Coil-to-α-helix transition at the Nup358-BicD2 interface activates BicD2 for dynein recruitment
Abstract
Nup358, a protein of the nuclear pore complex, facilitates a nuclear positioning pathway that is essential for many biological processes, including neuromuscular and brain development. Nup358 interacts with the dynein adaptor Bicaudal D2 (BicD2), which in turn recruits the dynein machinery to position the nucleus. However, the molecular mechanisms of the Nup358/BicD2 interaction and the activation of transport remain poorly understood. Here for the first time, we show that a minimal Nup358 domain activates dynein/dynactin/BicD2 for processive motility on microtubules. Using nuclear magnetic resonance titration and chemical exchange saturation transfer, mutagenesis, and circular dichroism spectroscopy, a Nup358 α-helix encompassing residues 2162–2184 was identified, which transitioned from a random coil to an α-helical conformation upon BicD2 binding and formed the core of the Nup358-BicD2 interface. Mutations in this region of Nup358 decreased the Nup358/BicD2 interaction, resulting in decreased dynein recruitment and impaired motility. BicD2 thus recognizes Nup358 through a ‘cargo recognition α-helix,’ a structural feature that may stabilize BicD2 in its activated state and promote processive dynein motility.
Editor's evaluation
The paper provides evidence that a peptide from the nuclear pore protein Nup358 (RanBP2) forms a helix upon binding the dynein/dynactin adaptor BICD2 and is able to activate BICD2 in the same way as the small G-protein Rab6. Helix formation to activate BicD2 is a novel concept and will open up the search space for other potential activators that may be unstructured in their apo states.
https://doi.org/10.7554/eLife.74714.sa0Introduction
Cytoplasmic dynein is the predominant motor responsible for minus-end-directed traffic on microtubules (Reck-Peterson et al., 2018), which facilitates a vast number of transport events that are critical for chromosome segregation, signal transmission at synapses, and brain and muscle development (Fu and Holzbaur, 2014; Hendricks et al., 2010; Wilson and Holzbaur, 2012; Zhu et al., 2017; Splinter et al., 2010; Bolhy et al., 2011; Morris and Hollenbeck, 1993; Soppina et al., 2009; Encalada et al., 2011; Dharan and Campbell, 2018; Hu et al., 2013; Baffet et al., 2015; Gonçalves et al., 2020; Zhang et al., 2007; Zhang et al., 2009). Integral to the transport machinery are dynein adaptors, such as Bicaudal D2 (BicD2), whose N-terminal region (BicD2CC1) recruits and activates dynein-dynactin (DD) for processive motility (Splinter et al., 2012; Schlager et al., 2014b; Schlager et al., 2014a; Hoogenraad et al., 2003; Hoogenraad et al., 2001; McKenney et al., 2014; Urnavicius et al., 2018; Urnavicius et al., 2015; Sladewski et al., 2018; McClintock et al., 2018; Liu et al., 2013). Also integral to the dynein transport machinery are cargoes, which bind to the C-terminal domain (CTD) of BicD2 (Matanis et al., 2002). Cargoes are required to activate BicD2 for dynein binding, which is a key regulatory step for dynein-dependent transport (Splinter et al., 2012; Schlager et al., 2014b; Schlager et al., 2014a; Hoogenraad et al., 2003; Hoogenraad et al., 2001; McKenney et al., 2014; Urnavicius et al., 2018; Urnavicius et al., 2015; Sladewski et al., 2018; McClintock et al., 2018; Liu et al., 2013). In the absence of cargo adaptor/dynein adaptor complexes such as Nup358/BicD2, dynein and dynactin are autoinhibited and only show diffusive motion on microtubules. Furthermore, in the absence of cargoes, BicD2 assumes a looped, autoinhibited conformation, in which its N-terminal dynein/dynactin-binding site binds to the CTD and remains inaccessible. The CTD is required for autoinhibition as a truncated BicD2 without the CTD activates dynein/dynactin for processive motility. Binding of cargo to the CTD releases autoinhibition, likely resulting in an extended conformation that recruits dynein and dynactin (Splinter et al., 2012; Schlager et al., 2014b; Schlager et al., 2014a; Hoogenraad et al., 2003; Hoogenraad et al., 2001; McKenney et al., 2014; Urnavicius et al., 2018; Urnavicius et al., 2015; Sladewski et al., 2018; McClintock et al., 2018; Liu et al., 2013). The crystal structures of the C-terminal cargo recognition domains of three BicD2 homologs have been determined (Liu et al., 2013; Terawaki et al., 2015; Noell et al., 2019). However, to date, there are no detailed structural studies of BicD2/cargo complexes and the structural mechanisms of BicD2-mediated cargo recognition and dynein activation remain poorly understood.
The binding partners for human BicD2 include Nup358 (Splinter et al., 2010), Rab6GTP (Matanis et al., 2002), and nesprin-2G (Gonçalves et al., 2020). Nup358, also known as RanBP2, is a 358 kDa nuclear pore complex protein with multiple functions (Wu et al., 1995). During G2 phase, Nup358 engages in a pathway for positioning of the nucleus relative to the centrosome along microtubules by binding to BicD2, which in turn recruits dynein and dynactin (Figure 1; Splinter et al., 2010). This pathway is essential for apical nuclear migration during differentiation of radial glial progenitor cells, which give rise to the majority of neurons and glia cells of the neocortex (Hu et al., 2013; Baffet et al., 2015). A second nuclear positioning pathway is facilitated by BicD2/dynein and nesprin-2G (Gonçalves et al., 2020), a component of linker of nucleoskeleton and cytoskeleton (LINC) complexes (Fridolfsson et al., 2010), which is important for migration of post-mitotic neurons during brain development (Gonçalves et al., 2020). Apart from its roles in nuclear positioning, BicD2 is also involved in the transport of Golgi-derived and secretory vesicles. In this process, BicD2 and dynein are recruited by the vesicle-associated small GTPase Rab6 (Matanis et al., 2002; Grigoriev et al., 2007). Thus, BicD2 plays important roles in faithful chromosome segregation, neurotransmission at synapses, as well as brain and muscle development (Splinter et al., 2010; Hu et al., 2013; Baffet et al., 2015; Gonçalves et al., 2020; Zhang et al., 2009; Matanis et al., 2002). Mutations in BicD2 cause neuromuscular diseases, including a subset of spinal muscular atrophy cases (Neveling et al., 2013; Peeters et al., 2013; Martinez-Carrera and Wirth, 2015; Tsai et al., 2020; Synofzik et al., 2014), the most common genetic cause of infant death (Meijboom et al., 2017).
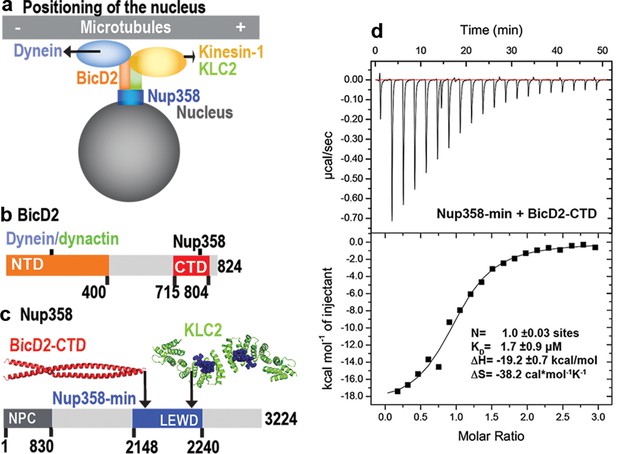
A minimal Nup358 domain interacts with BicD2 with micromolar affinity.
(a) Nup358 interacts with both BicD2/dynein/dynactin and kinesin-1 (via kinesin-1 light chain 2, KLC2) to mediate bidirectional nuclear positioning in G2 phase of the cell cycle (Splinter et al., 2010; Cai et al., 2001; Cui et al., 2019). This pathway is essential for a fundamental process in brain development that is required for radial glial progenitor cells to differentiate to the majority of neurons and glia cells of the neocortex (Hu et al., 2013). (b, c) Schematic representation of the expression constructs BicD2-CTD (b, red) and Nup358-min (c, blue), in the context of the full-length proteins (gray). (c) KLC2 is recruited to Nup358-min via a W-acidic motif with the sequence LEWD (Cui et al., 2019). The X-ray structures of the TPR domain of the KLC2 (green, referred to as KLC2 hereafter), fused to a LEWD motif (purple) (Pernigo et al., 2013), and the X-ray structure of the BicD2-CTD (red) (Noell et al., 2019) are shown in cartoon representation in (c). The α-helical N-terminal domain of Nup358 (dark gray) promotes anchorage to the nuclear pore complex (NPC). (d) A representative isothermal titration calorimetry (ITC) thermogram of Nup358-min and BicD2-CTD is shown, from which the affinity (dissociation constant KD) was determined to be 1.7 ± 0.9 μM. N: number of sites; ∆S: the change in entropy; ∆H: the change in enthalpy. The experiment was repeated three times. The error of KD was calculated as the standard deviation from three experiments. In Figure 1—figure supplement 1, the affinity of Nup358-min-GST (i.e., with the GST-tag intact) and BicD2-CTD was determined by ITC to be 1.6 ± 1.0 μM. See also Figure 1—source data 1–4.
-
Figure 1—source data 1
ITC thermogram of BicD2-CTD and Nup358-min.
- https://cdn.elifesciences.org/articles/74714/elife-74714-fig1-data1-v4.zip
-
Figure 1—source data 2
ITC thermogram of BicD2-CTD and Nup358-min-GST (i.e., with the GST-tag intact).
- https://cdn.elifesciences.org/articles/74714/elife-74714-fig1-data2-v4.zip
-
Figure 1—source data 3
ITC thermogram of Nup358-min into buffer.
- https://cdn.elifesciences.org/articles/74714/elife-74714-fig1-data3-v4.zip
-
Figure 1—source data 4
ITC thermogram of Nup358-min-GST into buffer.
- https://cdn.elifesciences.org/articles/74714/elife-74714-fig1-data4-v4.zip
Within the Nup358 sequence, there are many intrinsically disordered regions (IDRs), which are also commonly found in proteins involved in dynein-dependent transport (Zhu et al., 2017; Celestino et al., 2019; Lee et al., 2020; Henen et al., 2021; Lee et al., 2018). Although IDRs and intrinsically disordered proteins (IDPs) make up ~30% of eukaryotic proteins and have important physiological functions (Ward et al., 2004), they remain the most poorly characterized class of proteins in regards to their structure, dynamics, and interactions. IDRs play important roles in dynein biology. For example, an IDR in the dynein light intermediate chain 1 (LIC1) undergoes a coil-to-α-helix transition when it interacts with the N-terminal domain of BicD2, which is an important step in the activation of dynein for processive motility (Celestino et al., 2019; Lee et al., 2020; Lee et al., 2018). A second, larger interface is formed between the N-terminal coiled coil of BicD2, the dynein tail, and dynactin, which promotes activation of dynein for processive motility (Splinter et al., 2012; Schlager et al., 2014b; McKenney et al., 2014; Urnavicius et al., 2015). Currently, the mechanism of dynein activation by full length BicD2/cargo complexes is not well understood since much of the available information pertaining to dynein activation was derived from studies with isolated BicD2 fragments.
In addition to BicD2, Nup358 also recruits the opposite polarity motor kinesin-1 via the subunit kinesin-1 light chain 2 (KLC2), which binds to a W-acidic motif with the sequence LEWD in Nup358 (Cai et al., 2001; Cui et al., 2019; Dodding et al., 2011). While dynein is the predominant motor in G2 phase, kinesin-1 is also actively involved in nuclear positioning in G2 phase, modulating overall motility (Splinter et al., 2010). Such bidirectional transport is also displayed by mitochondria, endosomes, viruses, phagosomes, secretory vesicles, and many vesicles in neuronal axons and growth cones (Fu and Holzbaur, 2014; Hendricks et al., 2010; Wilson and Holzbaur, 2012; Zhu et al., 2017; Splinter et al., 2010; Bolhy et al., 2011; Morris and Hollenbeck, 1993; Soppina et al., 2009; Encalada et al., 2011; Dharan and Campbell, 2018; Gonçalves et al., 2020). Opposite polarity motors such as BicD2/dynein and kinesin-1 often bind in close spatial proximity to adapter-binding proteins such as Nup358, but it is unknown how their overall motility is regulated, likely because their interactions remain poorly characterized by structural and biophysical methods.
Here, we have determined the structural properties of the interface of a minimal Nup358/BicD2 complex by a combination of nuclear magnetic resonance (NMR) spectroscopy, mutagenesis, circular dichroism (CD) spectroscopy, and small-angle X-ray scattering. These results establish a structural basis for cargo recognition by BicD2 and suggest that Nup358 interacts with BicD2 through a ‘cargo recognition’ α-helix. Direct activation of BicD2 by Nup358 has not been shown before. Here, we use single-molecule-binding and processivity assays to show that a minimal dimerized Nup358 construct is sufficient to activate full-length dynein/dynactin/BicD2 complexes for processive motility. Mutations of the cargo recognition α-helix of Nup358 decreased the Nup358/BicD2 interaction and resulted in decreased dynein recruitment and impaired motility, shedding light on the important role of the cargo recognition α-helix for the activation of BicD2 and dynein motility. Intriguingly, our results also show that the binding site of BicD2 in Nup358 is spatially close to but does not overlap with the LEWD motif that acts as a kinesin-1-binding site, suggesting that the kinesin and dynein machineries may interact simultaneously via Nup358. Our results thus provide mechanistic insights into the regulation of bidirectional transport by adapter-binding proteins.
