Opposing chemosensory functions of closely related gustatory receptors
eLife assessment
This valuable study focuses on the role of the Gr28 family of insect chemoreceptors. Using the Drosophila larva, the authors show that taste neurons expressing different members of this family of bitter taste receptors trigger opposite behavior – attraction and repulsion. They establish the minimal bitter taste receptor subunit composition needed in these neurons to mediate the repulsion of bitter tastants. The evidence presented is convincing, using well-validated and controlled tools and experiments.
https://doi.org/10.7554/eLife.89795.3.sa0Valuable: Findings that have theoretical or practical implications for a subfield
- Landmark
- Fundamental
- Important
- Valuable
- Useful
Convincing: Appropriate and validated methodology in line with current state-of-the-art
- Exceptional
- Compelling
- Convincing
- Solid
- Incomplete
- Inadequate
During the peer-review process the editor and reviewers write an eLife Assessment that summarises the significance of the findings reported in the article (on a scale ranging from landmark to useful) and the strength of the evidence (on a scale ranging from exceptional to inadequate). Learn more about eLife Assessments
Abstract
In the fruit fly Drosophila melanogaster, gustatory sensory neurons express taste receptors that are tuned to distinct groups of chemicals, thereby activating neural ensembles that elicit either feeding or avoidance behavior. Members of a family of ligand -gated receptor channels, the Gustatory receptors (Grs), play a central role in these behaviors. In general, closely related, evolutionarily conserved Gr proteins are co-expressed in the same type of taste neurons, tuned to chemically related compounds, and therefore triggering the same behavioral response. Here, we report that members of the Gr28 subfamily are expressed in largely non-overlapping sets of taste neurons in Drosophila larvae, detect chemicals of different valence, and trigger opposing feeding behaviors. We determined the intrinsic properties of Gr28 neurons by expressing the mammalian Vanilloid Receptor 1 (VR1), which is activated by capsaicin, a chemical to which wild-type Drosophila larvae do not respond. When VR1 is expressed in Gr28a neurons, larvae become attracted to capsaicin, consistent with reports showing that Gr28a itself encodes a receptor for nutritious RNA. In contrast, expression of VR1 in two pairs of Gr28b.c neurons triggers avoidance to capsaicin. Moreover, neuronal inactivation experiments show that the Gr28b.c neurons are necessary for avoidance of several bitter compounds. Lastly, behavioral experiments of Gr28 deficient larvae and live Ca2+ imaging studies of Gr28b.c neurons revealed that denatonium benzoate, a synthetic bitter compound that shares structural similarities with natural bitter chemicals, is a ligand for a receptor complex containing a Gr28b.c or Gr28b.a subunit. Thus, the Gr28 proteins, which have been evolutionarily conserved over 260 million years in insects, represent the first taste receptor subfamily in which specific members mediate behavior with opposite valence.
Introduction
Meaningful animal behavior is established through cooperative engagement of multiple sensory systems. In many insects, the chemosensory system plays a central role in such integration processes. The fruit fly Drosophila melanogaster has served as the primary insect model system for elucidating the molecular basis and neural circuitry of both olfaction and taste, by virtue of the vast genetic resources, amenability to both neurophysiological recording and live imaging, and simple yet powerful behavioral assays, allowing investigators to link genes to chemosensory behavior and neural activity (Montell, 2021).
The Drosophila gustatory system is characterized by several insect-specific anatomical and molecular features. For example, taste cells are primary sensory neurons, with dendritic processes that express taste receptors, while long axons project and convey taste information directly to the brain. In adult flies, these neurons, referred to as Gustatory Receptor Neurons (GRNs), are distributed across several appendages, such as labial palps, legs, and presumably the antennae. Additionally, some GRNs reside internally, arranged in cell clusters along the pharynx (Amrein, 2016; Joseph and Carlson, 2015; Scott, 2018). Likewise, Drosophila larvae have numerous structures located both on the head surface and internally along the larval pharynx, where chemical compounds are assessed during their passage toward the digestive system (Figure 1A; Apostolopoulou et al., 2015; Kwon et al., 2011; Rist and Thum, 2017).
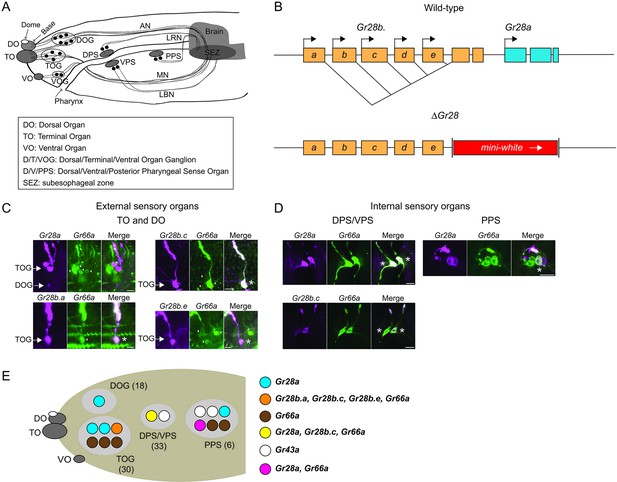
Expression of the Gr28 genes in the larval sensory organs.
(A) Schematic representation of the larval chemosensory system. Three external sensory organs (dorsal organ [DO], terminal organ [TO], and ventral organ [VO]) hold collectively the dendritic extensions of neuronal cell bodies in the respective ganglia (dorsal organ ganglia [DOG], terminal organ ganglia [TOG], and ventral organ ganglia [VOG]). Three clusters of sensory neurons (dorsal pharyngeal sense organ [DPS], ventral pharyngeal sense organ [VPS], and posterior pharyngeal sense organ [PPS]) reside along the pharynx. The antennal nerve (AN) connects the DOG neurons to the subesophageal zone (SEZ). The TOG and VOG neurons project along the maxillary nerve (MN) to the SEZ. The DPS and PPS neurons project axons to the SEZ via the labral nerve (LRN). The VPS neurons project to the SEZ through the labial nerve (LBN). Note that olfactory neurons are omitted in the schematic here and in (E) (below). (B) Structure of the Gr28 locus. The six Gr28 genes are clustered within 10 kilobases. The five Gr28b genes are transcribed from distinct promotors, with first unique exons that are spliced to common second and third exons. Gr28a is downstream of the Gr28b genes. The Gr28 mutation (ΔGr28) used in this study lacks the shared common exons of the Gr28b genes and the entire Gr28a gene. (C) Expression of the Gr28 genes in the external sensory organs. Note that images show only one of the bilaterally symmetrical organs. Co-expression between Gr66a (a marker for bitter taste gustatory receptor neurons [GRNs]) and different Gr28 genes (in ‘Merge’ panel) was assessed using GAL4 and LexA drivers for Gr28 genes and Gr66a, respectively. For Gr28b.a, Gr28b.c and Gr28b.e co-expression was observed in each case. However, Gr66a and Gr28a are expressed in different GRNs. Asterisks refer to a GRN expressing both Gr66a and the indicated Gr28 gene. Scale bars are 5 μm. (D) Expression of the Gr28 genes in internal sensory organs. Note that the bilaterally symmetrical halves of the DPS/VPS are physically close to each other, and the images includes both halves, while the image of the PPS shows only one side of the bilaterally symmetrical organ. Gr28b.c, but none of the other Gr28b genes, is co-expressed with Gr66a in the DPS/VPS. In the PPS, none of the Gr28b genes is found, while Gr28a and Gr66a are partially co-expressed, but each gene is also expressed exclusively in a subset of GRNs. Asterisks refer to a GRN expressing both Gr66a and the indicated Gr28 gene. Scale bars are 5 μm. (E) Expression summary: only relevant neurons in one of the paired taste organs are shown, with total number of neurons indicated in parenthesis. The cartoon summarizes the immunostainings shown in (C) and (D). The VOG is not shown as none of the Gr28-GAL4 drivers is expressed there. Immunostaining was performed on whole-mount preparations from larvae heads of the following genotypes: UAS-mCD8:RFP lexAop-rCD2:GFP;Gr66a-LexA/Gr28a-GAL4, UAS-mCD8:RFP lexAop-rCD2:GFP;Gr66a-LexA/Gr28b.c-GAL4, UAS-mCD8:RFP lexAop-rCD2:GFP;Gr66a-LexA/Gr28b.e-GAL4 and UAS-mCD8:RFP lexAop-rCD2:GFP;Gr66a-LexA/+; Gr28b.a-GAL4/+. The Gr43aGAL4 GRNs, which do not overlap with Gr28a-GAL4 neurons (Mishra et al., 2018), are shown for reference to experiments described in (Figure 2).
