.01). We used a flow rate of 0.5 mL/min. Two detector channels were connected in series with working potentials of 425 mV (DHBA, dopamine, serotonin) and 800 mV (octopamine, tyramine), respectively. Quantification was performed via an external calibration. The raw data analysis was carried out with the program Chromeleon (7.2.10, Thermo Fisher Scientific, Waltham, USA).
Quantitative analysis of cyclic nucleotides
Request a detailed protocolIndividual flight muscle tissues were dissected under liquid nitrogen. Individual DV and DL were pooled and 800 µL homogenization buffer (40% (v/v) acetonitrile, 40% (v/v) methanol, 20% (v/v) H2O) was added and homogenized as described above. Samples were incubated at 95 °C for 10 min and then stored in the freezer (–80 °C) until further processing. After centrifugation (10 min, 21,130 g), the supernatant was transferred to mass spectroscopic analysis (HPLC-MS) as described by Beste et al., 2012. The residual pellet was used for the protein quantification.
Pyruvate quantification
Request a detailed protocolWorkerbees were killed by decapitating and then the intact DL muscle was carefully dissected and separated into mirror-identical parts. Subsequently, both parts were incubated with different solutions using bath application. One part was treated with saline solution whereas the other part was treated with 0.01 M octopamine (in saline). After flash freezing in liquid nitrogen pyruvate was quantified using the pyruvate assay kit (MAK071, Sigma Aldrich). The muscles were homogenized in 100 µL Pyruvate Assay Buffer and in a tissue mill at 35 Hz for 3 min. After centrifugation (10 min, 21,130 g), 25 µL of the supernatant were used per reaction. Each reaction setup (50 µL) additionally contained 23 µL Pyruvate Assay Buffer, 1 µL Pyruvate Probe Solution and 1 µL Pyruvate Enzyme Mix. After incubation at room temperature for 30 min the absorption at 570 nm was measured for each sample and each external calibrator (0, 2, 4, 6, 8, 10 nmol per reaction).
Protein quantification
Request a detailed protocolTo compensate possible differences in the accuracy of tissue dissection for the HPLC-ECD, HPLC-MS and the pyruvate quantification analysis, we additionally measured the protein content in the samples after Bradford (Fic et al., 2010) and normalized amine or cyclic nucleotide concentration to protein content. The pellet (see above) was resuspended in 120 µL (HPLC-ECD: DV, DL), 30 µL (HPLC-ECD: MMTG), or 500 µL (HPLC-MS: DV+ DL) 0.2 M NaOH. After an incubation (15 min, 0 °C), the insoluble material was sedimented (9391 g, 5 min). Finally, 2 µL (HPLC-ECD: DV, DL), 10 µL (HPLC-ECD: MMTG), or 2,5 µL (HPLC-MS: DV+ DL) of the supernatant were transferred into a final volume of 1 mL 1 x ROTINanoquant solution (Carl Roth, Karlsruhe, Germany). All samples and the external calibrator (1, 2, 3, 5, 10, 20 µg/mL Albumin Fraction V, Carl Roth, Karlsruhe, Germany) were analyzed with a plate reader (Infinite 200 Pro, Tecan, Männedorf, Switzerland).
Gene expression analysis
Request a detailed protocolIndividual flight muscle tissues were dissected under liquid nitrogen. For the MMTG, we have used RNAlater ICE (Thermo Fisher Scientific, Waltham, USA) to prevent RNA degradation during the dissections. The GenUP Total RNA Kit (biotechrabbit, Henningsdorf, Germany) was used to extract total RNA following the standard protocol provided by the manufacturer including an extra DNase I digestion step. After binding of the RNA to the Mini Filter RNA, we added a 50 µL DNase mix containing 30 U RNase-free DNase I (Lucigen Corporation, Middleton, USA) together with the appropriate buffer and incubated for 15 min at room temperature. For the polymerase chain reaction (PCR) experiment, we pooled total RNA from one individual of each age (7, 14, 21 and 28-day-old bees) per tissue (brain, MMTG, DV, DL). 400 ng total RNA of each tissue were used for cDNA synthesis using the Biozym cDNA Synthesis Kit (Biozym, Hessisch Oldendorf, Germany). The cDNAs were then analyzed in 20 µL PCR reactions (1 µL cDNA, 8.2 µL H2O, 10 µL 2 x qPCR S’Green BlueMix (Biozym, Hessisch Oldendorf, Germany)), 0.4 µL of each primer (0.2 µM) using the following protocol: 95 °C for 2 min and 35 cycles at 95 °C for 5 s and 30 °C for 30 s. Finally, 10 µL for each PCR reaction was analyzed on a 1.5% agarose gel. For the qPCR experiments, we used individual total RNA per tissue. Here, for each sample 70 ng (DV) and 30 ng (DL) RNA were used. All cDNA synthesis reactions were performed with the Biozym cDNA Synthesis Kit (Biozym, Hessisch Oldendorf, Germany). PCR triplicates of each cDNA (5 µL) were analyzed in a qPCR on a Rotor-Gene Q (Qiagen, Hilden, Germany) in a total reaction volume of 20 µL. Every reaction contains 4.2 µL H2O, 10 µL 2 x qPCR S’Green BlueMix (Biozym, Hessisch Oldendorf, Germany), 0.4 µL of each primer (0.2 µM) and 5 µL cDNA. Finally, octopamine receptor gene expression was determined relative to the reference genes AmGAPDH and AmRPL10 using the R package ’EasypcR’ (v1.1.3) which uses the algorithm published by Hellemans et al., 2007. For the AmGAPDH relative expression analysis AmRPL32 and AmRPL19 served as reference genes.