Results
ITC establishes a minimal complex for Nup358/BicD2 interaction
Previously, we have determined an X-ray structure of the CTD of human BicD2 (BicD2-CTD, residues 715–804), which contains the binding sites for cargoes, including human Nup358 (Figure 1b; Noell et al., 2019). A complex was reconstituted with BicD2-CTD and a minimal fragment of human Nup358 containing residues 2148–2240, which is called Nup358-min (Noell et al., 2019; Cui et al., 2019; Figure 1c). Here, the affinity of the BicD2-CTD towards Nup358-min was determined by isothermal titration calorimetry (ITC) (Figure 1d, Figure 1—figure supplement 1). The ITC thermogram fits well to a one-site-binding model (with a single-equilibrium dissociation constant KD). The number of sites was determined to be N = 1.0, which is consistent with a molar ratio of [Nup358]/[BicD2] of 1. This molar ratio is in agreement with our previously published molar masses obtained from size-exclusion chromatography coupled to multi-angle light scattering (SEC-MALS), which showed that Nup358 and BicD2 form a 2:2 complex (Noell et al., 2018). The one-site-binding model and the 2:2 stoichiometry are in line with Nup358-min binding as a dimer to a single binding site on a BicD2 coiled-coil dimer, although we cannot exclude the possibility that two Nup358 monomers bind to two binding sites on BicD2, where both sites have the same dissociation constant KD. We also attempted to fit models assuming multiple binding sites and KDs, but those are not supported by the ITC thermogram. The equilibrium dissociation constant KD was determined to be 1.7 ± 0.9 μM, in a similar range as observed for other BicD2/cargo complexes as well as to the previously published affinity of 0.4 μM, obtained for BicD2-CTD towards a larger fragment of Nup358 (residues 2006–2443, with the GST-tag intact) (Noell et al., 2018). An ITC titration of Nup358-min-GST (i.e., with the GST-tag intact, whereas the GST was cleaved off in the first experiment) with BicD2-CTD yielded a very similar affinity of 1.6 ± 1.0 μM (Figure 1—figure supplement 1), demonstrating that the GST-tag does not affect the binding affinity. These ITC results confirm the mapped boundaries of the minimal binding site. The ITC analysis finally revealed that the Nup358-BicD2 interaction is driven by a favorable enthalpy change (ΔH = –19.2 ± 0.7 kcal/mol), which overcomes the unfavorable entropy change (ΔS = –38.2 ± 5.8 cal/mol/K).
The DD-BicD2-Nup358min complex moves processively on microtubules
Single-molecule reconstitutions were used to determine if the adaptor-binding protein Nup358min can bind and relieve BicD2 autoinhibition, which in turn allows dynein and dynactin (DD) to be recruited and activated for processive motion. Nup358min (N) and full-length BicD2 (B) were labeled with two different color quantum dots (Qdots), and tissue-purified DD was unlabeled (Figure 2a). When dynein, dynactin, BicD2, and Nup358min were all present (DDBNmin), 23% dual-color complexes were observed (Figure 2—figure supplement 1a and c). To increase complex formation, Nup358min was artificially dimerized with a leucine zipper (Nup358min-zip), which increased the number of dual-colored complexes to 35% (Figure 2—figure supplement 1a and b). The rationale for this strategy was based on our previous observation that dimerization of the Drosophila adaptor-binding protein Egalitarian enhanced its affinity for BicD and bypassed the requirement for mRNA cargo for BicD activation (Sladewski et al., 2018). All further single-molecule reconstitutions thus used the dimerized version of Nup358-min (Nup358min-zip) because of its enhanced affinity for BicD2.
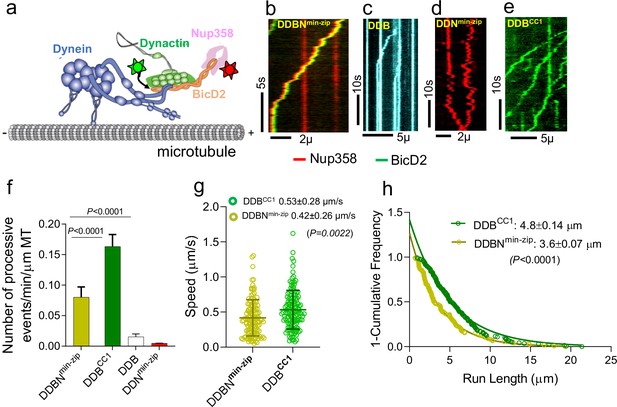
Nup358min-zip is capable of forming a dynein-dynactin-BicD2-Nup358min-zip complex (DDBNmin-zip) that is activated for processive motility.
(a) Schematic of a DDBNmin-zip complex bound to a microtubule. BicD2 and Nup358 are shown labeled with two different color quantum dots (Qdot; stars). (b) Representative kymograph of the DDBNmin-zip complex moving processively on microtubules. Nup358min-zip was labeled with a 655 nm Qdot and BicD2 with a 525 nm Qdot. (c) Dynein-dynactin-BicD2 (DDB) complexes bind to microtubules but show very little processive motion. (d) Dynein-dynactin-Nup358min-zip complexes (DDNmin-zip) showed diffusive movement on microtubules. Nup358min-zip was labeled with a 655 nm Qdot. (e) As a control, processive motion of dynein-dynactin in the presence of the N-terminal domain of BicD2 (DDBCC1), with BicD2CC1 labeled with a 525 nm Qdot. (f) Bar graph showing the number of processive events of DDBNmin-zip per min per micrometer microtubule (MT) length. As controls, number of processive events are shown for active DDBCC1, DDB, and DDNmin-zip (g, h) Speed and run length of DDBNmin-zip (yellow) were compared with the constitutively active complex DDBCC1 (green). Speeds for the two complexes are significantly different (p<0.0022, unpaired t-test with Kolmogorov–Smirnov test), as are the run lengths (p<0.0001, Kolmogorov–Smirnov test). See also Figure 2—figure supplement 1. For each panel, data were obtained from three independent experiments and two protein preparations.
-
Figure 2—source data 1
The number of processive events of DDBNmin-zip compared with DDBCC1, DDB, and DDNmin-zip.
- https://cdn.elifesciences.org/articles/74714/elife-74714-fig2-data1-v4.xlsx
-
Figure 2—source data 2
Speed of DDBNmin-zip compared with the constitutively active complex DDBCC1.
- https://cdn.elifesciences.org/articles/74714/elife-74714-fig2-data2-v4.xlsx
-
Figure 2—source data 3
Run length of DDBNmin-zip compared with the constitutively active complex DDBCC1.
- https://cdn.elifesciences.org/articles/74714/elife-74714-fig2-data3-v4.xlsx
The dynein-dynactin-BicD2-Nup358min-zip (DDBNmin-zip) complex exhibited robust processive motion on surface-immobilized microtubules (MTs), implying that Nup358min-zip relieves BicD2 autoinhibition to allow dynein activation (Figure 2b, Figure 2—video 1). As controls, DDB was shown to support a few processive events (Figure 2c, Figure 2—video 2), and DDNmin-zip exhibited only diffusive motion (Figure 2d, Figure 2—video 13). Nup358min-zip alone does not bind to microtubules (Figure 2—figure supplement 1d), and thus Nup358min-zip shows diffusive MT binding by virtue of an interaction with DD. The number of processively moving DDBN complexes on MTs is ~5.3 times higher than that of DDB complexes (Figure 2f). The speed and run length of all dual-color DDBNmin-zip complexes were analyzed, with the run length obtained from cumulative distribution analysis with a one-phase exponential decay fit, and speed determined from the mean ± standard deviation (SD). The speed and run length of DDBNmin-zip were quantified as 0.42 ± 0.26 µm/s (n = 123) and 3.6 ± 0.07 µm (n = 123), respectively (Figure 2g and h). These motile properties of DDBNmin-zip were compared with that of the DDBCC1 complex (BCC1, the N-terminal coiled-coil 1 domain of BicD2), a well-established fully active complex (McKenney et al., 2014; Sladewski et al., 2018; Figure 2e and f, Figure 2—video 3). The speed and run length of the DDBCC1 complex were 0.53 ± 0.28 µm/s (n = 137) and 4.8 ± 0.14 µm (n = 137), respectively, which are significantly faster (p=0.0022) and longer (p<0.0001 than that of DDBNmin-zip; Figure 2g and h). The number of processive events of DDBNmin-zip on MTs is approximately half that of the active DDBCC1 complex (Figure 2f). Importantly, the directed motion of DDBNmin-zip is very different from the autoinhibited DD complex, which shows only diffusive movement on MTs (Sladewski et al., 2018). The enhanced number of processive events only in the presence of both BicD2 and Nup358min-zip suggests that Nup358min-zip functionally relieves the autoinhibition of BicD2 so that DD can bind and move processively on MTs.
NMR titration mapped the BicD2-binding site to the N-terminal half of Nup358-min
Because our single-molecule processivity assays confirmed that the Nup358-min domain forms a DDBN complex that is activated for processive motility, we characterized the BicD2-binding site on Nup358-min, employing solution NMR, which can provide atomic resolution information for protein interactions in the native solution state. First, backbone assignment of Nup358-min was carried out using standard triple resonance experiments, 3D HNCO (Kay et al., 1990), HNCACO (Clubb et al., 1992), HNCA (Kay et al., 1990), HNCACB (Grzesiek and Bax, 1992), and CBCACONH (Grzesiek and Bax, 2002). 82 of the 89 total backbone amides were assigned in the 1H-15N HSQC (Figure 3—figure supplement 1). Then, 15N-labeled Nup358-min was titrated with unlabeled BicD2-CTD. Figure 3a shows the overlay of the HSQC spectrum of apo Nup358-min with that of the complex, for which 15N-labeled Nup358-min and unlabeled BicD2-CTD were mixed at a 1:1 molar ratio. The HSQC spectrum of the apo Nup358-min is characterized by a lack of dispersion, consistent with an IDP (Figure 1—figure supplement 1, Figure 3—figure supplement 1). Analysis of backbone chemical shifts (CA, CB, CO, N, and HN) of Nup358-min by TALOS-N (Shen and Bax, 2013) conclusively demonstrates that Nup358-min is a random coil in the apo state (Figure 3—figure supplement 3). Although the addition of BicD2-CTD resulted in little peak movement in the HSQC, significant peak intensity changes were observed for the N-terminal half of Nup358-min (Figure 3a and b). This points to a relatively wide binding region undergoing intermediate to slow exchange on the NMR time scale due to ligand binding.
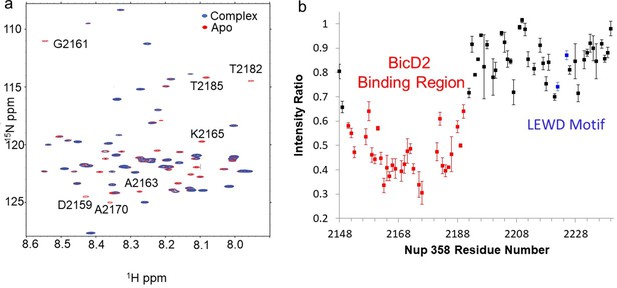
Nuclear magnetic resonance (NMR) titration mapped the BicD2-binding site to the N-terminal half of Nup358-min.
NMR mapping of Nup358 regions involved in BicD2-CTD binding was performed by titration of 15N-labeled Nup358-min with BicD2-CTD. (a) The HSQC spectrum of a 1:1 Nup358-min/BicD2-CTD complex (blue) is overlaid on that of apo-Nup358-min (red). The full HSQC assignment is shown in Figure 3—figure supplement 1. Many peaks disappeared in the complex spectrum (selected peaks labeled by residue name and number), indicating that BicD2 binding causes slow to intermediate chemical exchange on the NMR time scale. (b) Plot of the peak intensity change vs. the residue number of Nup358. The peak intensities I, as determined by peak heights, of the 1:1 BicD2-CTD/Nup358-min complex spectrum were divided by the peak intensities of the apo-Nup358 spectrum (I0) to obtain I/I0. Data points with an I/I0 of 0.65 or lower are colored red. This plot shows that the N-terminal half of Nup358-min is largely responsible for BicD2 binding. The peak intensities corresponding to the LEWD sequence motif (colored blue) in Nup358, which mediates binding of KLC2, are not affected by BicD2 binding. For the full titration results, please see Figure 3—figure supplement 2.
-
Figure 3—source data 1
Source data for NMR titration plot.
- https://cdn.elifesciences.org/articles/74714/elife-74714-fig3-data1-v4.xlsx
The Nup358-min domain also contains the previously published KLC2-binding LEWD motif (Cui et al., 2019), but the BicD2-binding site determined here is separated from the LEWD motif by over 30 residues (Figure 3b). Residues 2192–2240 of Nup358 show little change in NMR signal between the apo sample and the complex (Figure 3b), indicating that the LEWD motif is not involved in BicD2 binding. Therefore, kinesin-1 and the dynein adaptor BicD2 may bind to separate but spatially close binding sites on the cargo Nup358. The proximity of these motor recognition sites may serve to enable the interaction between dynein and kinesin machineries for the regulation of bidirectional motility and precise positioning of the nucleus.
CEST suggests a coil-to-α-helix transition at the BicD2-Nup358 interface
Although our results from the HSQC titrations establish the general region of the BicD2 interface in Nup358, the chemical shifts of the bound state were not obtained due to peak disappearance in the HSQC upon BicD2 addition. To further characterize the BicD2/Nup358 interface with NMR, chemical exchange saturation transfer (CEST) experiments were performed. CEST has recently emerged as a powerful technique in solution NMR for measuring the chemical shifts of NMR invisible states (Vallurupalli et al., 2012; Charlier et al., 2017), such as the BicD2-CTD-bound state of Nup358-min, whose resonances are absent from the 15N-HSQC (Figure 3a and b). In CEST, when the invisible state is saturated by a weak and long radiofrequency (RF) pulse (B1), the saturation of the bound state will be transferred to the free state due to Nup358-min dissociating from the Nup358-min-BicD2 complex, causing a dip in peak intensity when B1 is on resonance with the bound state chemical shift, generating the minor dip in the CEST curve. If the chemical shifts of bound and unbound state are significantly different, which is, for example, the case for interface residues or residues that undergo structural changes upon complex formation, this difference gives rise to a double-dip appearance in the CEST profile (Figure 4c and d). The major dip in the profile will be observed at the chemical shift of the free or unbound state (when the B1 matches the chemical shift of unbound resonance, the ‘visible state’). The second, smaller dip in the profile peak will be observed at the chemical shift of the bound state (due to saturation transfer from the ‘invisible state’). In contrast, residues that are not located at the complex interface or do not undergo structural changes upon complex formation will result in resonances without chemical exchange and the CEST profile will only show a single dip (Figure 4a and b), corresponding to the chemical shift of the unbound state.
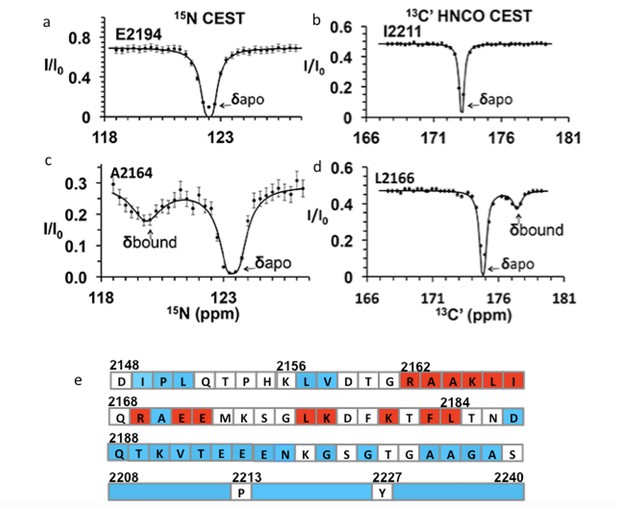
Chemical exchange saturation transfer (CEST) maps chemical shifts of nuclear magnetic resonance (NMR)-invisible, BicD2-bound state of Nup358.