Fruit flies, like most insects, employ taste receptors encoded by two major gene families, the Gustatory receptor (Gr) and the Ionotropic Receptor (IR) genes, to sense soluble chemicals, such as appetitive food compounds, noxious and toxic chemicals, as well as pheromones. Both Gr- and IR-based receptors are thought to form complexes composed of several, and generally different, subunits. Composition of only a few Gr-based taste receptors for sugars and bitter compounds is known (Jiao et al., 2008; Yavuz et al., 2014; Shim et al., 2015; Sung et al., 2017; Fujii et al., unpublished), and two Gr proteins, Gr21a and Gr63a, are co-expressed in a small subset of olfactory neurons where they form a receptor complex for gaseous carbon dioxide (Jones et al., 2007; Kwon et al., 2007).
The Gr genes represent the largest taste receptor gene family in insects. In D. melanogaster, it is comprised of 60 genes predicted to encode 68 proteins, expression of which has been extensively described (Dahanukar et al., 2007; Dunipace et al., 2001; Fujii et al., 2015; Scott et al., 2001; Weiss et al., 2011). Several Gr genes have been functionally characterized in adult flies using genetic mutations combined with either electrophysiological recordings, Ca2+ imaging studies, or behavioral analyses (Amrein, 2016; Montell, 2021), but only a few have been studied in larvae (Apostolopoulou et al., 2016; Choi et al., 2016; Choi et al., 2020; Mishra et al., 2013; Mishra et al., 2018).
The Gr28 gene subfamily is of particular interest for a number of reasons: first, it is one of the most conserved Gr subfamilies, homologs of which can be found across all insect families and even more distant arthropods (Eyun et al., 2017; Fujii et al., 2023; Suzuki et al., 2018). The six Gr28 genes are tightly clustered, with the five Gr28b genes transcribed from distinct promotors and unique exons spliced to shared second and third exons, while Gr28a is a separate transcription unit (Figure 1B). Overall conservation between the Gr28 proteins is high (≥50% similarity), characteristic for genes generated through recent gene duplication events. Second, the Gr28 genes are expressed not only in the gustatory system of larvae and adult flies, but also in many other organs, especially the central nervous system and non-chemosensory neurons of the peripheral nervous system, suggesting that they have functions beyond gustation and are important to sense chemical signals unrelated to food (Mishra et al., 2018; Thorne and Amrein, 2008). Notably, evidence for such roles has been reported before any direct link to gustatory perception was discovered. Ni and colleagues showed that Gr28b.d is essential for high-temperature avoidance in flies (Ni et al., 2013), while Xiang and collaborators found that Gr28 mutant larvae were deficient in UV light avoidance (Xiang et al., 2010). Third, the only known gustatory function for any Gr28 protein thus far is sensing of RNA and ribose by Gr28a, mediated by Gr28a-GAL4 GRNs (Mishra et al., 2018). Remarkably, sensing RNA and ribose is an appetitive taste quality found in other dipteran insects that diverged from Drosophila about 260 million years ago, including flesh flies and mosquitoes, and we showed that Gr28 homologs from Aedes aegypti and Anopheles gambiae can restore RNA and ribose preference in Drosophila Gr28 mutant larvae when expressed in Gr28a-GAL4 GRNs (Fujii et al., 2023).
Here, we present a detailed expression analysis and functional characterization of the Gr28 genes and the respective GRNs in Drosophila larvae. In addition to Gr28a, three of the five Gr28b genes (Gr28b.a, Gr28b.c, and Gr28b.e) are also expressed in the larval taste system. Interestingly, GRNs expressing Gr28a and the three Gr28b genes represent functionally distinct neuronal ensembles, with minimal expression overlap in a single pair of neurons expressing both Gr28a and Gr28b.c. When the mammalian Vanilloid Receptor 1 (VR1) is expressed under the control of specific GAL4 drivers, we found that Gr28a-GAL4 and Gr28b.c-GAL4 neurons mediate opposing taste behavior in the presence of capsaicin, the ligand for VR1. Specifically, Gr28a-GAL4/UAS-VR1 larvae show strong attraction for capsaicin, while Gr28b.c-GAL4/UAS-VR1 larvae show strong avoidance of capsaicin. Neuronal inactivation experiments reveal that the Gr28b.c GRNs are necessary to sense bitter compounds, such as denatonium, quinine, lobeline, and caffeine. Moreover, Ca2+ responses of Gr28b.c GRNs to denatonium and quinine are significantly reduced and avoidance behavior of these two chemicals is diminished in Gr28 mutant larvae, whereas Ca2+ responses and avoidance behavior were not affected when challenged with lobeline and caffeine. This implies that Gr28b proteins are subunits of receptor complexes sensing a subgroup of bitter tasting compounds. In summary, the Gr28 genes encode related Gr proteins mediating both positive and negative valence.
Results
Expression of the Gr28 genes in the larval taste organs
The peripheral chemosensory system of the larvae is subdivided into bilaterally symmetrical, ‘external’ and ‘internal’ taste organs (Stocker, 2008). The three external organs reside near the tip of the head and are organized as paired ganglia, the dorsal, terminal, and ventral organ ganglia (DOG, TOG, and VOG) that house the GRN cell bodies with dendritic extensions in respective sensory organs (DO, TO, and VO) at the head surface, while carrying information via their axons to the subesophageal zone (SEZ) in the brain (Figure 1A). The DOG harbors 21 olfactory neurons and 18 presumptive GRNs. For clarity, GRN numbers refer to neurons in one of the two, bilaterally symmetrical taste organs. The GRNs located in the DOG fall into two distinct groups, based on their dendritic extensions: 11 presumptive GRNs, 4 of which were shown to express Gr genes (Apostolopoulou et al., 2015), send dendrites to the base of the DO, while 7 neurons extend dendrites to the TO (the dorsolateral group, Figure 1A; Kwon et al., 2011). The TOG contains 30 neurons, with dendrites located in the TO (the distal group). The internal taste structures, referred to as the dorsal/ventral pharyngeal sense organ (DPS/VPS, 33 neurons) and the posterior pharyngeal sense organs (PPS, 6 neurons) are located along the pharynx and sense chemicals as they are moved toward the digestive system. We note that not all these neurons are confirmed GRNs, either by function or expression of Gr or IR genes, albeit based on their location and anatomy, most are thought to be GRNs (Rist and Thum, 2017; Sánchez-Alcañiz et al., 2018; Stewart et al., 2015).