Pharmacological thermography
Request a detailed protocolFor the reserpine experiments, forager bees and nurse bees were collected as described above. The bees were kept and fed in equal proportions in two adjacent cages (34 °C, RH = 65 %) for 3 days. The reserpine group was fed with 500 µM reserpine solution (in 30% sucrose solution) ad libitum and the control group with 30% sucrose only. To enhance the solubility, the reserpine was pre-dissolved in acetone. For the experiments with receptor antagonists, the day before each measuring day, 20 bees were collected from the same hive and kept overnight in a cage at 34 °C (RH = 65 %). In the incubator, the bees were fed ad libitum with 30% sucrose solution. All injection solutions were freshly prepared every experimental day. All biogenic amines (Sigma-Aldrich), receptor antagonists (Sigma-Aldrich) or Rp-8-CPT-cAMPS (Biolog) were used in a concentration of 0.01 M in saline solution (see above). For solubility reasons, a 10:1 volume mixture of saline solution and dimethyl sulfoxide was used for carvedilol instead of pure buffer. Each bee was immobilized on ice until no more movement could be detected. The thorax was then punctured centrally to inject 1.0 µL testing solution using a 10.0 µL Hamilton syringe. Directly before the start of every measurement, the control group received an injection of the pure saline solution and the treatment group an injection of 0.01 M of the biogenic amine or the respective antagonist directly into their flight muscles. To enable optimal conditions for thermogenesis and thermographic recordings, we adapted the method of a tethered animal that walks upon a treadmill (Moore et al., 2014). This allows the bee to seemingly move freely, while at the same time the camera always monitors the same area of the bees thorax. This setup was located inside an incubator (18.5 °C, RH = 65 %) together with a thermographic camera (FLIR A65 camera, lens: 45°, f = 13 mm, FLIR, Wilsonville, USA). A thermal imaging video with 30 frames/min was recorded of each bee over 10 min. We converted the thermographic videos using the R package Thermimage (4.1.2, Tattersall, 2020) to subsequently read out the thoracic temperatures with ImageJ (1.53 c, Schindelin et al., 2012).
Statistical analysis
Request a detailed protocolAll statistical analyses were performed using R (4.0.4 including ’stats’, R Development Core Team, 2020) and the R packages ’rstatix’ (0.7.0, Kassambara, 2020b) and ’FSA’ (0.9.1, Ogle et al., 2021). We performed a Shapiro-Wilk test to check the data for normality distribution. Since most data subsets did not display a normal distribution, we analyzed the data using either the Mann-Whitney U test or the Kruskal-Wallis test followed by Dunns post hoc analysis if significant differences were observable. For the statistical analysis of the pharmacological thermography experiments, we calculated the mean value per min for TTHX and TA, respectively. Afterwards, the temperature (TTHX — TA) for the total time span of the experiment (five or 10 min) was subjected to nonparametric analysis of longitudinal data using a F1 LD F1 model of the R package ’nparLD’ (2.1 Noguchi et al., 2012). Visualization of the data was performed with the R packages ’ggplot2’ (3.3.3, Wickham, 2016), ‘ggpubr’ (0.4.0, Kassambara, 2020a), ’png’ (0.1–7, Urbanek, 2013), ’cowplot’ (1.1.1, Wilke, 2019), and ’magick’ (2.7.0, Wilke, 2019).
Data availability
All data generated or analyzed during this study are included in the manuscript and supporting file; Source Data files have been provided for Figures 1,3,4,5,6.