In the CEST profile curve, E2194 and I2211 have only a single dip in 15N-CEST (a) and 13C′-CEST (b), respectively, due to little chemical shift perturbation upon BicD2-CTD binding. This suggests that E2194 is not at the binding interface and does not undergo conformational transition upon BicD2-CTD binding. In contrast, A2164 shows not only a dip at the chemical shift of the apo HSQC assignment, but also a smaller dip at about 3 ppm to the left in 15N-CEST (c), indicating that A2164 is in the binding region and/or experiences a conformational transition upon complex formation. (d) L2166 has a major peak and then a minor peak about 2.5 ppm to the right. The magnitude and direction of the chemical shift difference suggest a transition from a random coil to an α-helix (see Table 1). (e) Summary of the CEST data obtained across the entire Nup358-min region. Red squares show residues with double dip in CEST profile. Their chemical shift changes from the apo to the bound state indicate that these red residues are part of the α-helical region at the Nup358/BicD2 interface. Blue-colored residues show a single dip in CEST profile, suggesting that these remain in random coil conformation. White represents the residues where data were not obtained due to low signal-to-noise ratio or resonance overlap. All the curves with double dips and a sampling of curves with only single dip fits are shown in Figure 4—figure supplements 1–4.
-
Figure 4—source data 1
Source Data for CEST Curves.
- https://cdn.elifesciences.org/articles/74714/elife-74714-fig4-data1-v4.xlsx
Chemical shift differences from chemical exchange saturation transfer (CEST) of Nup358/BicD2 and apo-Nup358 (Δδbound-apo) match closely to Δδ for coil-to-α-helix transition (Δδhelix-coil)‡.
Residue | Δδbound-apo | Δδhelix-coil |
---|---|---|
A2163* | –3.6 | –2.2 |
A2164* | –3.6 | –2.2 |
K2165* | –0.8 | –1.3 |
L2166* | –3.8 | –1.9 |
I2167* | –5.7 | –1.2 |
K2178* | –3.6 | –1.3 |
L2184* | –5.5 | –1.9 |
R2162† | 1.7 | 2.3 |
A2164† | 2.8 | 1.7 |
K2165† | 2.7 | 2.1 |
L2166† | 2.6 | 1.6 |
R2169† | 2.9 | 2.3 |
E2171† | 2.2 | 2.2 |
E2172† | 3.0 | 2.2 |
L2177† | 0.9 | 1.6 |
K2181† | 3.0 | 2.1 |
F2183† | 3.3 | 1.5 |
L2184† | 1.0 | 1.6 |
-
See also Appendix 1—table 1.
-
CD spectroscopy confirms formation of an α-helix in Nup358 upon binding to BicD2.
-
*
Change in chemical shifts of amide 15N.
-
†
Change in chemical shifts of carbonyl 13C.
-
‡
Values taken from Wishart, 2011.
We performed CEST experiments on both the 15N amide and the 13C carbonyl (C′) resonances. In 15N CEST NMR experiments, the following residues were identified as interface residues or residues that undergo structural transition because of the double-dip appearance in the CEST profile: A2163, A2164, K2165, L2166, I2167, K2178, and L2184 (Figure 4—figure supplement 1). For these residues, CEST indicates a significant chemical shift difference between their bound and unbound states. Using the program RING NMR Dynamics (Beckwith et al., 2020), the CEST data were fit to obtain chemical shift difference between free and bound state (Δδ), the exchange rate (kex), and the fractional minor population (Pb) (see Table 1, Appendix 1—table 1a and b). The weighted average of the 15N CEST fit parameters resulted in kex = 200 ± 70 s–1, consistent with slow to intermediate exchange (kex < Δω) on an NMR time scale. The weighted average of the minor population (Pb) is 0.04 ± 0.01, which is consistent with our sample preparation at a molar ratio of 1:20 for BicD2-CTD:15N-Nup358-min. Many peaks in the 15N-CEST suffered from low signal-to-noise ratio and resonance overlap common in IDPs, preventing the determination of additional chemical shifts of invisible states. To overcome this problem, we further carried out 13C′-CEST experiments based on HNCO, which correlates amides (HN) with the carbonyl (CO) of the preceding residue. Here, the saturation pulse B1 is on the carbonyl, and the intensity change due to saturation is still reported in an 15N-HSQC-like 2D spectrum. This method provided additional data for residues missed with the 15N CEST due to resonance overlap or low signal-to-noise ratio. For example, the F2183 peak in 15N-1H HSQC was overlapped. However, the L2184 peak, a well-resolved signal with high S/N, showed a CEST effect from the saturation of the F2183 carbonyl in HNCO-based 13C′-CEST. With the 13C HNCO-CEST experiment, we were able to observe minor states in the following residues: R2162, A2164, K2165, L2166, R2169, E2171, E2172, L2177, K2181, F2183, and L2184 (Figure 4—figure supplement 2, Appendix 1—table 1c). Thus, with CEST, we were able to further map the binding region to residues 2162–2184 (Figure 4e). Fitting of HNCO-CEST profiles with RING Dynamics resulted in a global exchange rate of 270 ± 50 s–1, in reasonable agreement with the value of kex from 15N CEST. kex obtained here is much slower in time scale than the rate of coil-to-α-helix transition in model peptides (Wang et al., 2004). This suggests that the kex obtained by CEST include contributions from other events, such as binding and oligomerization, in addition to coil-to-α-helix transition, consistent with similar observations for other binding studies (Charlier et al., 2017). The weighted average of the minor population (Pb) is 0.06 ± 0.01, which is again consistent with the ratio in which we mixed the two proteins for the NMR sample. The 18 CEST profiles with double-dip appearance all resulted in chemical shift changes in the direction and magnitude as expected for a coil-to-α-helix transition (Wishart, 2011) (typically the chemical shift for 15N becomes lower in the helical conformation, and higher for 13C, as shown in Table 1). The CEST results suggest that residues 2162–2184 of Nup358 undergo coil-to-α-helix transition upon binding to BicD2, revealing that BicD2 recognizes Nup358 through a short ‘cargo recognition α-helix,’ which is embedded in an IDP domain.
To provide further evidence for the coil-to-α-helix transition, CD spectroscopy was carried out. The CD wavelength scans of the Nup358-min/BicD2-CTD complex had minima at 208 nm and 222 nm, characteristic for α-helical proteins (Noell et al., 2019; Figure 5). Such minima were absent in the CD spectra of Nup358-min as expected for an IDP. Notably, the minima at 208 and 222 nm were shifted towards more negative values in the complex compared to the sum of the individual spectra of Nup358-min and BicD2-CTD, suggesting that the α-helical content increases upon complex formation.
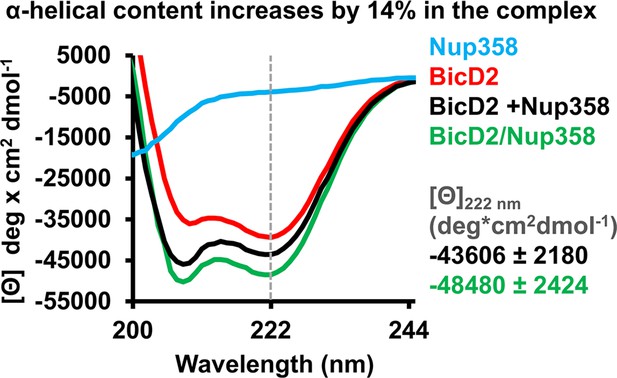
Circular dichroism (CD) spectroscopy confirms formation of an α-helix in the Nup358/BicD2 complex.
CD wavelength scans of BicD2-CTD (red), Nup358-min (blue), and the Nup358-min/BicD2 complex (green) at 4°C are shown. The sum of the individual wavelength scans of Nup358-min and BicD2-CTD is shown in black. The mean residue molar ellipticity [Θ] versus the wavelength is shown. Experiments were repeated two or three times, and representative scans are shown. A characteristic feature of α-helical structures is a local minimum at 222 nm (dashed line). Based on the values for [Θ] at 222 nm (shown on the right) and based on our calibration curve (Figure 5—figure supplement 1), the Nup358/BicD2 complex has a 14% ± 5% increase in α-helical content compared to the sum of the spectra of BicD2 and Nup358. See Figure 5—figure supplement 1 and Figure 5—source data 1.
-
Figure 5—source data 1
CD spectra.
- https://cdn.elifesciences.org/articles/74714/elife-74714-fig5-data1-v4.xlsx
To quantify the increase of the α-helical content, we determined the difference between the molar ellipticity at 222 nm of the complex and of the sum of the individual proteins, Δ[Θ], to be –4874 deg cm2/dmol (Figure 5), which corresponds to a 14% ± 5% increase of the α-helical content based on a published thermal unfolding curve of the BicD2-CTD, which was used for calibration (Noell et al., 2019; Figure 5, Figure 5—figure supplement 1, and Cui et al., 2020). We recently determined the experimental error to be ~5% (corresponding to Δ[Θ] of 2930 deg cm2/dmol) (Cui et al., 2020); therefore, we estimate that the α-helical content increases by 14% ± 5% upon Nup358/BicD2 complex formation, confirming a structural transition from a coil to an α-helix.
The minimal Nup358/BicD2 complex has a rod-like shape that is more compact than the individual proteins
Small-angle X-ray scattering (SAXS) experiments were carried out to obtain a low-resolution structure of the Nup358-min/BicD2-CTD complex. The quality of our SAXS data was confirmed by molar mass calculations: for Nup358-min, we determined a molar mass of 12.3 kDa (Appendix 2—table 1), which matches closely to the calculated mass of a monomer (10.6 kDa). For the Nup358-min/BicD2-CTD complex, we determined a molar mass of 47.6 kDa (Appendix 2—table 1), which matches closely to the expected mass of a Nup358-min/BicD2-CTD complex with a 2:2 stoichiometry (calculated molar mass of 43.0 kDa). These findings are in line with our previously published SEC-MALS data, which suggest that apo Nup358-min forms monomers, while the Nup358/BicD2 complex has a 2:2 stoichiometry (Cui et al., 2019; Noell et al., 2018).
A Kratky plot of the SAXS profiles further confirmed that Nup358-min is intrinsically disordered as the signal increases at high q values instead of approaching zero (Figure 6a). In contrast, the Kratky plots of the BicD2-CTD and the Nup358-min/BicD2-CTD are bell-shaped, suggesting that they are folded (Figure 6a). Thus, Nup358-min becomes more compact upon complex formation with BicD2-CTD, consistent with the coil-to-α-helix transition observed from NMR and CD spectroscopy.
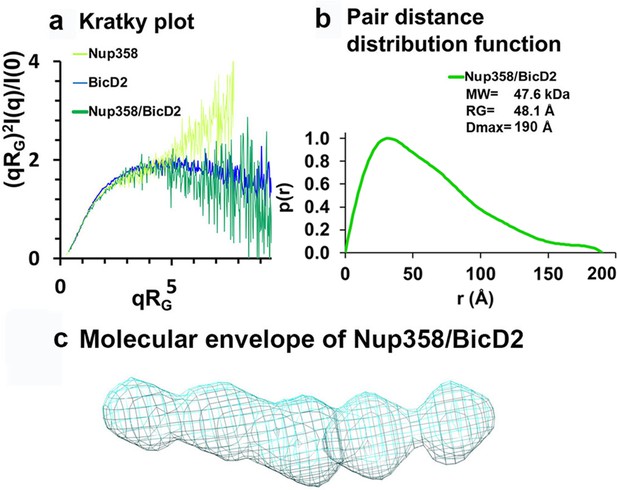
Low-resolution structures determined by small-angle X-ray scattering (SAXS) confirm that the complex has a rod-like shape that is more compact than the individual proteins.
(a) Dimensionless Kratky plots of the SAXS data collected from the minimal Nup358/BicD2 complex, from Nup358-min and BicD2-CTD (q: scattering vector; RG: radius of gyration; I(q): scattering intensity). (b) The pair distance distribution function p(r) of the Nup358-min/BicD2-CTD complex was derived from the scattering intensity profile (Figure 6—figure supplement 1). The molecular weight MW (Rambo and Tainer, 2013), the radius of gyration RG from the Guinier plot, and the largest dimension of the particle Dmax are shown. (c) Refined bead model 3D reconstruction of the Nup358-min/BicD2-CTD complex (cyan mesh). The statistical analysis and supporting SAXS data are summarized in Figure 6—figure supplement 1–4 and Appendix 2. See also Figure 6—source data 1.
-
Figure 6—source data 1
Small-angle X-ray scattering (SAXS) data.
- https://cdn.elifesciences.org/articles/74714/elife-74714-fig6-data1-v4.zip
The pair distance distribution function p(r) derived from the SAXS profile of the Nup358-min/BicD2-CTD complex has a peak that decays with a linear slope, which is characteristic of elongated, rod-like structures (Figure 6b). Since the width of a rod remains similar throughout its length, the sum of all pair distances will have a linear slope. The maximum particle diameter DMax of the complex is 190 Å, which is identical to the DMax that was determined for the BicD2-CTD sample (Appendix 2—table 1), suggesting that the overall length of the rod structure does not change. Bead models of the Nup358-min/BicD2 complex were reconstructed from the p(r) functions and confirm that the complex has a flexible, rod-like structure (Figure 6c). The normalized spatial discrepancy (NSD) is 0.7, suggesting the structural convergence and homogeneity of bead models reconstructed from SAXS profiles (Appendix 2—table 2).
Taken together, these SAXS data suggest that the complex has a 2:2 stoichiometry and is more compact than the apo state, further validating the coil-to-α-helix transition in the Nup358/BicD2 complex.
Nup358 mutagenesis validates α-helix formation at the Nup358/BicD2 interface
Our CEST, CD, and SAXS data provided strong evidence that residues 2162–2184 of Nup358 undergo a structural transition from random coil-to-α-helix upon complex formation with BicD2.