Our initial expression analysis of the Gr28 genes revealed that four of the six Gr28 genes (Gr28a, Gr28b.a, Gr28b.c, and Gr28b.e) were expressed in larval taste organs, in addition to cells in the gut, the brain, and non-chemosensory cells of the larvae (Mishra et al., 2018; Thorne and Amrein, 2008). This and all previous Gr expression studies were performed using bimodal expression systems, mostly GAL4/UAS, whereby Gr promotors driving GAL4 are assumed to faithfully reproduce expression of the respective Gr genes. Importantly, we analyzed two to four Gr28-GAL4 insertion lines for each transgene, and at least two generated the same expression pattern (Mishra et al., 2018; Thorne and Amrein, 2008), providing evidence that the drivers reflect a fairly accurate expression profile of respective endogenous genes. To further delineate the putative chemosensory roles of these genes, we performed a more detailed co-expression analysis between the Gr28 genes and the bitter taste receptor gene Gr66a by combining the GAL4/UAS (labeling Gr28 expressing neurons) with the LexA/lexAop (marking Gr66a neurons) system (Figure 1C and D). In the TOG, we found expression of all four Gr28 genes, along with that of Gr66a, which was expressed in three or four neurons (this number is slightly smaller than the six neurons previously reported by Kwon et al., 2011). GAL4 drivers for Gr28b.a, Gr28b.c, and Gr28b.e are co-expressed in a single neuron with Gr66a-LexA (Figure 1C). In contrast, Gr28a-GAL4 is expressed in a distinct TOG neuron than Gr66a-LexA (Figure 1C) and the Gr28b genes, an observation we independently confirmed using a Gr28b.c-LexA driver (Figure 1—figure supplement 1). In the DOG, we find only a single Gr28a-GAL4 neuron, while none of the Gr28b genes, or Gr66a, is expressed there (Figure 1C). None of the Gr28-GAL4 drivers was expressed in the VOG. In the internal sensory organs, Gr66a-LexA is found in one GRN in the DPS/VPS, where it is co-expressed with Gr28b.c-GAL4 as well as Gr28a-GAL4 (Figure 1D). In the PPS, none of the Gr28b genes is expressed, but Gr28a-GAL4 is found in two neurons, one of which also expresses Gr66a-LexA (Figure 1D). In summary, the internal sensory organs can be subdivided into three distinct groups (Figure 1E): Gr66a/Gr28b.c/Gr28a-positive neurons, Gr66a/Gr28a-positive neurons, and Gr28aonly neurons, which contrasts the external sensory organs where Gr28a and the Gr28b genes are expressed in a mutually exclusive fashion.
Subsets of Gr28 neurons mediate opposing feeding behaviors
We previously showed that at least one of the six Gr28 genes is necessary for feeding attraction to RNA, ribonucleosides, and ribose using a well-established two-choice feeding preference assay (Mishra et al., 2018; Figure 2A). Specifically, larvae homozygous mutant for Gr28 (ΔGr28, a deletion of the entire Gr28a gene and more than half of the coding region of all Gr28b genes, Figure 1B) lose their ability to sense these compounds, a phenotype that is restored when single UAS-Gr28 reporter transgenes are expressed in Gr28a-GAL4 neurons. The largely non-overlapping expression of Gr28a and the Gr28b genes suggests that respective neurons represent functionally distinct entities. To investigate this possibility, we took advantage of the mammalian VR1 protein, a TRP channel that is activated by capsaicin (Caterina et al., 1997). Drosophila have no VR1 like-gene in their genome and do not respond to capsaicin behaviorally, but flies are attracted to this chemical when a modified VR1 gene (VR1E600K) is expressed in sweet taste neurons (Marella et al., 2006). Thus, we expressed VR1E600K (henceforth referred to UAS-VR1) under the control of the four Gr28-GAL4 drivers (Gr28a-GAL4, Gr28b.a-GAL4, Gr28b.c-GAL4, and Gr28b.e-GAL4) in larvae and tested their response to capsaicin using the two-choice preference assay (Figure 2A). Additionally, we expressed UAS-VR1 in bitter neurons as well as appetitive fructose sensing neurons using respective GAL4 drivers (Gr66a-GAL4 and Gr43aGAL4) (Mishra et al., 2013; Scott et al., 2001), which served as control larvae (note that fructose sensing Gr43aGAL4 neurons are distinct from Gr28a-GAL4 neurons; Mishra et al., 2018). Just like adult flies, w1118 control larvae lacking either a UAS-VR1 reporter, a Gr-GAL4 driver, or both, were unresponsive to 0.1 mM capsaicin, while the positive control larvae expressing UAS-VR1 in either fructose sensing GRNs or bitter taste GRNs showed robust attraction to or avoidance of capsaicin (Figure 2B). Consistent with previous findings, which identified Gr28a GRNs as appetitive neurons (Mishra et al., 2018), larvae expressing UAS-VR1 under the control of Gr28a-GAL4 showed strong appetitive responses to capsaicin (Figure 2B). In contrast, larvae expressing UAS-VR1 under the control of Gr28b.c-GAL4 showed robust avoidance behavior to capsaicin, while expression in the single TOG GRN using either Gr28b.a-GAL4 or Gr28b.e-GAL4 (also expressing Gr28b.c; Figure 1C) caused neither attraction to nor avoidance of capsaicin.
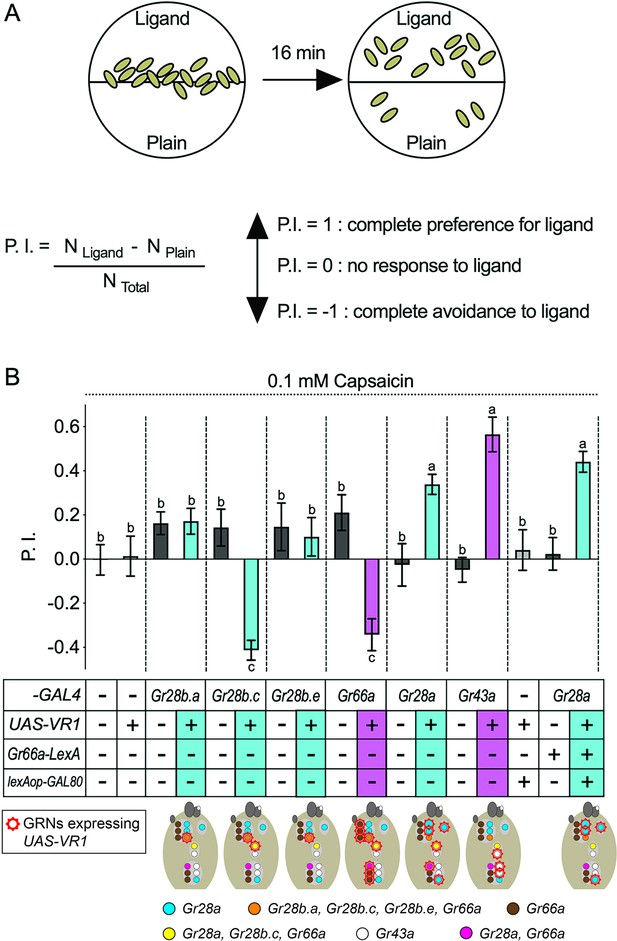
Intrinsic valence of different Gr28 gustatory receptor neurons (GRNs).