References
-
Pharmacological effects and serum levels of orally administered alprenolol in manEuropean Journal of Clinical Pharmacology 5:44–52.https://doi.org/10.1007/BF00560895
-
Serotoninergic innervation of the locust mandibular closer muscle modulates contractions through the elevation of cyclic adenosine monophosphateThe Journal of Comparative Neurology 294:623–632.https://doi.org/10.1002/cne.902940409
-
Molecular, pharmacological, and signaling properties of octopamine receptors from honeybee (Apis mellifera) brainJournal of Neurochemistry 129:284–296.https://doi.org/10.1111/jnc.12619
-
Defence reactions of Apis mellifera ligustica against attacks from the european hornet vespa crabroEthology, Ecology & Evolution 22:281–294.
-
Fructose 2,6-Bisphosphate in Cancer Cell MetabolismFrontiers in Oncology 8:331.https://doi.org/10.3389/fonc.2018.00331
-
Cloning, expression and functional analysis of an octopamine receptor from Periplaneta americanaInsect Biochemistry and Molecular Biology 34:511–521.https://doi.org/10.1016/j.ibmb.2004.02.003
-
The effect of octopamine on the glycolytic activator fructose 2,6-bisphosphate in perfused locust flight muscleInsect Biochemistry and Molecular Biology 24:677–683.https://doi.org/10.1016/0965-1748(94)90055-8
-
Metabolic integration in locust flight: the effect of octopamine on fructose 2,6-bisphosphate content of flight muscle in vivoJournal of Comparative Physiology B 164:11–15.https://doi.org/10.1007/BF00714565
-
AmOctα2R: functional characterization of a honeybee octopamine receptor inhibiting adenylyl cyclase activityInternational Journal of Molecular Sciences 21:.https://doi.org/10.3390/ijms21249334
-
BookThe unpaired median neurons of insectsIn: Bräunig P, editors. Advances in Insect Physiology. Academic Press. pp. 185–266.
-
Effects of metoprolol on aquatic invertebrates in artificial indoor streamsJournal of Environmental Science and Health. Part A, Toxic/Hazardous Substances & Environmental Engineering 53:728–739.https://doi.org/10.1080/10934529.2018.1444964
-
Hot spots in the bee hiveDie Naturwissenschaften 89:299–301.https://doi.org/10.1007/s00114-002-0338-7
-
Biogenic amine levels change in the brains of stressed honeybeesArchives of Insect Biochemistry and Physiology 68:241–250.https://doi.org/10.1002/arch.20259
-
Octopamine and tyramine modulate the thermoregulatory fanning response in honey bees (Apis mellifera)The Journal of Experimental Biology 220:1925–1930.https://doi.org/10.1242/jeb.149203
-
Probing the cyclic nucleotide binding sites of cAMP-dependent protein kinases I and II with analogs of adenosine 3’,5’-cyclic phosphorothioatesThe Journal of Biological Chemistry 265:10484–10491.https://doi.org/10.1016/S0021-9258(18)86973-3
-
Distribution and activation of different types of octopaminergic DUM neurons in the locustThe Journal of Comparative Neurology 403:119–134.https://doi.org/10.1002/(sici)1096-9861(19990105)403:1<119::aid-cne9>3.0.co;2-f
-
Physiological and reproductive effects of beta adrenergic receptor antagonists in Daphnia magnaArchives of Environmental Contamination and Toxicology 50:503–510.https://doi.org/10.1007/s00244-005-0121-9
-
Molecular and pharmacological analysis of an octopamine receptor from American cockroach and fruit fly in response to plant essential oilsArchives of Insect Biochemistry and Physiology 59:161–171.https://doi.org/10.1002/arch.20076
-
The modulatory effects of serotonin and octopamine in the visual system of the honey bee (Apis mellifera L.)Journal of Comparative Physiology A 176:.https://doi.org/10.1007/BF00197757
-
Ein neuer Bewegungstyp im Schwnzeltanz der BienenDie Naturwissenschaften 48:140–141.https://doi.org/10.1007/BF00631947
-
Body temperature and flight performance of honey bees in a servo-mechanically controlled wind tunnelJournal of Comparative Physiology? A 109:265–277.https://doi.org/10.1007/BF00663608
-
Neural control of fibrillar muscles in bees during shivering and flightJournal of Experimental Biology 159:419–431.https://doi.org/10.1242/jeb.159.1.419
-
Evolution of glycolysisProgress in Biophysics and Molecular Biology 59:105–235.https://doi.org/10.1016/0079-6107(93)90001-z