To confirm our results, we also carried out alanine mutagenesis for all residues of the Nup358 α-helix and assessed binding of the mutated GST-tagged Nup358-min to BicD2-CTD by pull-down assays (Figure 7, Figure 7—figure supplement 1). Eleven mutants displayed diminished binding, which are interspaced throughout the α-helix. An α-helical wheel representation was created, which revealed that these interface residues are clustered on one side of the α-helical wheel (Figure 7c, red). The mutagenesis experiments are consistent with our results from NMR spectroscopy. While interface residues and residues that undergo the transition from coil-to-α-helix will show a chemical shift change, not all residues in the newly formed α-helix in Nup358-min will be at the binding interface; therefore, the mutagenesis data provides additional information. Notably, all interface residues that were identified from mutagenesis (Figure 7d, red) also had a double dip in the CEST profile (Figure 7d, red) or were not assessed (Figure 7d, white). Furthermore, removal of residues 2163–2166 (AAKL) of Nup358-min (ΔAAKL), which had double dips in CEST curves, virtually abolishes the interaction with the BicD2-CTD (Figure 7—figure supplement 1b). We also removed the α-helix from Nup358-min, and as expected, the resulting Nup358-fragment (residues 2185–2240) shows virtually no interaction with the BicD2-CTD (Figure 7—figure supplement 1a, last lane). Finally, we successfully assembled a minimal complex of the BicD2-CTD with a Nup358 fragment (residues 2158–2199) that only contained the BicD2-binding site deduced from NMR, which confirmed our mapped binding site (Figure 7—figure supplement 1c). Furthermore, known BicD2 residues that are important for Nup358 interaction include L746, R747, M749, and R753 (Terawaki et al., 2015), suggesting a potential binding site for the Nup358 α-helix in the center of the BicD2-CTD coiled coil (Figure 7e).
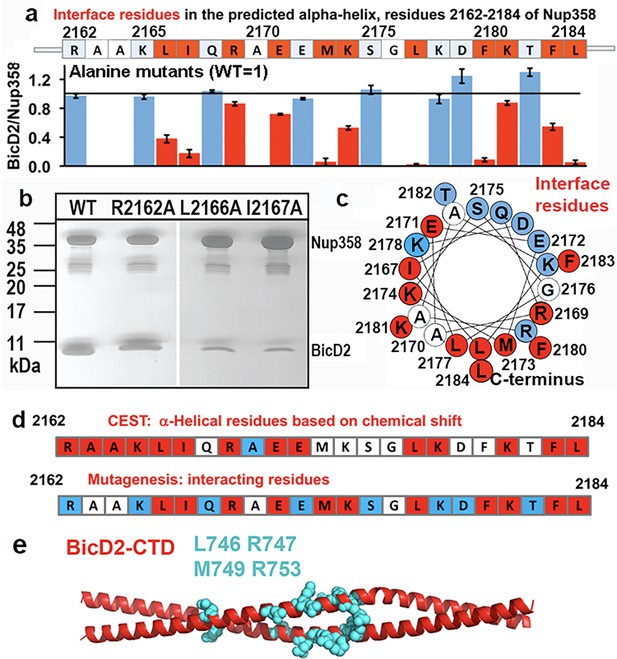
Mutagenesis of the Nup358 cargo recognition helix.
All residues of the Nup358 α-helix were mutated to alanine, and binding was assessed by GST-pull-down assays with purified Nup358-min-GST followed and BicD2-CTD. The elution fractions were analyzed by SDS-PAGE, and the intensities of the gel bands were quantified to obtain the ratio of bound BicD2-CTD/Nup358-min, normalized respective to the wild-type (Figure 7—figure supplement 1). The binding ratio was averaged from three independent experiments, and the error was calculated as the standard deviation. Eleven interface residues were identified, which are colored red and show a reduction of the binding ratio upon mutation by at least three times the standard deviation. In addition, interface residues were required to have a binding ratio that was reduced by 13% or more compared to the wild-type. Alanine and glycine residues were not assessed and are colored white. Residues for which mutations do not affect binding are colored light blue. (a) The sequence of the predicted Nup358 α–helix is shown above a bar graph of the ratios of bound BicD2 to Nup358 from the alanine mutant pull-down assays (WT = 1, indicated by the horizontal black line). (b) Representative SDS-PAGEs of elution fractions of the pull-down assays. A representative full dataset is shown in Figure 7—figure supplement 1. Note that a small gel band at 25 kDa represents GST. (c) Helical wheel representation for (a). (d) Comparison of helical residues in Nup358-BicD2 complex identified by chemical exchange saturation transfer (CEST) and results from pull-down assay of mutants. Red: CEST-positive (double dip in CEST profile) or strong reduction in binding from the pull-down assay with alanine mutation at this residue; blue: CEST-negative (single dip in CEST profile) or no effect in mutagenesis; white: data not available. (e) The structure of the Hs BicD2-CTD (Noell et al., 2019) is shown in red cartoon representation. Four Nup358/BicD2 interface residues that were previously identified by mutagenesis are shown in cyan spheres representation (Terawaki et al., 2015). See also Figure 7—figure supplement 1 and Figure 7—source data 1–3.
-
Figure 7—source data 1
Quantification of the pull-down assay.
- https://cdn.elifesciences.org/articles/74714/elife-74714-fig7-data1-v4.xlsx
-
Figure 7—source data 2
SDS-PAGE shown in Figure 7.
- https://cdn.elifesciences.org/articles/74714/elife-74714-fig7-data2-v4.png
-
Figure 7—source data 3
SDS-PAGEs shown in Figure 7—figure supplement 1 and SDS-PAGEs that were used for the quantification shown in Figure 7.
- https://cdn.elifesciences.org/articles/74714/elife-74714-fig7-data3-v4.zip
Nup358-BicD2 interface is essential for dynein activation
Five mutants that showed a reduced Nup358-min/BicD2-CTD interaction in the pull-down assays were selected for further characterization. Single-molecule-binding and processivity assays were used to further explore the impact of Nup358 mutants on dynein recruitment and activation of dynein motility. We assessed the interaction between BicD2 and the following Nup358min-zip mutants: I2167A, M2173A, L2177A, F2180A, and L2184A, in the context of the BNmin-zip and the DDBNmin-zip complex. The following parameters were quantified for WT and mutant Nup358min-zip constructs: (a) the number of single-molecule processive events of DDBNmin-zip on MTs, (b) the percent complex formation with BicD2 alone or with the DDB complex, and (c) the speed and run length of DDBNmin-zip on MTs.
The number of processively moving DDBNmin-zip complexes on MTs was quantified as the number of dual-color Qdots moving per min per micrometer MT length (Figure 8a). The processive events of DDBNmin-zip formed with the Nup358min-zip mutants I2167A, M2173A, F2180A, and L2184A Nup358min-zip were significantly lower than that formed with WT-Nup358min-zip (Figure 8a). DDBNmin-zip formed with the Nup358min-zip-L2177A mutant did not result in a lower number of processively moving complexes, suggesting that there is some plasticity at the Nup358/BicD2 interface.
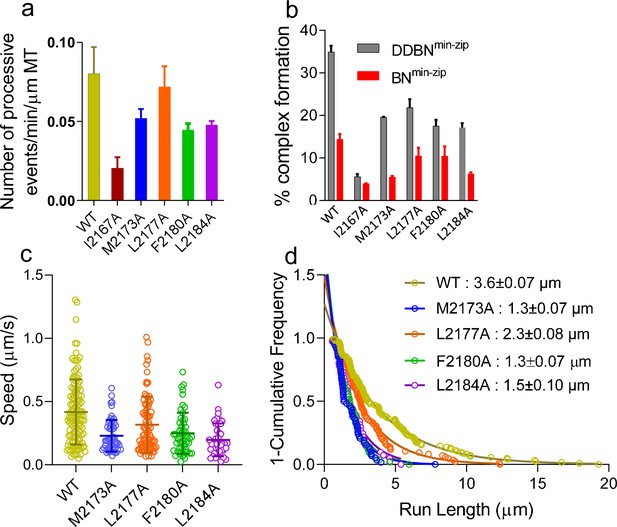
Nup358 point mutations that diminish the interaction with BicD2-CTD also diminish the formation of the DDBN complex.
(a) Bar graph of the processive events of DDBNmin-zip complexes per min per micrometer MT length. The number of processive events of DDBNmin-zip complexes formed with WT-Nup358min-zip is significantly higher (p<0.0001) than those formed with the Nup358min-zip mutants I2167A, M2173A, F2180A, and L2184A but the same as with mutant L2177A (orange, p=0.09). The number of processive events of DDBNmin-zip formed with WT-Nup358min-zip was 0.080 ± 0.0017/min/µm MT (n = 3, with n being the number of experiments). In contrast, these values for DDBNmin-zip formed with the Nup358min-zip mutants I2167A, M2173A, L2177A, F2180A, and L2184A were 0.020 ± 0.007/min/µm (n = 2), 0.052 ± 0.006/min/µm (n = 2), 0.072 ± 0.013min/µm (n = 3), 0.044 ± 0.005/min/µm (n = 2), 0.048 ± 0.0023/min/µm (n = 2) respectively. (b) The presence of dynein-dynactin (gray) increases the formation of BicD2-Nup358min-zip complexes (red). The percent formation of BNmin-zip was 14.41% (n = 580) for WT, 3.96% (n = 1142) for I2167A, 5.54% (n = 1877) for M2173A, 10.49% (n = 948) for L2177A, 10.47% (n = 412) for F2180A, and 6.31% (n = 1032) for L2184A Nup358min-zip. N is the total number of quantum dots (Qdots) counted. In contrast, these values for DDBNmin-zip complexes were 34.92% (n = 945), 5.62% (n = 986), 19.61% (n = 347), 21.87% (n = 778), 17.52% (n = 890), and 17.13% (n = 372), respectively. (c) Comparison of the speeds of DDBN complexes formed with WT-Nup358min-zip and mutant Nup358min-zip constructs. The speed of DDBN formed with Nup358min-zip mutants M2173A (0.23 ± 0.13 µm/s, n = 58; blue), L2177A (0.32 ± 0.22 µm/s; n = 86; orange), F2180A (0.25 ± 0.16 µm/s, n = 54; green), and L2184A (0.20 ± 0.13 µm/s, n = 41; purple) are significantly slower than that of DDBN-WT (p<0.0001 for mutants M2173A, F2180A, L2184A and p=0.0058 for L2177A), one-way ANOVA followed by Tukey’s test. (d). The run length of DDBNmin-zip formed with Nup358min-zip-M2173A (1.3 ± 0.07 µm, n = 58), L2177A (2.3 ± 0.08 µm, n = 86), F2180A (1.3 ± 0.07 µm, n = 54), and L2184A (1.5 ± 0.10 µm, n = 41) are significantly shorter than that formed with WT-Nup358min-zip (3.6 ± 0.07 µm; n = 123; data taken from 2 hr) (with p<0.0001 for mutants M2173A, F2180A, L2184A and p=0.0036 for L2177A; one-way ANOVA followed by Tukey’s test). See also Figure 8—figure supplement 1.
-
Figure 8—source data 1
The number of processive events of DDBNmin-zip complexes formed with WT-NUP358min-zip compared with DDBNmin-zip formed with NUP 358min-zip mutants I2167A, M2173A, F2180A, and L2184A.
- https://cdn.elifesciences.org/articles/74714/elife-74714-fig8-data1-v4.xlsx
-
Figure 8—source data 2
The presence of dynein-dynactin increases the formation of BicD2-NUP358min-zip complexes.
- https://cdn.elifesciences.org/articles/74714/elife-74714-fig8-data2-v4.xlsx
-
Figure 8—source data 3
Comparison of the speeds of DDBN complexes formed with WT-NUP358min-zip and mutant NUP358min-zip constructs.
- https://cdn.elifesciences.org/articles/74714/elife-74714-fig8-data3-v4.xlsx
-
Figure 8—source data 4
Comparison of the run lengths of DDBN complexes formed with WT-NUP358min-zip and mutant NUP358min-zip.
- https://cdn.elifesciences.org/articles/74714/elife-74714-fig8-data4-v4.xlsx
We next assessed how these Nup358min-zip mutants affected the BicD2-Nup358min-zip (BN) interaction in the absence or presence of DD. BicD2 and WT or mutant Nup358min-zip were labeled with different color Qdots, and the number of dual-color BNmin-zip and DDBNmin-zip complexes nonspecifically bound to a coverslip was quantified using the total internal reflection fluorescence (TIRF) microscopy (Figure 8—figure supplement 1). Nup358min-zip binds BicD2 alone moderately well, and the percentage of BN was reduced further for the Nup358min-zip mutants (Figure 8b, red). We also quantified how the presence of DD influences the BicD2-Nup358min-zip interaction. Strikingly, in the presence of DD, the formation of DDBNmin-zip complexes was about two times higher than the BN complexes (Figure 8b, gray). The mutant Nup358min-zip again showed reduced complex formation relative to WT (Figure 8b, gray). The difference between DDBNmin-zip and BNmin-zip indicates that DD enhances the BicD2-Nup358 interaction.
The motion of DDBNmin-zip complexes formed with the five Nup358min-zip mutants was quantified and compared with DDBNmin-zip containing WT-Nup358min-zip. DDBNmin-zip complexes with Nup358min-zip mutants showed slower speed and shorter run length compared with WT-DDBNmin-zip (Figure 8c and d). There were too few dual-colored complexes formed with the I2167A mutant to allow speed and run length quantification. Compromised motility in the presence of the five characterized Nup358min-zip mutants suggests the formation of a less stable DDBN complex, even for the L2177A mutant that showed a similar number of processive runs as WT Nup358min-zip. The functional importance of the Nup358 cargo recognition-α-helix is validated by the impaired motility observed with point mutants of this α-helix.
Discussion
Here, we elucidate the molecular details of the interface between the dynein activating adaptor BicD2 and the nuclear pore protein Nup358, which links BicD2 to the cell nucleus. First, we reconstituted a minimal Nup358-min/BicD2-CTD complex with μM affinity. Single-molecule processivity assays revealed that a dimerized Nup358-min domain forms a complex with dynein/dynactin/BicD2 (DDBN) that shows robust processive motility on microtubules. Similarly, the small GTPase Rab6aGTP that links BicD2 to membranous cargo promotes processive motility (Huynh and Vale, 2017), suggesting that both adaptor-binding proteins act to release the autoinhibition of BicD2. Dimerization of Nup358-min enhances interaction with BicD2 and with the DDB complex, suggesting that activation is linked to oligomerization, which for Rab6GTP could occur by clustering on the vesicular membrane.