(A) Behavioral two-choice preference assay to quantify appetitive and avoidance of larvae for chemical ligands. Fifteen feeding stage, third-instar larvae are placed along the demarcation of a 1% agarose containing dish (35 mm), with one side plain, and the other side containing ligand. The preference index (P.I.; see ‘Materials and methods’) is calculated after counting location of larvae after 16 min. (B) Gr28a GRNs mediate capsaicin preference, while Gr28b.c GRNs elicit capsaicin avoidance in larvae. GRNs expressing the capsaicin receptor VR1 are marked with a red crown in diagrams below. w1118, reporter gene only (UAS-VR1/+), and respective GAL4 driver only (Gr-GAL4/+) larvae serve as negative controls (white panels) and show neither preference for nor avoidance to 0.1 mM capsaicin. Experimental larvae expressing VR1 in Gr28-GAL4 neurons are shown in blue. Positive control larvae (purple panels) expressing VR1 in bitter taste GRNs (Gr66a-Gal4) or appetitive, sweet GRNs (Gr43aGAL4) show expected avoidance to or preference for capsaicin. Experimental larvae (blue panels) expressing VR1 in Gr28a-GAL4 GRNs display robust preference for capsaicin, which is still observed when expression is further restricted to Gr28aonly GRNs (Gr66a-LexA/UAS-VR1; Gr28a-GAL4/lexAop-GAL80; right panel). In contrast, when VR1 is expressed in Gr28b.c-GAL4 GRNs, larvae strongly avoid capsaicin. Neither avoidance nor preference was observed when VR1 is expressed in the Gr28b.a-GAL4 or Gr28b.e-GAL4 GRNs. Each bar represents the mean ± SEM of P.I. (n = 10–22 assays). The taste behavior of Gr-GAL4>UAS-VR1 larvae is compared to three controls (w1118, UAS-VR1/+ and Gr-GAL4/+) using one-way ANOVA with Bonferroni’s multiple comparison tests (p<0.05), whereby different letters indicate a statistically significant difference. Dashed lines delineate groups for ANOVA. All control and experimental larvae are in the w1118 background, carry one copy of the indicated transgene(s), and were generated from crosses of respective strains listed in ‘Materials and methods’.
-
Figure 2—source data 1
Taste preference assay for 0.1 mM capsaicin of larvae expressing VR1 in different GRNs.
Taste preference assay of larvae with expression of VR1 in Gr28aonly GRNs using lexAop-GAL80 under control of Gr66a-LexA for 0.1 mM capsaicin.
- https://cdn.elifesciences.org/articles/89795/elife-89795-fig2-data1-v1.xlsx
Overlap between Gr28a and Gr66a in internal GRNs raises the question about their contribution to appetitive and/or avoidance behavior. We deemed it unlikely that these neurons were critical for appetitive behavior since the one pair located in the DPS/VPS (expressing also Gr28b.c) is necessary for capsaicin avoidance (see above). Indeed, when VR1 was suppressed in all Gr66a/Gr28b.c neurons by means of the GAL4 suppressor GAL80, these larvae, expressing VR1 in Gr28aonly GRNs, remained strongly attracted to capsaicin (Figure 2B, last panel; for effective suppression, see Figure 2—figure supplement 1). Taken together, this analysis indicates that Gr28a-GAL4 is expressed in appetitive-inducing neurons, while Gr28b.c-GAL4 neurons mediate avoidance behavior.
The capsaicin experiments above suggest that the two larval Gr28b.c-GAL4 GRNs mediate negative valence. Previous reports have shown that larvae avoid many bitter compounds (Apostolopoulou et al., 2016; Apostolopoulou et al., 2015; Choi et al., 2020; Choi et al., 2016; van Giesen et al., 2016), and thus, we expected that eliminating activity of Gr28b.c-GAL4 GRNs would result in loss of avoidance behavior to at least some bitter chemicals. Indeed, when Gr28b.c-GAL4 GRN activity was blocked using the inward-rectifying potassium channel Kir2.1 (Baines et al., 2001; Paradis et al., 2001), larvae no longer avoided the four tested bitter compounds denatonium, quinine, lobeline, and caffeine (Figure 3). A compound-specific avoidance phenotype to lobeline and caffeine was observed when the Gr28b.e-GAL4 GRNs in the TOG were inactivated. These data, together with the capsaicin experiments, suggest that two pairs of GRNs, one in the TOG and one in the DPS/VPS, are necessary and sufficient for avoidance of these four bitter tasting chemicals.
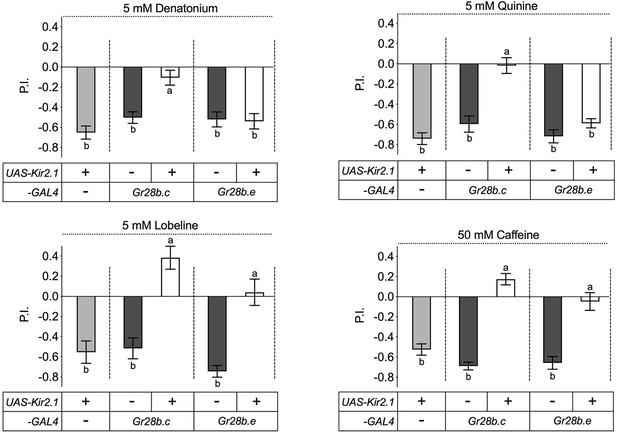
The Gr28b.c neurons mediate avoidance behavior to bitter compounds.
Inactivation of Gr28b.c gustatory receptor neurons (GRNs) (Gr28b.c-GAL4/UAS-Kir2.1) elicits significantly reduced avoidance of larvae to all four bitter compounds tested – denatonium, quinine, lobeline, and caffeine – while control larvae, carrying either the driver or the reporter only, showed strong avoidance of these compounds. In contrast, larvae with inactivated Gr28b.e GRNs (Gr28b.e-GAL4/UAS-Kir2.1) still avoid denatonium and quinine (top), but no longer avoid lobeline and caffeine (bottom). Each bar represents the mean ± SEM of preference index (P.I.) (n = 11–20 assays). The taste behavior of Gr28b.c-GAL4; UAS-Kir2.1 and Gr28b.e-GAL4; UAS-Kir2.1 larvae was compared to two controls (UAS-Kir2.1/+ and Gr28b.c -GAL4/+ or Gr28b.e-GAL4/+) using Kruskal–Wallis test by ranks with Dunn’s multiple comparison tests (p<0.05). Bars with different letters are significantly different. Dashed lines delineate groups for ANOVA. Fly genotypes: w1118; UAS-Kir2.1/+ (light gray), w1118; Gr28b.c-GAL4/+ (dark gray), w1118; Gr28b.c-GAL4/UAS-Kir2.1 (white), w1118; Gr28b.e-GAL4/+ (dark gray), w1118; Gr28b.e-GAL4/UAS-Kir2.1 (white).
-
Figure 3—source data 1
Taste preference assay for bitter compounds of larvae with inactivated Gr28b.c or Gr28b.e GRNs using expression of UAS-Kir2.1.