-
Octopamine and tyramine influence the behavioral profile of locomotor activity in the honey bee (Apis mellifera)Journal of Insect Physiology 52:1083–1092.https://doi.org/10.1016/j.jinsphys.2006.07.008
-
Novel (Rp)-cAMPS Analogs as Tools for Inhibition of cAMP-kinase in Cell CultureJournal of Biological Chemistry 270:20599–20607.https://doi.org/10.1074/jbc.270.35.20599
-
Carvedilol in the treatment of hypertension - a review of the clinical data aseScandinavian Cardiovascular Journal 32:67–80.https://doi.org/10.1080/140174398428072
-
Roles of individual honeybee workers and drones in colonial thermogenesisThe Journal of Experimental Biology 129:53–61.https://doi.org/10.1242/jeb.129.1.53
-
Soluble adenylyl cyclase accounts for high basal cCMP and cUMP concentrations in HEK293 and B103 cellsBiochemical and Biophysical Research Communications 448:236–240.https://doi.org/10.1016/j.bbrc.2014.04.099
-
Die Temperaturregulierung im BienenvolkZeitschrift Fur Vergleichende Physiologie 4:465–487.https://doi.org/10.1007/BF00340746
-
Die temperaturverhältnisse bei den sozialen hymenopterenBiological Reviews 7:224–253.https://doi.org/10.1111/j.1469-185X.1962.tb01042.x
-
Metabolic recruitment of spinal locomotion: intracellular neuromodulation by trace amines and their receptorsNeural Regeneration Research 10:1940–1942.https://doi.org/10.4103/1673-5374.169625
-
Pharmacological characterization of a Bombyx mori alpha-adrenergic-like octopamine receptor stably expressed in a mammalian cell lineArchives of Insect Biochemistry and Physiology 73:74–86.https://doi.org/10.1002/arch.20341
-
Role of fructose 2,6-bisphosphate in the control of glycolysis in mammalian tissuesThe Biochemical Journal 245:313–324.https://doi.org/10.1042/bj2450313
-
Health effects of metoprolol in epibenthic and endobenthic invertebrates-A basis to validate future in vitro biotests for effect-based biomonitoringJournal of Environmental Science and Health. Part A, Toxic/Hazardous Substances & Environmental Engineering 52:189–200.https://doi.org/10.1080/10934529.2016.1246930
-
BookInsect flight metabolismIn: Treherne J, Berridge M, Wigglesworth V, editors. Advances in Insect Physiology. Academic Press. pp. 133–228.
-
Heat-balling wasps by honeybeesDie Naturwissenschaften 92:492–495.https://doi.org/10.1007/s00114-005-0026-5
-
Characterization of beta 1- and beta 2-adrenoceptors in rat skeletal musclesBiochemical Pharmacology 42:1783–1789.https://doi.org/10.1016/0006-2952(91)90516-8
-
Colonial thermoregulation in honey bees (Apis mellifera)Journal of Comparative Physiology ? B 148:65–76.https://doi.org/10.1007/BF00688889
-
Signal transduction pathways regulating the contraction of an insect visceral muscleArchives of Insect Biochemistry and Physiology 33:183–196.https://doi.org/10.1002/(SICI)1520-6327(1996)33:3/4<183::AID-ARCH2>3.0.CO;2-U
-
Characterization of beta-adrenergic receptors of human skeletal muscle obtained by needle biopsyThe American Journal of Physiology 254:795–798.https://doi.org/10.1152/ajpendo.1988.254.6.E795
-
Formica Polyctena483–496, Peripheres Nervensystem und Muskulatur im Thorax von der Arbeiterin von Apis mellifera L, Formica Polyctena, Zool Jb Anat.
-
FicTrac: A visual method for tracking spherical motion and generating fictive animal pathsJournal of Neuroscience Methods 225:106–119.https://doi.org/10.1016/j.jneumeth.2014.01.010
-
Regulation of phosphofructokinase in perfused rat heart. Requirement for fructose 2,6-bisphosphate and a covalent modificationThe Journal of Biological Chemistry 260:9750–9758.
-
Substrate cycles in metabolic regulation and in heat generationBiochemical Society Symposium pp. 61–109.
-
nparLD: An R software package for the nonparametric analysis of longitudinal data in factorial experimentsJournal of Statistical Software 50:1–23.
-
B96Bom encodes a Bombyx mori tyramine receptor negatively coupled to adenylate cyclaseInsect Molecular Biology 12:217–223.https://doi.org/10.1046/j.1365-2583.2003.00404.x
-
ThesisThe neural organization of the ocellar system andassociated pathways in the central nervous system634 of the workerhoneybee. PhD thesisUniversity of London.