NMR titration of BicD2-CTD into 15N labeled Nup358-min mapped the binding region to the N-terminal half of Nup358-min. Furthermore, we obtained chemical shifts for the CA and CB atoms for the apo state of Nup358-min, which confirm that the apo state is intrinsically disordered. Due to slow chemical exchange on the NMR time scale and fast relaxation in the BicD2-bound state of Nup358-min, this state cannot be directly observed in standard NMR experiments, and thus a powerful solution NMR technique termed CEST was applied to map the chemical shifts of the ‘invisible state.’ This approach not only identified key residues at the Nup358/BicD2 interface, but also showed that chemical shift changes upon binding are consistent with a coil-to-α-helix transition in Nup358-min. Notably, the coil-to-α-helix conformational change is supported by our results from CD spectroscopy and SAXS, and the BicD2/Nup358 interface was validated by mutagenesis. The observed time scale is also in line with other proteins undergoing coil-to-α-helix transitions (Charlier et al., 2017). However, it should be noted that in order to obtain higher-resolution structural information, the chemical shifts for the CA and CB atoms of Nup358-min in the bound state remain to be determined in the future. Notably, our functional assays highlight the important role of the interaction between Nup358 and BicD2 in dynein activation. Single-molecule-binding assays showed that mutagenesis of the Nup358 cargo recognition α-helix diminished the interaction of Nup358-min with full-length BicD2 and decreased the formation of dynein/dynactin/BicD2/Nup358 complexes (DDBN). Furthermore, the mutant DDBN complexes that were formed exhibited reduced run length and speed reflecting formation of a less stable complex. Our data highlight the important role of dynein activating-adaptor/adaptor-binding protein interactions in activating and fine-tuning dynein motility.
Our data establish that BicD2 recognizes its cargo-binding protein Nup358 through an α-helix of ~28 residues. Coil-to-α-helix transitions and folding upon binding, as shown here, have been observed in many studies of IDP/IDR interactions and recognition (Celestino et al., 2019; Charlier et al., 2017). We propose that the dynein activating-adaptor BicD2 recognizes its cargoes through a ‘cargo recognition α-helix’ (Figure 9). It is conceivable that the cargo adaptors Rab6GTP and nesprin-2G are also recognized by similar short α-helices, although this needs to be confirmed by future experiments. Rab6GTP binds to BicD2 with 10-fold higher affinity compared to Rab6GDP (Matanis et al., 2002; Bergbrede et al., 2009; Garcia-Saez et al., 2006) and associated structural changes are found in the Switch I and Switch II regions of Rab6 (Garcia-Saez et al., 2006), which include short α-helices and intrinsically disordered domains and are possibly candidates for the BicD2-binding site. The mapped nesprin-2G-binding domain for BicD2 is also predicted to be largely intrinsically disordered (Zhu et al., 2017). Our data thus suggest a structural basis for cargo recognition by BicD2 and will enable further studies aimed at identifying cell cycle-specific regulatory mechanisms for distinct transport pathways that are facilitated by BicD2 (Splinter et al., 2010; Gonçalves et al., 2020; Matanis et al., 2002). For example, our Nup358 mutants that selectively target the interaction with BicD2 can be used to dissect biological roles of the Nup358/BicD2, Rab6/BicD2, and nesprin 2G/BicD2 pathways in distinct stages of brain development.
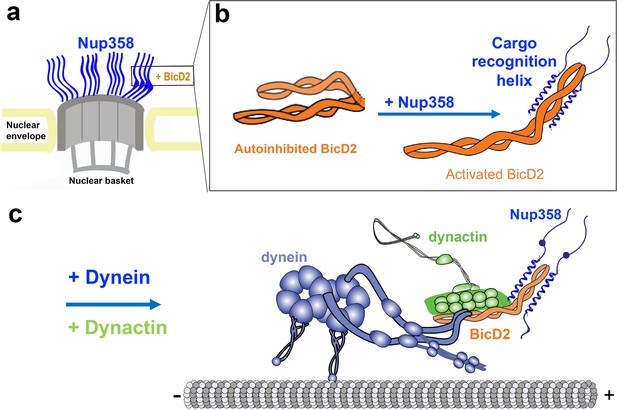
We propose that BicD2 recognizes its cargo through a short ‘cargo recognition α-helix,’ which may also be a structural feature that stabilizes the activated state of BicD2 for the recruitment of dynein and dynactin.
(a) Cutaway view of half of an NPC. Each of the eight spokes of the NPC contains four molecules of Nup358 on the cytoplasmic side (i.e., 32 in total), which provide binding sites for dynein (via BicD2) and kinesin-1 (via KLC2). Nup358 makes up most of the mass of the cytoplasmic filaments of the NPC (von Appen et al., 2015; Ori et al., 2013). (b) Left panel: schematic representation of the looped, autoinhibited conformation of BicD2 that is formed in the absence of cargoes such as Nup358. Apo-Nup358 contains many intrinsically disordered regions (IDR), including the BicD2-binding region. Upon binding of Nup358 to BicD2, a short α-helix is formed at the Nup358/BicD2 interface, which is a structural feature that stabilizes BicD2 in the active state. Binding of Nup358 to BicD2 likely promotes loop opening, which activates BicD2 for dynein/dynactin recruitment (Sladewski et al., 2018). (c) Schematic representation of the proposed dynein/dynactin/BicD2/Nup358 complex (DDBN) bound to microtubules for processive motility for nuclear positioning. Nup358 has a LEWD motif that recruits kinesin-1 via KLC2. The binding sites for the opposite polarity motor kinesin-1 on Nup358 are indicated by the small blue sphere. The location of the LEWD motif that recruits kinesin-1 via KLC2 is indicated. We propose that BicD2 and KLC2 interact simultaneously with Nup358 for precise control of nuclear positioning.
A key step in activating dynein motility is overriding the autoinhibited conformation of BicD2 to allow dynein recruitment. We and others have recently proposed that cargo binding activates BicD2 for dynein recruitment by inducing a coiled-coil registry shift in BicD2 (i.e., a vertical displacement of the two α-helices against each other by one helical turn) (Liu et al., 2013; Terawaki et al., 2015; Noell et al., 2019; Cui et al., 2020). In the absence of cargo, BicD2 forms an autoinhibited state that cannot recruit dynein, with the CTD masking the N-terminal dynein/dynactin-binding site (NTD). When cargo is loaded, BicD2 can directly link dynein with its activator dynactin, which is required to activate dynein for processive motility (Splinter et al., 2012; Schlager et al., 2014b; Schlager et al., 2014a; Hoogenraad et al., 2003; Hoogenraad et al., 2001; McKenney et al., 2014; Urnavicius et al., 2018; Urnavicius et al., 2015; Sladewski et al., 2018; McClintock et al., 2018; Liu et al., 2013; Cui et al., 2020). We recently showed that an F684I mutant of the Drosophila (Dm) homolog BicD can activate dynein/dynactin for processive motility in the absence of cargo. X-ray structures of the WT and F684I mutant as well as molecular dynamics simulations suggest that this activating mutation causes a coiled-coil registry shift in the Dm BicD-CTD (Noell et al., 2019; Cui et al., 2020). It is tempting to hypothesize that the cargo recognition helix of Nup358 intercalates into the coiled-coil of the BicD2-CTD to stabilize a coiled-coil registry shift in the Nup358/BicD2 complex, resulting in activation of BicD2 for dynein recruitment. This hypothesis remains to be experimentally confirmed.
Nup358-min binds more strongly to the dynein/dynactin/BicD2 complex compared to full-length BicD2 alone (Figure 8b). This observation supports the proposed loop-opening activation mechanism of BicD2 (Hoogenraad et al., 2001; Sladewski et al., 2018; McClintock et al., 2018; Liu et al., 2013; Cui et al., 2020; Figure 9b and c). In the full-length dynein/dynactin/BicD2/Nup358 (DDBN) complex, once Nup358 binding displaces the autoinhibited loop, dynein and dynactin bind and prevent its reformation. This is not true in the full-length BicD2/Nup358 complex, resulting in a lower apparent affinity than in the full complex. It is also possible that additional weak interfaces further stabilize the DDBN complex compared to Nup358/BicD2 or the DDBCC1 complex. Here, we observed a direct interaction of Nup358 with DD that could contribute to enhanced affinity. It has been shown for several cargo adaptors that they interact weakly with the dynein light chains (Tai et al., 1999) and the dynein LIC1 forms a small interface with the so-called CC1 box of BicD2, which is required for the activation of processive motility (Celestino et al., 2019; Lee et al., 2020; Sweeney and Holzbaur, 2018). Cargoes and adaptors often interact through multiple interfaces with motors, and these additional interfaces in many cases enhance overall motility (Fu and Holzbaur, 2014; Urnavicius et al., 2018; Urnavicius et al., 2015; Celestino et al., 2019; Lee et al., 2018; Sanger et al., 2017; Blasius et al., 2007).
The affinity of the monomeric cargo Nup358-min for BicD2 is enhanced by dimerization by a leucine zipper, similar to what was previously observed for binding of the cargo Egalitarian for Drosophila BicD (Sladewski et al., 2018; McClintock et al., 2018). Thus, it is conceivable that a Nup358-min dimer binds to a BicD2 dimer, which is also in line with our ITC results, which reveal a single KD. While Nup358-min is a monomer on its own, it oligomerizes to form 2:2 complexes with either the BicD2-CTD or the KLC2, and also forms a ternary complex with both BicD2-CTD and KLC2 with 2:2:2 stoichiometry (Cui et al., 2019; Noell et al., 2018). We thus propose that cargo adaptor-induced dimerization may potentially be a universal feature of the activation mechanism since BicD2 and KLC2 both form dimers in active dynein and kinesin-1 motors (McKenney et al., 2014; Urnavicius et al., 2015; Cui et al., 2019; Cockburn et al., 2018). Importantly, NMR titration showed that BicD2 binding has no effect on the NMR signal of the LEWD motif of Nup358 (Figure 3), which can interact directly with KLC2 (Cui et al., 2019). These data further suggest that the Nup358-min domain is potentially capable of recruiting both dynein and kinesin-1 machineries for bidirectional positioning of the nucleus, although this remains to be confirmed in the context of full-length proteins and intact motors. Intriguingly, our NMR data show that the BicD2-binding site is only separated by 30 residues from the LEWD motif that acts as KLC2-binding site. The proximity of these binding sites may play a role in coordinating motility for bidirectional transport (Fu and Holzbaur, 2014; Hendricks et al., 2010; Wilson and Holzbaur, 2012; Splinter et al., 2010; Soppina et al., 2009; Encalada et al., 2011; Zhang et al., 2007; Cui et al., 2019; Belyy et al., 2016; Feng et al., 2020; Roberts et al., 2014; Gross et al., 2003; Derr et al., 2012; Ally et al., 2009; Kendrick et al., 2019; Furuta et al., 2013). Another intriguing possibility is that if kinesin binding dimerizes Nup358, this may be the key initial step leading to BicD2 activation and recruitment of DD to form a bidirectional complex (Figure 9). The ternary Nup358/BicD2/KLC2 complex may be a model for other transport modules, in which opposite polarity motors such as dynein and kinesin-1 act together.
Conclusion
Based on our data, we propose a structural basis for cargo recognition through the dynein adaptor BicD2. Our results establish that BicD2 recognizes its cargo Nup358 through a small ‘cargo recognition α-helix’ that is embedded in an IDR. This region undergoes a structural transition from a random coil to an α-helix upon binding to BicD2. In single-molecule TIRF assays, four single-point mutations within the cargo recognition helix significantly inhibited the interaction between BicD2 and Nup358min-zip, and impaired run length and speed of the mutant DDBNmin-zip complexes on microtubules. Our results may facilitate the identification of regulatory mechanisms for BicD2-dependent transport pathways, which are important for cell cycle control, brain and muscle development, and vesicle transport.
Activation of BicD2 is a key regulatory step for transport as it is required to activate dynein for processive motility. The cargo recognition α-helix may be a structural feature that stabilizes BicD2 in its activated state. We propose that binding of cargo induces a coiled-coil registry shift in BicD2, which promotes loop opening and activates BicD2 for dynein/dynactin binding. Notably, Nup358 interacts more strongly with the dynein/dynactin/BicD2 complex compared to BicD2 alone, which supports the loop-opening mechanism for activation. We also show that BicD2 and KLC2 bind to spatially close, but nonoverlapping binding sites on Nup358, supporting the hypothesis that Nup358 is capable of simultaneously recruiting dynein and kinesin-1 machineries to the nucleus for bidirectional transport.
Materials and methods
Reagent type (species) or resource | Designation | Source or reference | Identifiers | Additional information |
---|---|---|---|---|
Strain, strain background (Escherichia coli) | Rosetta 2(DE3)-pLysS | Fisher Scientific | Cat# 714033 | |
Strain, strain background (E. coli) | BL21-DE3-CodonPlus-RIL | Fisher scientific | Cat#50-125-350 | |
Recombinant DNA reagent | Human BicD2-CTD pet28a | GenScript Reference Noell et al., 2019 doi:10.1021/acs.jpclett.9b01865 | Sequence encoding residues 715–804 of human BicD2 cloned into a pet28a vector via the NdeI and XhoI restriction sites | For the protein sequence expressed from this vector, see ‘Supplementary methods’ |
Recombinant DNA reagent | Human Nup358-min pGEX-6P-1 | GenScript Reference Noell et al., 2019doi:10.1021/acs.jpclett.9b01865 | Sequence encoding residues 2148–2240 of human Nup358 cloned into a pGEX-6P-1 vector via the BamHI and XhoI restriction sites | For the protein sequence expressed from this vector, see ‘Supplementary methods’ |
Recombinant DNA reagent | Nup358-min-pGEX-6P-1 | GenScriptThis paper | Modified Nup358-min pGEX-6P-1 vector that includes a SNAP-tag at its C-terminal domain | For the protein sequence expressed from this vector, see ‘Supplementary methods’This vector can be obtained from Dr. Solmaz’s lab |
Recombinant DNA reagent | Nup358-min-zip pGEX-6P-1 | GenScriptThis paper | Modified Nup358-min-pGEX-6P-1 vector that includes a leucine zipper that was added at the C-terminus before the snap-tag | For the protein sequence expressed from this vector, see ‘Supplementary methods’This vector can be obtained from Dr. Solmaz’s lab |
Recombinant DNA reagent | Human Nup358 (residues 2158–2199) pGEX-6P-1 | GenScriptThis paper | Sequence encoding residues 2158–2199 of human Nup358 cloned into a pGEX-6P-1 vector via the BamHI and XhoI restriction sites | Protein overexpression plasmid, which can be obtained from Dr. Solmaz’s lab |
Peptide, recombinant protein | PreScission protease | Cytiva | Cat# 27084301 | |
Peptide, Recombinant protein | Thrombin, human plasma | Fisher Scientific | Cat# 6051951000U | |
Chemical compound, drug | 13C D-Glucose (U-13C6) | Cambridge Isotope Laboratories | Cat# CLM-1396-PK | |
Chemical compound, drug | 15NH4Cl | Cambridge Isotope Laboratories | Cat# NLM-467-10 | |
Chemical compound, drug | cOmplete EDTA-free Protease Inhibitor Cocktail tablets | Roche | Cat# 45-5056489001-EA | |
Software, algorithm | Origin Student 2018 | OriginLab | CD spectroscopy | |
Software, algorithm | Origin GE Microcal ITC200 | OriginLab | ITC | |
Software, algorithm | BioXTAS RAW software suite (version 2.0.3). | Reference Hopkins et al., 2017 | ||
Software, algorithm | ImageJ 1.52v | Reference Schneider et al., 2012 | ||
Software, algorithm | UCSF Chimera (version 1.14) | Resource for Biocomputing, Visualization, and Informatics at the University of California, San FranciscoReference Pettersen et al., 2004 | ||
Chemical compound, drug | Coomassie brilliant blue R-250 | VWR | Cat# VWRV0472-25G | |
Chemical compound, drug | RNase Inhibitor | Promega | N261B | |
Chemical compound, drug | Q-dot 525 streptavidin conjugate | Invitrogen | Q10141MP | |
Chemical compound, drug | Q-dot 565 streptavidin conjugate | Invitrogen | Q10131MP | |
Chemical compound, drug | Q-dot 655 streptavidin conjugate | Invitrogen | Q10121MP | |
Chemical compound, drug | SNAP-Biotin | New England BioLabs | S9110S | |
Chemical compound, drug | Tubulin protein (X-rhodamine): bovine brain | Cytoskeleton, Inc | TL620M-A | |
Chemical compound, drug | Paclitaxel | Cytoskeleton, Inc | TXD01 | |
Recombinant DNA reagent | Bicaudal D homolog 2 isoform 2 (Homo sapiens) | This paper | NCBI:NP_056065.1 | Protein overexpression plasmid, which can be obtained from Dr. Solmaz’s lab |
Biological sample (Bos taurus) | Dynein-dynactin | Bovine brain | ||
Biological sample(B. taurus) | Tubulin | Bovine brain | ||
Software, algorithm | Nikon ECLIPSE Ti microscope | Nikon | ||
Software, algorithm | Nikon NIS Elements | Nikon | ||
Software, algorithm | Andor EMCCD Camera | Andor Technology USA | ||
Software, algorithm | Prism | GraphPad | v7; RRID:SCR_002798 | |
Chemical compound, drug | 2-[Methoxy(polyethyleneoxy)propyl]trimethoxysilane | J&K Scientific | 967192 | |
Chemical compound, drug | n-Butylamine | Acros Organics | A0344582 | |
Software, algorithm | ImageJ Fiji | NIH | 1.53c | |
Software, algorithm | NMRPipe | NIH, reference Delaglio et al., 1995 | ||
Software, algorithm | NMRFAM_SPARKY | Reference Lee et al., 2015 |
Protein expression and purification
Request a detailed protocolNup358-min and BicD2-CTD were expressed and purified as previously described (Noell et al., 2019; Noell et al., 2018; Cui et al., 2020). For details, see Appendix 3.