- https://cdn.elifesciences.org/articles/89795/elife-89795-fig3-data1-v1.xlsx
Gr28b.c and Gr28b.a are subunits of a taste receptor complex for denatonium
We next examined whether any of the Gr28b proteins is part of a taste receptor complex detecting any of these bitter chemicals (Figure 4). Surprisingly, only avoidance of denatonium was affected in larvae lacking the Gr28 gene cluster (ΔGr28; Figure 1B), while avoidance to quinine was somewhat reduced, albeit not significantly, and avoidance to lobeline or caffeine remained robust. In fact, avoidance to caffeine increased modestly, but significantly (Figure 4A). We then examined whether any of the Gr28 proteins was sufficient to restore denatonium avoidance by expressing individual Gr28 genes under the control of the Gr28b.c-GAL4 driver. Indeed, either Gr28b.a or Gr28b.c expression led to a full recovery of denatonium avoidance, while expression of any other Gr28b gene, or Gr28a, failed to do so (Figure 4B). This observation suggests that despite the high level of similarity between these receptors, recognition of denatonium is dependent on specific structural features present in Gr28b.a and Gr28b.c, but not in any of the other Gr28 proteins.
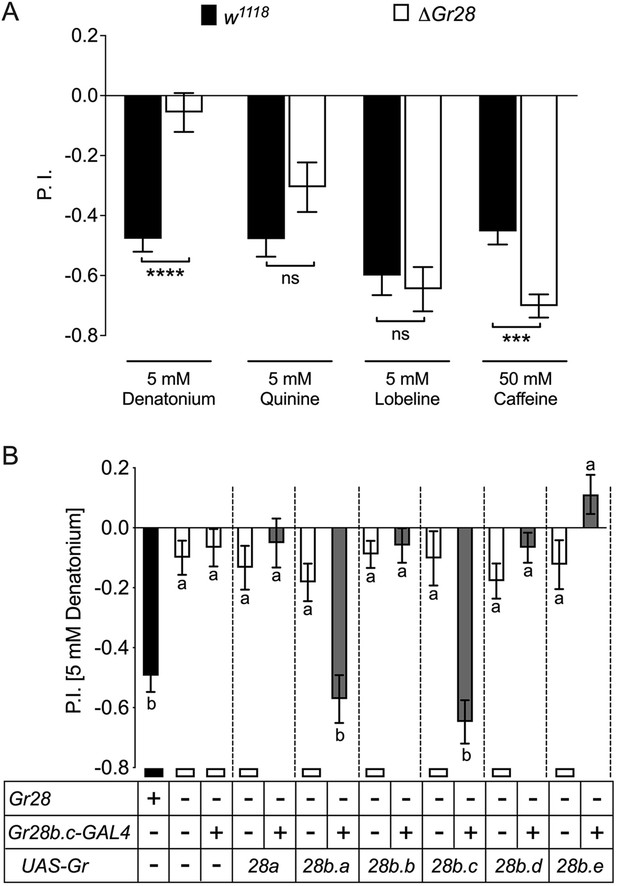
Role of individual Gr28 genes in bitter taste avoidance.
(A) Gr28 genes are required for sensing denatonium. Wild-type (w1118) larvae, but not Gr28 mutant larvae (w1118;ΔGr28/ΔGr28), strongly avoid denatonium. In contrast, w1118;ΔGr28/ΔGr28 larvae do not show significantly reduced avoidance to quinine, lobeline and caffeine. Each bar represents the mean ± SEM of preference index (P.I.) (n = 12–22 assays). Asterisks indicate a significant difference between w1118; ΔGr28/ΔGr28 and w1118 larvae (two-tailed, Mann–Whitney U test, ****p<0.0001, ***p<0.001, ns, not significant). (B) Single Gr28b genes can rescue avoidance response to denatonium when expressed in Gr28b.c neurons of Gr28 mutant larvae. The behavior of w1118;ΔGr28/ΔGr28 larvae expressing UAS-Gr28 transgenes under control of the Gr28b.c-GAL4 driver was compared to Gr28+ control (w1118, black bar), and three Gr28 mutant controls (ΔGr28, ΔGr28 plus driver and ΔGr28 plus respective UAS-Gr28 transgene, white bar) using Kruskal–Wallis test by ranks with Dunn’s multiple comparison tests (p<0.05). Each bar represents the mean ± SEM of P.I. (n = 11–22 assay). Bars with different letters are significantly different. Dashed lines delineate groups for ANOVA. Fly genotypes: wild-type: w1118 (black), mutants: w1118;ΔGr28/ΔGr28, w1118; ΔGr28/ΔGr28 Gr28b.c-GAL4, w1118;ΔGr28/ΔGr28; UAS-Gr28 (indicated Gr28 genes)/+ and w1118; ΔGr28/ΔGr28 UAS-GCaMP6m; UAS-Gr28 (for Gr28b.b or Gr28b.c genes)/+ (white), rescues: w1118; ΔGr28/ΔGr28 Gr28b.c-GAL4; UAS-Gr28 (indicated Gr28 genes)/+ and w1118; ΔGr28 UAS-GCaMP6m/ΔGr28 Gr28b.c-GAL4; UAS-Gr28 (for Gr28b.b or Gr28b.c genes)/ + (gray).
-
Figure 4—source data 1
Taste response to bitter compounds of Gr28 mutant larvae.
(A) Taste preference assay of wild-type and Gr28 mutant larvae for bitter compounds. (B) Taste preference assay of Gr28 mutant larvae for denatonium expressing single Gr28 genes in Gr28b.c GRNs.
- https://cdn.elifesciences.org/articles/89795/elife-89795-fig4-data1-v1.xlsx
Since Gr66a-LexA is co-expressed in all Gr28b-GAL4-expressing GRNs, we wondered whether Gr66a is a component of the denatonium receptor. Previous work had established that Gr66a is required for caffeine avoidance in both larvae and adult flies (Apostolopoulou et al., 2016; Lee et al., 2009; Moon et al., 2006), which we confirmed (Figure 4—figure supplement 1). Surprisingly, avoidance of denatonium and quinine was not diminished, but increased significantly (Figure 4—figure supplement 1). Given the multimeric nature of bitter taste receptors (Sung et al., 2017), one possibility is that the absence of a Gr subunit not required for the detection of denatonium (Gr66a) could favor formation of multimeric complexes containing Gr subunits that recognize this compound (such as Gr28b.a and/or Gr28b.c).
Finally, we investigated neuronal responses in larvae expressing the Ca2+ indicator GCaMP6m in Gr28b.c-GAL4 GRNs (Figure 5). We developed a whole animal imaging preparation, whereby larvae were placed in an ‘imaging chamber’ to minimize head movements (Figure 5A), and visualized neural activity of the Gr28b.c-GAL4 GRN in the TOG in real time (Chen et al., 2013) upon exposure to the four bitter compounds, as well as sucrose, ribose, and fructose (Figure 5B–D). All bitter compounds elicited rapid Ca2+ increases in Gr28b.c-GAL4 GRNs, while none of the sugars did (Figure 5C and D). When neural activity was recorded in Gr28b.c-GAL4 GRNs of ΔGr28 homozygous mutant larvae (Figure 5E), Ca2+ responses to denatonium and quinine were severely reduced, while responses to both caffeine and lobeline were not affected. Re-expression of either Gr28b.a or Gr28b.c, but not Gr28b.b, Gr28b.d, Gr28b.e, or Gr28a rescued Ca2+ response to denatonium, but not to quinine (Figure 5F and G). Together, these experiments identified Gr28b.c and Gr28b.a as redundant subunits of a denatonium receptor complex, a complex that does not require Gr66a or any of the other Gr28b subunits.