-
Pharmacology of Rauwolfia alkaloids, including reserpineAnnals of the New York Academy of Sciences 59:8–21.https://doi.org/10.1111/j.1749-6632.1954.tb45914.x
-
A new Drosophila octopamine receptor responds to serotoninInsect Biochemistry and Molecular Biology 90:61–70.https://doi.org/10.1016/j.ibmb.2017.09.010
-
SoftwareR FoundatioR Foundation for Statistical Computing, Vienna, Austria.
-
AmTAR2: Functional characterization of a honeybee tyramine receptor stimulating adenylyl cyclase activityInsect Biochemistry and Molecular Biology 80:91–100.https://doi.org/10.1016/j.ibmb.2016.12.004
-
Muscle biochemistry and the ontogeny of flight capacity during behavioral development in the honey bee, Apis melliferaThe Journal of Experimental Biology 208:4193–4198.https://doi.org/10.1242/jeb.01862
-
Tyramine and octopamine: ruling behavior and metabolismAnnual Review of Entomology 50:447–477.https://doi.org/10.1146/annurev.ento.50.071803.130404
-
The control of metabolic traits by octopamine and tyramine in invertebratesThe Journal of Experimental Biology 223:.https://doi.org/10.1242/jeb.194282
-
Fiji: an open-source platform for biological-image analysisNature Methods 9:676–682.https://doi.org/10.1038/nmeth.2019
-
Mesothoracic ventral unpaired median (mesVUM) neurons in the blowfly Calliphora erythrocephalaThe Journal of Comparative Neurology 467:435–453.https://doi.org/10.1002/cne.10930
-
BookThe Wisdom of the HiveCambridge Mass, London: Harvard University Press.https://doi.org/10.4159/9780674043404
-
Endothermic heat production in honeybee winter clustersThe Journal of Experimental Biology 206:353–358.https://doi.org/10.1242/jeb.00082
-
`Futile cycle’ enzymes in the flight muscles of North American bumblebeesJournal of Experimental Biology 207:749–754.https://doi.org/10.1242/jeb.00825
-
Softwaregtatters/thermimage, version v4.1.2Thermimage.
-
Flying insects: model systems in exercise physiologyExperientia 52:404–412.https://doi.org/10.1007/BF01919307
-
Über die Lebensfähigkeit von offener und gedeckelter Brut ausserhalb des BienenvolkesZ. Bienenforsch 6:104–114.
-
Sound production during the waggle dance of the honey beeAnimal Behaviour 10:79–95.https://doi.org/10.1016/0003-3472(62)90135-5
-
Elegant Graphics for Data Analysisggplot2, Elegant Graphics for Data Analysis, Cham, Springer-Verlag New York, 10.1007/978-3-319-24277-4.
-
Thermodynamic aspects of ATP hydrolysis of actomyosin complexBiophysics Reports 2:87–94.https://doi.org/10.1007/s41048-016-0032-5
-
PKM2, function and expression and regulationCell & Bioscience 9:52.https://doi.org/10.1186/s13578-019-0317-8
Article and author information
Author details
Funding
Deutsche Forschungsgemeinschaft (TH2264/2-1)
- Markus Thamm
The funders had no role in study design, data collection and interpretation, or the decision to submit the work for publication.
Acknowledgements
We thank Dirk Ahrens for beekeeping; Annette Garbe, Anna Senker, Feriba Ghanbari, Karin Möller, Lena Wolf, Linnéa Jürgensen, Saskia Delac and Valentina Vey for technical assistance during the experiments; Flavio Roces, Johannes Spaethe, Marcus Gutmann, Petra Högger, Ricarda Scheiner and Robin Grob for supplying technical equipment and Michael G.K. Brunk for his help with ImageJ. Usage of the Leica SP2 confocal Laser Scanning Microscope was made possible by the the Deutsche Forschungsgemeinschaft (DFG, German Research Foundation) - HBFG-133–612. A special thanks goes to Christian Wegener, Jens Schlossmann, Anna Stöckl and Basil el Jundi for fruitful discussions and/or reviewing earlier versions of the manuscript. This publication was supported by the Open Access Publication Fund of the University of Wuerzburg.
Copyright
© 2022, Kaya-Zeeb et al.
This article is distributed under the terms of the Creative Commons Attribution License, which permits unrestricted use and redistribution provided that the original author and source are credited.
Metrics
-
- 2,111
- views
-
- 316
- downloads
-
- 16
- citations
Views, downloads and citations are aggregated across all versions of this paper published by eLife.
Citations by DOI
-
- 16
- citations for umbrella DOI https://doi.org/10.7554/eLife.74334