Pull-down assays
Request a detailed protocolGST-pull-down assays of human Nup358-min-GST and human BicD2-CTD were performed as described (Cui et al., 2020). For details, see Appendix 3.
Isothermal titration calorimetry
Request a detailed protocolITC experiments were performed as previously described (Noell et al., 2018). In brief, protein samples were extensively dialyzed against a buffer containing 150 mM NaCl, 30 mM HEPES pH 7.5, 0.5 mM TCEP, and 1 mM MgCl2. For ITC experiments, BicD2-CTD was placed in the cell of a calorimeter (MicroCal Auto-iTC200, GE Healthcare) and titrated with Nup358-min (either without the GST-tag Figure 1) or with the GST-tag intact (Figure 1—figure supplement 1a) at 25°C. As controls, titrations of Nup358-min (with or without the GST-tag) into buffer were carried out, which resulted in a flat line (Figure 1—figure supplement 1b and c). The corrected ITC curves were analyzed using a nonlinear least-squares minimization method in Origin 7.0 and fitted with the one-site model to determine the number of sites N, the equilibrium binding constant K, the change in entropy ∆S, and the change in enthalpy ∆H. The affinity (dissociation constant Kd) was calculated as the inverse of K. Data was analyzed with the Origin software (OriginLab). For the titration of Nup358-min + BicD2 CTD, the protein concentrations were 0.27 mM (Nup358-min) and 0.019 mM (BicD2-CTD), respectively. For the titration of Nup358-min-GST + BicD2 CTD, the protein concentrations were 0.12 mM (Nup358-min-GST) and 0.013 mM (BicD2-CTD), respectively. Protein concentrations were determined by UV absorbance at 280 nm.
Nuclear magnetic resonance
Request a detailed protocolHSQC NMR experiments of 15N-labeled Nup358-min were recorded on a 0.2 mM, 440 μl sample with 10% D2O on a Bruker 800 MHz spectrometer equipped with a cryoprobe at 25°C. HSQC was taken on a 1:1 mixture of 15N-Nup358min-BicD2-CTD, where the sample was concentrated to keep the concentration of Nup358-min to 0.2 mM in 20 mM HEPES pH 7.5, 150 mM NaCl, 0.5 mM TCEP. Backbone assignments were accomplished using standard triple resonance experiments and standard NMR processing analysis tools (Delaglio et al., 1995; Ying et al., 2017; Lee et al., 2009; Lee et al., 2019). For details, see Appendix 3. 15N CEST NMR was initially performed with a 0.6 mM sample and a 10:1 ratio of BicD2-CTD:15N-Nup358-min. The temperature was reduced to 20°C, and the pH was reduced to 6.5 to help improve signal/noise by decreasing the rate of solvent exchange of amide protons. 15N DCEST NMR (Yuwen et al., 2018a; Yuwen et al., 2018b) was performed with the same sample conditions. A second sample with a 20:1 ratio of 15N-Nup358-min:BicD2-CTD was utilized to improve the S/N ratio of the weakest peaks. For both experiments, the 800 MHz spectrometer with cryoprobe was used. For CEST, the saturation pulse was 400 ms at both 10 Hz and 20 Hz. The saturation frequency ranged from 118 ppm (the center of the HSQC spectrum) to 126 ppm in steps of 0.25 ppm in CEST. The second attempt was run from 116 to 124 ppm. The DCEST was performed to cover the whole spectral width for amides, with a 10 Hz saturation and a 700 Hz spectral width, and a 20 Hz saturation pulse with a 600 Hz spectral width. 13C′ HNCO-CEST NMR (Charlier et al., 2017) was performed with a 0.6 mM sample and a 1:20 ratio of BicD2-CTD:Nup358-Min at 20°C and pH of 6.5. For this experiment, a 600 MHz spectrometer with cryoprobe was used to minimize the effect of 13C′ transverse relaxation. The saturation pulse was 300 ms at 10 Hz. The saturation frequency ranged from 170 to 180 ppm in steps of 0.33 ppm. The CEST data were fitted with the program RING NMR Dynamics (Beckwith et al., 2020) and are presented in Appendix 1—table 1.
CD spectroscopy
Request a detailed protocolCD spectroscopy was performed as previously described (Cui et al., 2020). For details, see Appendix 3.
SAXS experiments
Request a detailed protocolNup358-min and BicD2-CTD were purified as described above. The monodispersity of the protein was confirmed by SEC-MALS, which is published (Cui et al., 2019; Noell et al., 2018). Purified Nup358-min and BicD2-CTD were dialyzed against the following buffer: 150 mM NaCl, 20 mM HEPES pH 7.5, 0.5 mM TCEP. The dialysis buffer was used as buffer match for SAXS experiments as well as for dilutions. The following protein concentrations were used: Nup358-min 4 mg/ml, BicD2-CTD 1 mg/ml. To assemble the complex, Nup358-min and BicD2-CTD were mixed in a 1:1 molar ratio and incubated for 30 min on ice, with a final concentration of 1.3 mg BicD2-CTD and 1.3 mg Nup358-min. The affinity of BicD2-CTD towards Nup358-min is 1.7 ± 0.9 μM (Figure 1); therefore, this protein concentration is sufficient for complex formation. To assure monodispersity, SAXS data were collected for at least three protein concentrations for each sample, and we also collected data of a Nup358-min/BicD2-CTD complex that was further purified by gel filtration, with comparable results (data not shown). Prior to data collection, samples were thawed, filtered (pore size 0.2 μm), and centrifuged (30 min, 21,700 × g, 4°C). SAXS data was collected at the beamline 7A1 at the Cornell High Energy Synchrotron Source, with a dual Pilatus 100k detector system (Dectris, Baden, Switzerland), at a single detector position, on July 3, 2019, as described previously (Zhao et al., 2021). Quartz capillary with a path length of 1.6 mm was used as the sample cell (OD = 1.5 mm, wall thickness = 10 μm). For each dataset, 20 frames were collected at 4°C, with 0.1 s exposure times (wavelength = 9.835 keV, beam dimensions = 250 * 250 μm, beam current = 49.9 mA [positrons], beam flux = 2.4 * 1012 photons/s). Most samples showed no detectable radiation damage, which was monitored by averaging 20 frames.
SAXS data were processed with the BioXTAS RAW software suite (version 2.0.3) (Hopkins et al., 2017). To obtain scattering intensity profiles, 20 data frames were reduced to scattering intensity profiles, placed on an absolute scale, averaged, and the scattering intensity profile of the buffer match was subtracted. The data quality was assessed by Guinier plots, molar mass calculations, and dimensionless Kratky plots in BioXTAS RAW (Hopkins et al., 2017; Hajizadeh et al., 2018; Konarev et al., 2003; Mylonas and Svergun, 2007; Trewhella et al., 2017). Pair distance distribution p(r) functions were derived from the scattering intensity profiles by the program GNOM (Svergun, 1992) of the ATSAS 3.0.0-1 software suite (Franke et al., 2017) implemented in RAW (Hopkins et al., 2017). Fifteen bead model 3D reconstructions were performed with the Dammiff program (Franke and Svergun, 2009) implemented in ATSAS/RAW (Hopkins et al., 2017; Franke et al., 2017). The resulting models were aligned, grouped into clusters, averaged, and the average model was refined in Dammiff (Franke and Svergun, 2009; Volkov and Svergun, 2003; Svergun, 1999). Figures of the refined molecular envelopes were created in the program UCSF Chimera (version 1.14)(Pettersen et al., 2004), developed by the Resource for Biocomputing, Visualization, and Informatics at the University of California, San Francisco, with support from P41-GM103311. P(r) functions were normalized to the highest signal of each curve.
Protein expression and purification for single-molecule assays
Request a detailed protocolCytoplasmic dynein and dynactin were purified from 300 g bovine brain as described in Bingham et al., 1998, and tubulin was purified from 200 g bovine brain as described in Castoldi and Popov, 2003. Purified dynein was stored at −20°C, and dynactin and tubulin were stored at –80°C, in 10 mM imidazole, pH 7.4, 0.2 M NaCl, 1 mM EGTA, 2 mM DTT, 10 μM Mg ATP, 5 μg/ml leupeptin, 50% glycerol. The N-terminal domain of human BicD2 (BicD2CC1) with a biotin tag at its N-terminus was expressed in bacteria as described (Sladewski et al., 2018). Full-length human wild-type BicD2 was expressed in Sf9 cells as described for Drosophila BicD (Sladewski et al., 2018). The Bradford reagent (Bio-Rad, USA) was used to measure the protein concentration. To create a fluorescently labeled version of Nup358-min, the expression vector described above was modified to include a SNAP-tag for fluorescent labeling at its CTD, which is referred to as Nup358min. Nup358min was expressed and purified with the N-terminal GST-tag intact as described for Nup358-min (Noell et al., 2018; Sckolnick et al., 2013) using the BL21-DE3-CodonPlus-RIL strain for expression. Nup358min was dimerized using a leucin zipper (hereafter called Nup358min-zip); the leucine zipper sequence was added at the C-terminus before the snap-tag. The sequences of these two constructs are shown in Appendix 3. The SNAP tag on Nup358min-zip and Nup358min was biotinylated with SNAP-biotin substrate (New England BioLabs, MA) as described (Sckolnick et al., 2013).
Single-molecule assay
Request a detailed protocolDynein, dynactin, BicD2, and Nu358min-zip constructs were diluted into high salt buffer (30 mM HEPES pH 7.4, 300 mM potassium acetate, 2 mM magnesium acetate, 1 mM EGTA, 20 mM DTT) and clarified for 20 min at 400,000 × g to remove aggregates. To form the dynein-dynactin-BicD2-Nup358min-zip (DDBNmin-zip) complex, BicD2 and Nup358min-zip were mixed with 525 nm and 655 nm streptavidin Qdots (Invitrogen, CA), respectively, at a 1:1 molar ratio in separate tubes and incubated for 15 min on ice. To block excess binding sites on streptavidin Qdots, 5 µM biotin was added to both tubes. Labeled BicD2 and Nup358min-zip were then mixed with preformed DD complex at a molar ratio of 1:1:2:2 (250 nM dynein, 250 nM dynactin, 500 nM BicD2, and 500 nM Nup358min-zip) and incubated on ice for 30 min in motility buffer (30 mM HEPES pH 7.4, 150 mM potassium acetate, 2 mM magnesium acetate, 1 mM EGTA, 20 mM DTT). The dynein-dynactin-Nup358min-zip (DDNmin-zip) complex contained Nup358min-zip that was labeled with a 655 nm Qdot. In the dynein-dynactin-BicD2CC1 (DDBCC1) complex, BicD2CC1 was labeled with a 525 nm streptavidin Qdot. The DDBNmin-zip, DDNmin-zip, and DDBCC1 complexes were diluted in motility buffer (30 mM HEPES pH 7.4, 150 mM potassium acetate, 2 mM magnesium acetate, 1 mM EGTA, 2 mM MgATP, 20 mM DTT, 8 mg/ml BSA, 0.5 mg/ml kappa-casein, 0.5% pluronic F68, 10 mM paclitaxel, and an oxygen scavenger system) to a final concentration of 1.25–2.50 nM dynein for observing motion on microtubules. The oxygen-scavenging system consisted of 5.8 mg/ml glucose, 0.045 mg/ml catalase, and 0.067 mg/ml glucose oxidase (Sigma-Aldrich). To analyze the motion of the complexes without any ambiguity, oxygen scavengers were not used in two-color experiments so that the microtubules would photo bleach. Purified tubulin was mixed with rhodamine-labeled tubulin at a molar ratio of 10:1 and polymerized as described (Sladewski et al., 2018). PEGylated glass slides were prepared and coated with 0.3 mg/ml rigor kinesin for microtubule attachment as described (Sladewski et al., 2018). After rinsing 2–3 times with motility buffer to remove excess rigor kinesin, MTs were added. Excess microtubules were removed by rinsing with motility buffer. Then, dynein protein samples were added to the glass surface. Motion of DDBNmin-zip, DDNmin-zip, and DDBCC1 were observed using TIRF microscope as described (Sladewski et al., 2018).