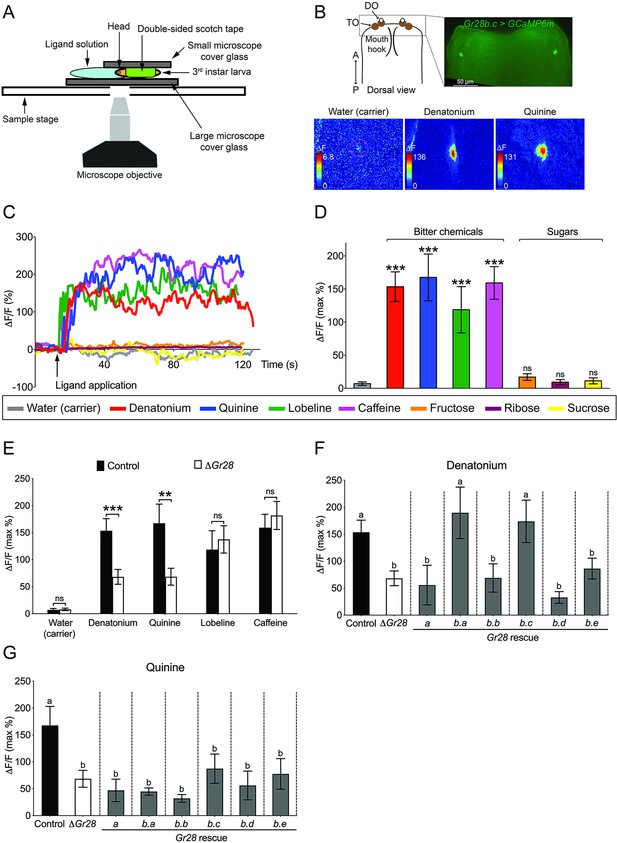
Cellular Ca2+ responses of Gr28b.c gustatory receptor neurons (GRNs) to select bitter compounds requires Gr28b.a or Gr28b.c.
(A) Diagram of Ca2+ imaging experimental set up. (B) Representative still images of Ca2+ response in the Gr28b.c expressing GRN of the TOG. Ca2+ responses of the Gr28b.c GRNs upon stimulation with indicated ligands. ΔF indicates the changes in fluorescence light intensity of the cell body after ligand application. (C, D) Representative traces (C) and quantified Ca2+ responses (D) of the Gr28b.c GRNs after stimulation with indicated ligands. Fly genotype: w1118; Gr28b.c-GAL4/UAS-GCaMP6m. Each bar represents the mean ± SEM of Ca2+ imaging with 12–16 larvae. Asterisks indicate a significant difference between carrier (water) and indicated ligands (two-tailed, Mann–Whitney U test, ***p<0.001, ns, not significant). (E) Neurons of larvae lacking the Gr28 genes exhibit significantly reduced responses to denatonium and quinine. Gr28b.c-expressing GRNs in the TOG of Gr28 mutant larvae (ΔGr28) have significantly reduced Ca2+ responses to denatonium and quinine but not to lobeline or caffeine when compared to Gr28b.c-expressing GRNs of wild-type controls. Larvae genotypes: Gr28+ control (black bar): w1118; Gr28b.c-GAL4/UAS-GCaMP6m. ΔGr28 control (white bar): w1118; ΔGr28 Gr28b.c-GAL4/ΔGr28 UAS-GCaMP6m. Each bar represents the mean ± SEM with 13–16 larvae. Asterisks indicate a significant difference between Gr28+ and ΔGr28 larvae (two-tailed, Mann–Whitney U test, ***p<0.001, **p<0.01; ns, not significant). (F, G) Gr28b.c or Gr28b.a transgenes rescue denatonium responses in Gr28b.c-GAL4 neurons of ΔGr28 larvae. Expression of Gr28b.c or Gr28b.a is under control of Gr28b.c-GAL4 restores responses to denatonium, but not quinine in TOG GRNs of ΔGr28 larvae. Each bar represents the mean ± SEM of Ca2+ imaging with 12–17 larvae. The Ca2+ responses of Gr28 mutant larvae expressing UAS-Gr28 transgenes under Gr28b.c-GAL4 driver is compared to Gr28+ (black) and ΔGr28 (white) controls using Kruskal–Wallis test by ranks with Dunn’s multiple comparison tests (p<0.05). Bars with different letters are significantly different. Dashed lines delineate groups for ANOVA. Fly genotypes: Gr28+ control (black bar): w1118; Gr28b.c-GAL4/UAS-GCaMP6m. ΔGr28 control (white bar): w1118; ΔGr28 Gr28b.c-GAL4/ΔGr28 UAS-GCaMP6m. Gr28 rescues (gray bar): w1118; ΔGr28 Gr28b.c-GAL4/ΔGr28 UAS-GCaMP6m; UAS-Gr28 (indicated Gr28 genes)/+. Concentration of ligands was 100 mM for sugars, 50 mM for caffeine, and 5 mM for denatonium, quinine, and lobeline.
-
Figure 5—source data 1
Ca2+ imaging experiments with Gr28b.c GRNs in the TOG.
(D) Ca2+ responses of Gr28b.c GRNs in the TOG to bitter compounds and sugars.
(E) Ca2+ responses of Gr28 mutant larvae to bitter compounds. (F, G) Ca2+ responses of Gr28 mutant larvae to denatonium (F) or quinine (G) expressing single Gr28 genes in Gr28b.c GRNs.
- https://cdn.elifesciences.org/articles/89795/elife-89795-fig5-data1-v1.xlsx
Discussion
The Gr28 receptors comprise six related Gr proteins (Figure 6), forming one of the few Gr subfamilies conserved across diverse insect species (Agnihotri et al., 2016; Engsontia and Satasook, 2021; Yu et al., 2023). Yet, they were the least characterized when compared to other conserved subfamilies, such as the sugar receptors (Gr5a, Gr61a, and Gr64a-f), the carbon dioxide receptors Gr21a and Gr63a, or the bitter taste receptors. The only ligands associated with the Gr28 proteins were ribonucleosides and RNA, which are appetitive nutrients essential for larvae and detected by Gr28a neurons (Mishra et al., 2018). Indeed, RNA has been found to be an appetitive taste ligand across many dipteran insects, including mosquitoes, and we showed that Gr28 homologs of both A. aegypti and A. gambiae can rescue the preference for RNA and ribose when expressed in Gr28a neurons of ΔGr28 mutant larvae (Fujii et al., 2023).
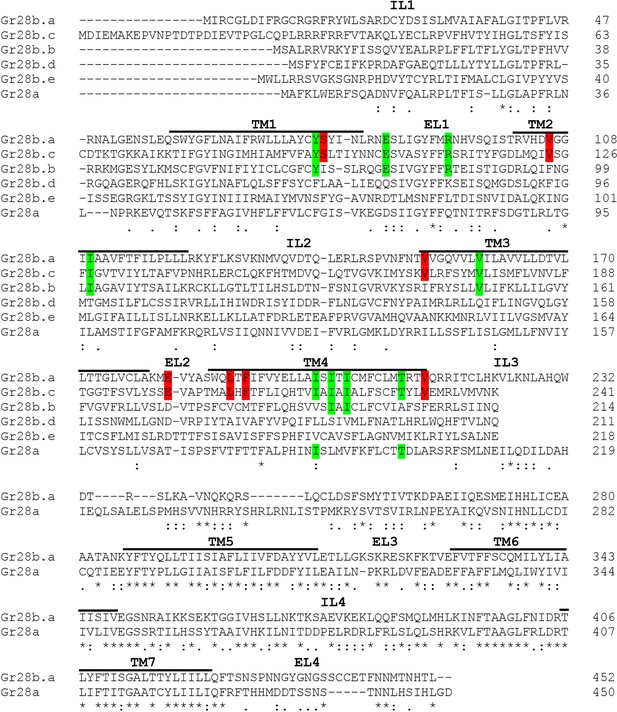
Amino acid alignment of the six Gr28 proteins.