Microscopy and data analysis
Request a detailed protocolTo detect the motion of Qdot-labeled DDBNmin-zip and DDBCC1 complexes on microtubules, TIRF microscopy was used. The TIRF microscope system is operated by the Nikon NIS Elements software, and single-molecule images were acquired on a Nikon ECLIPSE Ti microscope equipped with objective-type TIRF. The laser lines 488 and 561 nm were used to illuminate 525 nm and 655 nm Qdots and rhodamine-labeled microtubules. Typically, 300–600 frames were captured at 100 or 200 ms intervals (10 or 5 frames/s) using two Andor EMCCD cameras (Andor Technology USA, South Windsor, CT). Individual Qdots were tracked using the ImageJ MtrackJ plugin for run length and speed measurements of single complexes (Meijering et al., 2012). Run length is defined as the total travel distance by individual complexes, and speed was calculated by dividing the run length by the total time. To determine the characteristic run length, data were plotted as 1-cumulative probability distribution (1-CDF) with GraphPad Prism Software and fit to a one-phase exponential decay equation p(x) = Ae-x/λ, where p(x) is the relative frequency, x is the travel distance along a microtubule track, and A is the amplitude. Speed was reported as mean ± SD, and run length reported as mean ± standard error (SE). The number of processive events was calculated by counting the number of moving dual-color Qdots per time per μm microtubule. The number of dual-color Qdots (DDBNmin-zip) was counted on 18–32 microtubules in each case. For measuring the formation of DDBNmin-zip and BNmin-zip complexes containing WT or mutant Nup358min-zip, the number of dual-color and single-color (green or red) Qdots was counted on multiple 512 × 512 pixel fields. The DDBNmin-zip and BNmin-zip complexes were bound to the glass surfaces nonspecifically. The percentage of colocalization was calculated from the total number of dual-color Qdots divided by all dual- and single-color Qdots. Statistical significance for two sets of run length data was determined by the Kolmogorov–Smirnov test, a nonparametric distribution. For speed data comparison, an unpaired t-test was performed. For three or more datasets of run length or speed, statistical significance was calculated using one-way ANOVA followed by Tukey’s post-hoc test. Statistical differences for binding frequency of DDBNmin-zip containing WT or mutant Nup358min-zip were determined by one-way ANOVA followed by Tukey’s post-hoc test.
Appendix 1
Summary of the 15N CEST Curve Fits.
(a) Summary of 15N CEST curve fits at 20:1 molar ratio of BicD2:15N-Nup358 | |||
Residue | kex (s–1) | Pb | Δδ (ppm) |
A2164 | 704 ± 211 | 0.07 ± 0.02 | –4.3 ± 0.4 |
K2165 | 139 ± 162 | 0.25 ± 0.08 | –0.8 ± 0.3 |
L2166 | 381 ± 182 | 0.07 ± 0.03 | –3.8 ± 0.3 |
I2167 | 975 ± 624 | 0.02 ± 0.04 | –5.7 ± 0.2 |
K2178 | 31 ± 35 | 0.01 ± 0.01 | –3.6 ± 0.2 |
L2184 | 160 ± 92 | 0.07 ± 0.03 | –5.5 ± 0.2 |
(b) Summary of 15N CEST curve fits at 10:1 molar ratio of BicD2:15N-Nup358 | |||
Residue | kex (s–1) | Pb | Δδ (ppm) |
A2163 | 12 ± 73 | 0.07 ± 0.05 | –3.6 ± 0.2 |
A2164 | 228 ± 72 | 0.09 ± 0.02 | –3.6 ± 0.2 |
K2165 | 757 ± 273 | 0.13 ± 0.07 | –3.6 ± 0.2 |
L2166 | 297 ± 195 | 0.05 ± 0.02 | –3.6 ± 0.2 |
-
The change in chemical shift in Table 1 was taken from Table S1b for the alanine residues due to a clearer minor peak. For the others, the change in chemical shift in Table 1 was taken from Table S1a. The values used in the weighted averages for kex and Pb were taken from Table S1a, excluding the K2165 and K2178 with abnormal Pb and significant noise in their CEST curves. Including the K2165 and K2178 values, the weighted average kex value would be 80 ± 30 s–1, and the Pb value would be 0.03 ± 0.01.
-
CEST: chemical exchange saturation transfer.
Summary of 13C′ CEST curve fits at 20:1 molar ratio of [BicD2]:[Nup358].
Residue | kex (s–1) | Pb | Δδ (ppm) | ||||||
---|---|---|---|---|---|---|---|---|---|
R2162 | 313 ± 328 | 0.05 ± 0.03 | 1.7 ± 0.3 | ||||||
A2164 | 474 ± 304 | 0.07 ± 0.03 | 2.8 ± 0.2 | ||||||
K2165 | 208 ± 179 | 0.05 ± 0.07 | 2.7 ± 0.1 | ||||||
L2166 | 391 ± 170 | 0.04 ± 0.03 | 2.6 ± 0.2 | ||||||
R2169 | 140 ± 125 | 0.06 ± 0.01 | 2.9 ± 0.2 | ||||||
E2171 | 359 ± 175 | 0.07 ± 0.03 | 2.2 ± 0.2 | ||||||
E2172 | 8 ± 98 | 0.22 ± 0.07 | 3.0 ± 0.1 | ||||||
L2177 | 540 ± 241 | 0.03 ± 0.02 | 0.9 ± 0.3 | ||||||
K2181 | 316 ± 155 | 0.06 ± 0.02 | 3.0 ± 0.1 | ||||||
F2183 | 464 ± 98 | 0.06 ± 0.01 | 3.3 ± 0.3 | ||||||
L2184 | 238 ± 165 | 0.08 ± 0.04 | 1.0 ± 0.2 | ||||||
Appendix 2
Summary of SAXS data.
Sample | RG (Å)Guinier plot | RG (Å) p(r) function | I(0) p(r) function/I(0) Guinier plot | TE /χ2p(r) function | DMax p(r) function | MW*† ‡(kDa) |
---|---|---|---|---|---|---|
Nup358/BicD2 | 48.1 ± 0.6 | 55.5 ± 0.2 | 0.086/0.086 | 0.61/1.04 | 190 | 47.7/46.0/47.6 |
Nup358-min | 28.4 ± 0.3 | 30.6 ± 0.4 | 0.035/0.036 | 0.69/1.05 | 120 | 12.7/12.3/na |
BicD2-CTD | 42.6 ± 1.0 | 49.7 ± 0.9 | 0.066/0.070 | 0.67/1.10 | 190 | na/na/38.7 |
-
Note that not all methods can be applied to all samples (na). The error of molar masses determined by SAXS is 10%. The calculated MWs are 10.9 kDa for BicD2-CTD and 10.6 kDa for Nup358-min.
-
RG: radius of gyration; I(0): scattering intensity at zero angle; TE: total estimate; DMAX: maximum particle diameter; MW: molar mass; SAXS: small-angle X-ray scattering.
-
*
MWs were determined from I(0), using glucose isomerase as a standard (Konarev et al., 2003).
-
†
MWs were determined from I(0) without a molar mass standard (Konarev et al., 2003).
-
‡
MWs were determined from the volume of correlation as described by Rambo and Tainer, 2013.
Statistics of SAXS bead model 3D reconstruction.
Sample | Mean normalized spatial discrepancy score (NSD) | χ2 (refined model)/χ2 (representative model) | Resolution (Å) | Ambimeter score | |
---|---|---|---|---|---|
Nup358-min/BicD2-CTD | 0.70 ± 0.05 | 1.08/1.05 | 41 ± 3 | 1.23 | |
Appendix 3
Supplementary methods
Sequences of expression constructs
Nup358min
MW 56.5kDa
MSPILGYWKIKGLVQPTRLLLEYLEEKYEEHLYERDEGDKWRNKKFELGLEFPNLPYYIDGDVKLTQSMAIIRYIADKHNMLGGCPKERAEISMLEGAVLDIRYGVSRIAYSKDFETLKVDFLSKLPEMLKMFEDRLCHKTYLNGDHVTHPDFMLYDALDVVLYMDPMCLDAFPKLVCFKKRIEAIPQIDKYLKSSKYIAWPLQGWQATFGGgDHPPKSDLEVLFQGPLGSDIPLQTPHKLVDTGRAAKLIQRAEEMKSGLKDFKTFLTNDQTKVTEEENKGSGTGAAGASDTTIKPNPENTGPTLEWDNYDLREDALDDSVSSMSGDKDCEMKRTTLDSPLGKLELSGCEQGLHRIIFLGKGTSAADAVEVPAPAAVLGGPEPLMQATAWLNAYFHQPEAIEEFPVPALHHPVFQQESFTRQVLWKLLKVVKFGEVISYSHLAALAGNPAATAAVKTALSGNPVPILIPCHRVVQGDLDVGGYEGGLAVKEWLLAHEGHRLGKPGLG
Nup358min-zip
MW 60.3 kDa
MSPILGYWKIKGLVQPTRLLLEYLEEKYEEHLYERDEGDKWRNKKFELGLEFPNLPYYIDGDVKLTQSMAIIRYIADKHNMLGGCPKERAEISMLEGAVLDIRYGVSRIAYSKDFETLKVDFLSKLPEMLKMFEDRLCHKTYLNGDHVTHPDFMLYDALDVVLYMDPMCLDAFPKLVCFKKRIEAIPQIDKYLKSSKYIAWPLQGWQATFGGgDHPPKSDLEVLFQGPLGSDIPLQTPHKLVDTGRAAKLIQRAEEMKSGLKDFKTFLTNDQTKVTEEENKGSGTGAAGASDTTIKPNPENTGPTLEWDNYDLREDALDDSVSSMKQLEDKVEELLSKNYHLENEVARLKKLVGERMSGDKDCEMKRTTLDSPLGKLELSGCEQGLHRIIFLGKGTSAADAVEVPAPAAVLGGPEPLMQATAWLNAYFHQPEAIEEFPVPALHHPVFQQESFTRQVLWKLLKVVKFGEVISYSHLAALAGNPAATAAVKTALSGNPVPILIPCHRVVQGDLDVGGYEGGLAVKEWLLAHEGHRLGKPGLG
Nup358-min
10.5 kDa
GPLGSDIPLQTPHKLVDTGRAAKLIQRAEEMKSGLKDFKTFLTNDQTKVTEEENKGSGTGAAGASDTTIKPNPENTGPTLEWDNYDLREDALDDSVSS
BicD2-CTD
10.9 kDa
GSHMYENEKAMVTETMMKLRNELKALKEDAATFSSLRAMFATRCDEYITQLDEMQRQLAAAEDEKKTLNSLLRMAIQQKLALTQRLELLELDHE
Protein expression and purification
Proteins were expressed and purified as previously described (Noell et al., 2019; Noell et al., 2018; Cui et al., 2020). Codon-optimized expression constructs and point mutants were created by a commercial cloning and gene synthesis service (GenScript). Sequences are listed in Appendix 3. For purification of isotope-labeled Nup358-min, the previously described expression vector for Nup358-min (which encoded the sequence for residues 2148–2240 of human Nup358 cloned into a pGEX6p1 vector) (Noell et al., 2019) was expressed in the Escherichia coli Rosetta 2(DE3)-pLysS strain as described (Noell et al., 2019), with the following modifications: M9 minimal media (3 g KH2PO4, 6.8 g Na2HPO4, 1 g NaCl, 4 g D-glucose, 1 g NH4Cl, 2 mM MgSO4, 0.1 mM CaCl2, 100 μg ampicillin, and 35 μg chloramphenicol per liter medium) were used for the expression, which was performed at 37°C. For expression of 15N-labeled Nup358-min, unlabeled NH4Cl was replaced in the medium by 1 g 15NH4Cl per liter. For expression of 13C and 15N-labeled Nup358-min, 1 g 13C D-glucose (U-13C6) and 1 g 15NH4Cl were used per 1 l of M9 media instead of the unlabeled compounds. Isotopes were obtained from Cambridge Isotope Laboratories.
Nup358-min was purified using the previously described protocol (Cui et al., 2019), but the cell pellet from a 6 l bacteria culture was dissolved in 75 ml of the lysis buffer to which 1 ½ tablets of cOmplete EDTA-free Protease Inhibitor Cocktail tablets were added (Roche). In short, Nup358-min was purified by glutathione affinity chromatography and eluted by proteolytic cleavage on the column with PreScission protease. The protein was further purified by size-exclusion chromatography as described (Cui et al., 2019), using the following buffer: 20 mM HEPES pH 7.5, 150 mM NaCl, 0.5 mM TCEP.
BicD2-CTD was expressed and purified as previously described (Noell et al., 2019; Cui et al., 2019; Noell et al., 2018). In short, BicD2-CTD was purified by Ni-NTA affinity chromatography, followed by proteolytic cleavage of the His6-tag by thrombin. BicD2-CTD was further purified by a second round of Ni-NTA affinity chromatography, followed by size-exclusion chromatography in the same buffer as described above. The Nup358 (residues 2158–2199)/BicD2-CTD complex was purified as described for the Nup358-min/BicD2-CTD complex (Noell et al., 2019).
Pull-down assays
GST-pull-down assays of human Nup358-min-GST and human BicD2-CTD were performed as described (Cui et al., 2020). For the assays, Nup358-min-GST was immobilized on glutathione sepharose beads and incubated with purified BicD2-CTD prior to elution with glutathione. The elution fractions were analyzed by SDS-PAGE (16% acrylamide gels) and stained with Coomassie blue. ImageJ was used for quantification of gel bands (Schneider et al., 2012).
Nuclear magnetic resonance
For backbone assignment, triple resonance experiments (HNCO, HNCA, HNCACO, HNCOCA, HNCACB, CBCCACONH, and HNN; Panchal et al., 2001) were performed on double-labeled 15N/13C Nup358-min at 0.4 mM on a Bruker 800 MHz spectrometer equipped with a cryoprobe. They were performed with nonuniform sampling (NUS). Processing of the data was performed with NMRPipe (Delaglio et al., 1995) and SMILE (Ying et al., 2017). Further analysis of the data was performed with NMRFAM_SPARKY (Lee et al., 2015), including iPine (Lee et al., 2009; Lee et al., 2019).
CD spectroscopy
Purified proteins at a concentration of 0.3 mg/ml were dialyzed in the following buffer: 150 mM NaCl, 10 mM Tris pH 8, and 0.2 mM TCEP. Data were recorded with a Jasco J-1100 CD Spectrometer, equipped with a thermoelectric control device. A quartz cuvette with a path length of 0.1 cm was used. After the buffer baseline subtraction, CD signals were normalized to the protein concentration and converted to mean residue molar ellipticity [Θ]. Thus, the ellipticity Θ (mdeg) was multiplied with the conversion factor 391.51 cm2/dmol for all spectra with the exception of the Nup358-min spectrum, for which the conversion factor was 362.00 cm2/dmol. For the Nup358 + BicD2 spectrum, the spectra of Nup358-min and BicD2-CTD were added together prior to conversion to [Θ].
Data availability
Protein backbone assignments have been deposited in the BMRB under accession code 5182. All other data generated or analyzed during this study are included in the manuscript and supporting files; Source Data files have been provided for Figures 1, 2, 3, 4, 5, 6, 7, and 8.
-
Biological Magnetic Resonance Data BankID 51282. NMR Backbone Assignment of Nup358-Min.
References
-
Opposite-polarity motors activate one another to trigger cargo transport in live cellsThe Journal of Cell Biology 187:1071–1082.https://doi.org/10.1083/jcb.200908075
-
RING NMR dynamics: software for analysis of multiple NMR relaxation experimentsJournal of Biomolecular NMR 10:350.https://doi.org/10.1007/s10858-020-00350-w
-
The mammalian dynein-dynactin complex is a strong opponent to kinesin in a tug-of-war competitionNature Cell Biology 18:1018–1024.https://doi.org/10.1038/ncb3393
-
Biophysical analysis of the interaction of Rab6a GTPase with its effector domainsThe Journal of Biological Chemistry 284:2628–2635.https://doi.org/10.1074/jbc.M806003200
-
PredictProtein - Predicting Protein Structure and Function for 29 YearsNucleic Acids Research 49:W535–W540.https://doi.org/10.1093/nar/gkab354
-
Purification of dynactin and dynein from brain tissueMethods in Enzymology 298:171–184.https://doi.org/10.1016/s0076-6879(98)98017-x
-
Two binding partners cooperate to activate the molecular motor Kinesin-1The Journal of Cell Biology 176:11–17.https://doi.org/10.1083/jcb.200605099
-
A Nup133-dependent NPC-anchored network tethers centrosomes to the nuclear envelope in prophaseThe Journal of Cell Biology 192:855–871.https://doi.org/10.1083/jcb.201007118
-
The docking of kinesins, KIF5B and KIF5C, to Ran-binding protein 2 (RanBP2) is mediated via a novel RanBP2 domainThe Journal of Biological Chemistry 276:41594–41602.https://doi.org/10.1074/jbc.M104514200
-
Purification of brain tubulin through two cycles of polymerization-depolymerization in a high-molarity bufferProtein Expression and Purification 32:83–88.https://doi.org/10.1016/S1046-5928(03)00218-3
-
Structure and Dynamics of an Intrinsically Disordered Protein Region That Partially Folds upon Binding by Chemical-Exchange NMRJournal of the American Chemical Society 139:12219–12227.https://doi.org/10.1021/jacs.7b05823
-
Insights into Kinesin-1 Activation from the Crystal Structure of KLC2 Bound to JIP3Structure (London, England 26:1486–1498.https://doi.org/10.1016/j.str.2018.07.011
-
Coiled-coil registry shifts in the F684I mutant of Bicaudal D result in cargo-independent activation of dynein motilityTraffic (Copenhagen, Denmark) 21:463–478.https://doi.org/10.1111/tra.12734
-
NMRPipe: A multidimensional spectral processing system based on UNIX pipesJournal of Biomolecular NMR 6:277–293.https://doi.org/10.1007/BF00197809
-
Tug-of-war in motor protein ensembles revealed with a programmable DNA origami scaffoldScience (New York, N.Y.) 338:662–665.https://doi.org/10.1126/science.1226734
-
Role of Microtubules and Microtubule-Associated Proteins in HIV-1 InfectionJournal of Virology 92:e00085-18.https://doi.org/10.1128/JVI.00085-18
-
Dynactin p150 promotes processive motility of DDB complexes by minimizing diffusional behavior of dyneinMolecular Biology of the Cell 31:782–792.https://doi.org/10.1091/mbc.E19-09-0495
-
DAMMIF, a program for rapid ab-initio shape determination in small-angle scatteringJournal of Applied Crystallography 42:342–346.https://doi.org/10.1107/S0021889809000338
-
ATSAS 2.8: a comprehensive data analysis suite for small-angle scattering from macromolecular solutionsJournal of Applied Crystallography 50:1212–1225.https://doi.org/10.1107/S1600576717007786
-
Integrated regulation of motor-driven organelle transport by scaffolding proteinsTrends in Cell Biology 24:564–574.https://doi.org/10.1016/j.tcb.2014.05.002
-
The structure of human neuronal Rab6B in the active and inactive formActa Crystallographica. Section D, Biological Crystallography 62:725–733.https://doi.org/10.1107/S0907444906015319
-
Rab6 regulates transport and targeting of exocytotic carriersDevelopmental Cell 13:305–314.https://doi.org/10.1016/j.devcel.2007.06.010
-
An efficient experiment for sequential backbone assignment of medium-sized isotopically enriched proteinsJournal of Magnetic Resonance 99:201–207.https://doi.org/10.1016/0022-2364(92)90169-8
-
Correlating backbone amide and side chain resonances in larger proteins by multiple relayed triple resonance NMRJournal of the American Chemical Society 114:6291–6293.https://doi.org/10.1021/ja00042a003
-
BioXTAS RAW: improvements to a free open-source program for small-angle X-ray scattering data reduction and analysisJournal of Applied Crystallography 50:1545–1553.https://doi.org/10.1107/S1600576717011438
-
Disease-associated mutations in human BICD2 hyperactivate motility of dynein-dynactinThe Journal of Cell Biology 216:3051–3060.https://doi.org/10.1083/jcb.201703201
-
Three-dimensional triple-resonance NMR spectroscopy of isotopically enriched proteinsJournal of Magnetic Resonance 89:496–514.https://doi.org/10.1016/0022-2364(90)90333-5
-
Hook3 is a scaffold for the opposite-polarity microtubule-based motors cytoplasmic dynein-1 and KIF1CThe Journal of Cell Biology 218:2982–3001.https://doi.org/10.1083/jcb.201812170
-
PRIMUS: a Windows PC-based system for small-angle scattering data analysisJournal of Applied Crystallography 36:1277–1282.https://doi.org/10.1107/S0021889803012779
-
PINE-SPARKY: graphical interface for evaluating automated probabilistic peak assignments in protein NMR spectroscopyBIOINFORMATICS (Oxford, England) 25:2085–2087.https://doi.org/10.1093/bioinformatics/btp345
-
NMRFAM-SPARKY: enhanced software for biomolecular NMR spectroscopyBioinformatics (Oxford, England) 31:1325–1327.https://doi.org/10.1093/bioinformatics/btu830
-
I-PINE web server: an integrative probabilistic NMR assignment system for proteinsJournal of Biomolecular NMR 73:213–222.https://doi.org/10.1007/s10858-019-00255-3
-
Activation of cytoplasmic dynein motility by dynactin-cargo adapter complexesScience (New York, N.Y.) 345:337–341.https://doi.org/10.1126/science.1254198
-
Methods for cell and particle trackingMethods in Enzymology 504:183–200.https://doi.org/10.1016/B978-0-12-391857-4.00009-4
-
The regulation of bidirectional mitochondrial transport is coordinated with axonal outgrowthJournal of Cell Science 104 (Pt 3):917–927.https://doi.org/10.1242/jcs.104.3.917
-
Accuracy of molecular mass determination of proteins in solution by small-angle X-ray scatteringJournal of Applied Crystallography 40:s245–s249.https://doi.org/10.1107/S002188980700252X
-
Mutations in BICD2, which encodes a golgin and important motor adaptor, cause congenital autosomal-dominant spinal muscular atrophyAmerican Journal of Human Genetics 92:946–954.https://doi.org/10.1016/j.ajhg.2013.04.011
-
A Quantitative Model for BicD2/Cargo InteractionsBiochemistry 57:6538–6550.https://doi.org/10.1021/acs.biochem.8b00987
-
Role of Coiled-Coil Registry Shifts in the Activation of Human Bicaudal D2 for Dynein Recruitment upon Cargo BindingThe Journal of Physical Chemistry Letters 10:4362–4367.https://doi.org/10.1021/acs.jpclett.9b01865
-
Molecular defects in the motor adaptor BICD2 cause proximal spinal muscular atrophy with autosomal-dominant inheritanceAmerican Journal of Human Genetics 92:955–964.https://doi.org/10.1016/j.ajhg.2013.04.013
-
Structural basis for kinesin-1:cargo recognitionScience (New York, N.Y.) 340:356–359.https://doi.org/10.1126/science.1234264
-
UCSF Chimera--A visualization system for exploratory research and analysisJournal of Computational Chemistry 25:1605–1612.https://doi.org/10.1002/jcc.20084
-
The cytoplasmic dynein transport machinery and its many cargoesNature Reviews. Molecular Cell Biology 19:382–398.https://doi.org/10.1038/s41580-018-0004-3
-
SKIP controls lysosome positioning using a composite kinesin-1 heavy and light chain-binding domainJournal of Cell Science 130:1637–1651.https://doi.org/10.1242/jcs.198267
-
NIH Image to ImageJ: 25 years of image analysisNature Methods 9:671–675.https://doi.org/10.1038/nmeth.2089
-
More than just a cargo adapter, melanophilin prolongs and slows processive runs of myosin VaThe Journal of Biological Chemistry 288:29313–29322.https://doi.org/10.1074/jbc.M113.476929
-
BICD2, dynactin, and LIS1 cooperate in regulating dynein recruitment to cellular structuresMolecular Biology of the Cell 23:4226–4241.https://doi.org/10.1091/mbc.E12-03-0210
-
Determination of the regularization parameter in indirect-transform methods using perceptual criteriaJournal of Applied Crystallography 25:495–503.https://doi.org/10.1107/S0021889892001663
-
Motor ProteinsCold Spring Harbor Perspectives in Biology 10:a021931.https://doi.org/10.1101/cshperspect.a021931
-
Dominant spinal muscular atrophy due to BICD2: A novel mutation refines the phenotypeJournal of Neurology, Neurosurgery, and Psychiatry 85:590–592.https://doi.org/10.1136/jnnp-2013-306777
-
Structural basis for cargo binding and autoinhibition of Bicaudal-D1 by a parallel coiled-coil with homotypic registryBiochemical and Biophysical Research Communications 460:451–456.https://doi.org/10.1016/j.bbrc.2015.03.054
-
2017 publication guidelines for structural modelling of small-angle scattering data from biomolecules in solution: an updateActa Crystallographica. Section D, Structural Biology 73:710–728.https://doi.org/10.1107/S2059798317011597
-
The structure of the dynactin complex and its interaction with dyneinScience (New York, N.Y.) 347:1441–1446.https://doi.org/10.1126/science.aaa4080
-
Studying “invisible” excited protein states in slow exchange with a major state conformationJournal of the American Chemical Society 134:8148–8161.https://doi.org/10.1021/ja3001419
-
Uniqueness of ab initio shape determination in small-angle scatteringJournal of Applied Crystallography 36:860–864.https://doi.org/10.1107/S0021889803000268
-
Length Dependent Helix−Coil Transition Kinetics of Nine Alanine-Based PeptidesThe Journal of Physical Chemistry B 108:15301–15310.https://doi.org/10.1021/jp037272j
-
Prediction and functional analysis of native disorder in proteins from the three kingdoms of lifeJournal of Molecular Biology 337:635–645.https://doi.org/10.1016/j.jmb.2004.02.002
-
Opposing microtubule motors drive robust nuclear dynamics in developing muscle cellsJournal of Cell Science 125:4158–4169.https://doi.org/10.1242/jcs.108688
-
Interpreting Protein Chemical Shift DataProgress in Nuclear Magnetic Resonance Spectroscopy 58:62–87.https://doi.org/10.1016/j.pnmrs.2010.07.004
-
PredictProtein--an open resource for online prediction of protein structural and functional featuresNucleic Acids Research 42:W337–W343.https://doi.org/10.1093/nar/gku366
-
Exploring methods to expedite the recording of CEST datasets using selective pulse excitationJournal of Magnetic Resonance (San Diego, Calif) 292:1–7.https://doi.org/10.1016/j.jmr.2018.04.013
-
Dramatic Decrease in CEST Measurement Times Using Multi-Site ExcitationChemphyschem: A European Journal of Chemical Physics and Physical Chemistry 19:1707–1710.https://doi.org/10.1002/cphc.201800249
Article and author information
Author details
Funding
National Institute of General Medical Sciences (R01 GM144578)
- M Yusuf Ali
- Sozanne R Solmaz
- Chunyu Wang
National Cancer Institute (CA206592)
- Chunyu Wang
National Institute on Aging (AG069039)
- Chunyu Wang
National Institute of General Medical Sciences (R15 GM128119)
- Sozanne R Solmaz
Chemistry Department and the Research Foundation of SUNY
- Sozanne R Solmaz
National Institute of General Medical Sciences (R35 GM136288)
- Kathleen M Trybus
National Institute of Neurological Disorders and Stroke (R03 NS114115)
- M Yusuf Ali
The funders had no role in study design, data collection and interpretation, or the decision to submit the work for publication.
Acknowledgements
We thank Fabien Ferrage, Guillaume Bouvignies, and Philippe Pelupessy for help with the CEST experiments and Bruce Johnson for help with fitting of the CEST data. We thank Michael Cosgrove for helpful discussions regarding SAXS. SRS, CW, and MYA were funded by NIH grant R01 GM144578. CW was funded by NIH grants CA206592 and AG069039. SRS was funded by NIH grant R15 GM128119 and additional funds came from the Chemistry Department and the Research Foundation of SUNY. KMT was funded by NIH grant R35 GM136288. MYA was funded by NIH grant R03 NS114115. The CD instrument was supported by NIGMS grants 1R01GM125853-02S1 and 3R35GM130207-01S1. SAXS data was collected at beamline 7A1, Cornell High Energy Synchrotron Source, supported by NSF award DMR-1829070, and by NIH/NIGMS award GM-124166. We thank Qingqiu Huang and Richard Gillilan for user support at the synchrotron source. We also thank Patty Fagnant, Carol Bookwalter, and Elena Krementsova for cloning, protein expression, and protein purification. We thank the High-Throughput and Spectroscopy Resource Center at Rockefeller University for support of the ITC experiments. The authors declare that they have no conflicting interests.
Copyright
© 2022, Gibson et al.
This article is distributed under the terms of the Creative Commons Attribution License, which permits unrestricted use and redistribution provided that the original author and source are credited.
Metrics
-
- 2,055
- views
-
- 296
- downloads
-
- 22
- citations
Views, downloads and citations are aggregated across all versions of this paper published by eLife.
Citations by DOI
-
- 22
- citations for umbrella DOI https://doi.org/10.7554/eLife.74714