The sequence alignment was generated using Clustal Omega tool from ClustalW2 (https://www.ebi.ac.uk/Tools/msa/clustalo/). IL and EL indicate intracellular loop and extracellular loop, respectively. Note that the C terminal region starting at the IL3 is identical in the Gr28b proteins. Red highlighted letters indicate amino acids identical only in Gr28b.a and Gr28b.c. Green highlighted letters indicate amino acids conserved in Gr28b.a, Gr28b.c, and one other Gr28 protein. Asterisks below the sequences indicate residues identical in all Gr28 proteins, colons indicate conserved residue (STA, NEQK, NHQK, NDEQ, QHRK, MILV, MILF, HY, FYW), and periods indicate moderately conserved residue (CSA, ATV, SAG, STNK, STPA, SGND, SNDEQK, NDEQHK, NEQHRK, FVLIM, HFY). TM1-7 indicate helical transmembrane segments predicted using HMMTOP 2.0 software.
Previous studies in adult Drosophila have shown that members of conserved Gr protein families such as the carbon dioxide receptors (Gr21a and Gr63a) (Jones et al., 2007; Kwon et al., 2007) or the receptors for sweet taste encoded by the eight sugar Gr genes (Fujii et al., 2015) are largely co-expressed in one type of neuron in the fly’s taste organs. For example, with the exception of Gr5a (see below), sugar Gr genes are only expressed in a single GRN (the ‘sweet’ neuron) of each taste sensilla, and activation of these ‘sweet’ neurons by sugars requires the function of at least two of the eight sugar Gr genes (Dahanukar et al., 2007; Yavuz et al., 2014; Fujii et al., unpublished). Similarly, the approximately 33 putative bitter taste receptors, which comprise several small conserved subfamilies (Robertson et al., 2003), as well as individual Gr genes with little overall similarity to one another, are partially co-expressed in the bitter GRN of each taste sensilla (Weiss et al., 2011). Molecular genetic studies combined with electrophysiological recordings have shown that at least three different Gr subunits are required to constitute functional receptor complexes that can sense a bitter compound (Shim et al., 2015). We note that two rare exceptions to the heteromeric nature of taste receptor complexes exist, namely the RNA receptor Gr28a and the fructose receptor Gr43a, which have been proposed to function as homomultimeric complexes (Mishra et al., 2018; Mishra et al., 2013). Cryo-EM structural analysis of the conserved insect olfactory receptor co-receptor (ORCO) suggests that insect odorant receptors form tetramers (Butterwick et al., 2018), and biochemical characterization and comparative modeling of BmGr9, the Bombyx mori homolog of the Drosophila Gr43a fructose receptor, supports such structures for Gr proteins as well (Morinaga et al., 2022).
Distinct functions are mediated by small set of GRNs expressing specific Gr28 subunits
Our expression analysis of the bitter taste receptor gene Gr66a and the Gr28 genes in larvae is consistent with earlier studies, despite some small variation in neuron number (Choi et al., 2016; Kwon et al., 2011), which is likely due to the use of different GAL4 driver lines and/or variability in expression levels. Importantly, all Gr28b genes are co-expressed with the bitter taste receptor gene Gr66a (Figure 1) and probably several other putative bitter Gr genes (Kwon et al., 2011; Rist and Thum, 2017), while Gr28a is found in a largely, but not entirely, distinct set of GRNs. Whether and what kind of Gr genes might be co-expressed with Gr28a in Gr28aonly GRNs will require more in-depth expression studies and might shed light on other receptors involved in appetitive behaviors of larvae.
A key finding of the work presented here is the observation that Gr28aonly and Gr28b.c neurons dictate distinct behavioral programs, the former representing an ensemble of neurons that instruct larvae to ‘go toward’ a chemical source and consume it, while the latter do the opposite (Figure 2). This observation is reminiscent of a seminal study by Troemel and colleagues in the Caenorhabditis elegans chemosensory system, who reported that the valence of a chemical compound is dependent on the identity of a neuron, and not the identity of the molecular receptor the neuron expresses (Troemel et al., 1997). The number of ‘go-away’ GRNs in Drosophila larvae co-expressing Gr28b.c and Gr66a is remarkably small, consisting of only two pairs, one in the TO and the other in the DPS/VPS. It seems likely that this is the smallest, minimal subset of neurons sufficient to induce avoidance behavior as expression of VR1 in only the TO pair (under the control of either Gr28b.a-GAL4 or Gr28b.e-GAL4) has no behavioral effect when challenged with capsaicin. The ‘go-to’ neurons are characterized by expression of Gr28a and represent a slightly larger set of four GRN pairs (Gr28aonly GRNs) (Figure 7) . Thus, the minimal requirement to induce ‘go-to’ and ‘go-away’ behavior is defined by distinct sets of GRNs, and each appears to be composed of neurons located in both external and the internal taste organs. Co-expression of the RNA taste receptor Gr28a in the DPS/VPS GRN essential for bitter taste (Figures 2B and 3) raises interesting questions about additional functions for Gr28a in bitter taste. We note that the sweet taste receptor Gr5a, a subunit of a multimeric trehalose receptor, is also expressed in non-sweet neurons of unknown function (Fujii et al., 2015).
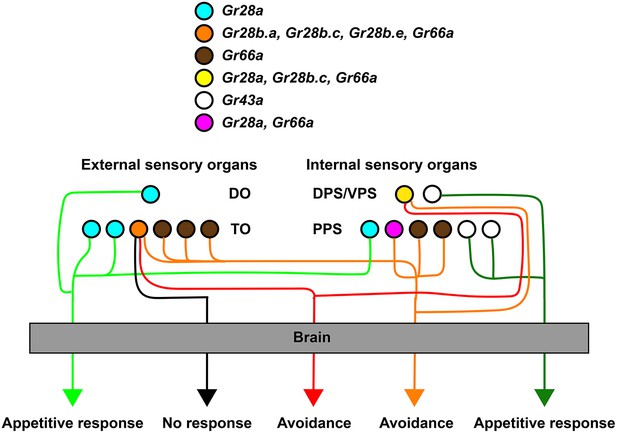
Role of different gustatory receptor neuron (GRN) subsets in taste behavior of larvae.
GRNs sufficient for mediating avoidance behavior can be defined by Gr28b.c-GAL4, while GRNs sufficient for mediating appetitive behavior are defined by a subset of Gr28a-GAL4 GRNs (Gr28aonly GRNs; see also Figure 2B and Figure 2—figure supplement 1). Note that each ensemble is composed of at least a pair of neurons located in the external taste organs and a pair of neurons in the internal taste organs. Also, a larger set of avoidance neurons (Gr66a-GAL4) might function independently of any Gr28b.c neurons, and one set of fructose sensing neurons (Gr43aGAL4) distinct from Gr28a-GAL4 GRNs mediates appetitive behavior.
Functional redundancy of taste receptors
Both behavioral analyses and Ca2+ imaging experiments implicate at least one Gr28b protein as an essential component of a denatonium receptor complex as ΔGr28 larvae exhibit total loss of avoidance (Figure 4) and respective GRNs fail to elicit a response upon exposure to this chemical (Figure 3). What the precise composition of that complex is remains to be determined, but recovery of denatonium responses by expressing either Gr28b.c and/or Gr28b.a indicates that either one of these (or possibly both) is an essential subunit, in addition to other Grs expressed in this GRNs, such as Gr22a and Gr59c (Choi et al., 2020; Rist and Thum, 2017), while Gr66a is unlikely to be part of such a complex based on our behavioral analysis (Figure 4—figure supplement 1).
Since only Gr28b.a and Gr28b.c can rescue denatonium responses in Gr28b.c GRNs of ΔGr28 mutant larvae, sequence comparison between the unique N-terminal halves of the Gr28 proteins comprising the first four transmembrane domains and the extracellular loops 1 and 2 might provide insights as to possible residues important for ligand recognition. When interrogating these regions, only seven residues are identical between Gr28b.a and Gr28b.c (Table 1 and Figure 6). Reducing the stringency requirement by allowing one of the remaining receptors to share the same residue, nine additional sites are identified. One experimental avenue to validate these residues as important sites contributing to denatonium binding might involve introduction of point mutations that converts respective amino acids of other Gr28b proteins into those found in Gr28b.a/Gr28b.c.
Conserved amino acids in the amino termini of Gr28b.a and Gr28b.c.
The seven amino acid residues identical in the amino-terminal region of Gr28b.c and Gr28b.a are shown in bold (residue number is taken from Gr28b.c). The nine additional amino acids also identical in one additional Gr28 proteins are also listed. These residues are considered potentially critical for recognition of denatonium since only Gr28b.a and Gr28b.c can rescue response to denatonium when expressed in Gr28b.c neurons of ΔGr28/ΔGr28 mutant larvae.
Location | Conserved in Gr28b.c/Gr28b.a | Other (if applicable) |
---|---|---|
EL1 | E103 | Gr28b.b |
R111 | Gr28b.b | |
EL2 | E200 | None |
TM1 | Y94 | Gr28b.b |
S95 | None | |
TM2 | V124 | None |
I128 | Gr28b.b | |
TM3 | V170 | None |
V177 | Gr28b.b | |
TM4 | L207 | None |
F209 | None | |
I218 | Gr28a | |
I220 | Gr28b.b | |
I222 | Gr28b.b | |
T229 | Gr28a | |
V232 | none |
The role of Gr28b proteins in quinine detection is less clear, and the different phenotypes observed in behavioral experiments and Ca2+ imaging suggest that at least two molecular types of quinine receptors exist in larvae. Ca2+ imaging experiments implicate a role for multiple Gr28b subunits in a quinine receptor complex in the TOG neuron since single Gr28b genes cannot restore the loss of quinine response in ΔGr28 larvae (Figure 5E and G). However, because ΔGr28 mutant larvae still avoid quinine (Figure 4), at least one Gr28b-independent receptor must exist in one or several other GRNs, one of which is likely the 28b.c GRN in the DPS/VPS, since expression of Kir2.1 in that neuron, along with the one in the TOG, totally abolishes quinine avoidance (Figure 3). We note that functional redundancy is also observed in sweet taste receptors, where we found that different combinations of sugar Gr genes can restore responses to the same sugar when expressed in tarsal sweet GRNs of sugar blind flies (Fujii et al. unpublished).
Materials and methods
Reagent type (species) or resource | Designation | Source or reference | Identifiers | Additional information |
---|---|---|---|---|
Antibody | Anti-GFP (rabbit polyclonal) | Thermo Fisher Scientific | Cat# A6455, RRID:AB_221570 | IF (1:1000) |
Antibody | Anti-mCD8 (rat monoclonal) | Thermo Fisher Scientific | Cat# MCD0800, RRID:AB_10392843 | IF (1:200) |
Antibody | Anti-rabbit Alexa 488 (goat polyclonal) | Thermo Fisher Scientific | Cat# A11070, RRID:AB_2534114 | IF (1:500) |
Antibody | Anti-rat Cy3 (goat polyclonal) | Jackson ImmunoResearch Laboratories Inc | Cat# 112-165-072, RRID:AB_2338248 | IF (1:300) |
Chemical compound, drug | Caffeine | MilliporeSigma | C0750 | |
Chemical compound, drug | Capsaicin | MilliporeSigma | M2028 | |
Chemical compound, drug | Denatonium benzoate | MilliporeSigma | D5765 | |
Chemical compound, drug | Lobeline hydrochloride | MilliporeSigma | 141879 | |
Chemical compound, drug | D-(-)-ribose | MilliporeSigma | R7500 | |
Chemical compound, drug | Quinine hydrochloride dihydrate | MilliporeSigma | Q1125 | |
Chemical compound, drug | Fructose | Spectrum Chemical | F1092 | |
Chemical compound, drug | Agarose | Apexbio | 20-102 | |
Chemical compound, drug | Sucrose | Macron Fine Chemicals | 8360-06 | |
Chemical compound, drug | Charcoal | J.T. Baker | 1560-01 | |
Genetic reagent (Drosophila melanogaster) | w1118 | Bloomington Drosophila Stock Center | BDSC: 3605; FLYB: FBst0003605 | |
Genetic reagent (D. melanogaster) | Gr28a-GAL4 | Thorne and Amrein, 2008 | FLYB: FBtp0056017 | FlyBase symbol: w*; P{Gr28a-GAL4.T}SF36S |
Genetic reagent (D. melanogaster) | Gr28a-GAL4 | Thorne and Amrein, 2008 | FLYB: FBtp0056017 | FlyBase symbol: w*; P{Gr28a-GAL4.T}SF36B1 |
Genetic reagent (D. melanogaster) | Gr28b.a-GAL4 | Thorne and Amrein, 2008 | FLYB: FBtp0054526 | FlyBase symbol: w*; P{Gr28b.a-GAL4}NT42aC51a |
Genetic reagent (D. melanogaster) | Gr28b.c-GAL4 | Thorne and Amrein, 2008 | FLYB: FBtp0054528 | FlyBase symbol: w*; P{Gr28b.c-GAL4}NT21B1 |
Genetic reagent (D. melanogaster) | Gr28b.e-GAL4 | Scott et al., 2001 | FLYB: FBtp0014672 | FlyBase symbol: w*; P{Gr28b.e-GAL4.4.245}Gr28a3AII |
Genetic reagent (D. melanogaster) | ΔGr28/ΔGr28 | Mishra et al., 2018 | FLYB: FBab0049019 | FlyBase symbol: w*; Df(2L)ΔGr28 |
Genetic reagent (D. melanogaster) | Gr66a-GAL4 | Scott et al., 2001 | FLYB: FBtp0014661 | FlyBase symbol: w*; P{Gr66C1-GAL4.3.153} |
Genetic reagent (D. melanogaster) | UAS-Gr28a | Ni et al., 2013 | FLYB: FBal0344045 | FlyBase symbol: w*; P{UAS-Gr28a.G}attP2 |
Genetic reagent (D. melanogaster) | UAS-Gr28b.a | Ni et al., 2013 | FLYB: FBal0291410 | FlyBase symbol: w*; P{UAS-Gr28b.A}attP2 |
Genetic reagent (D. melanogaster) | UAS-Gr28b.b | Ni et al., 2013 | FLYB: FBal0291412 | FlyBase symbol: w*; P{UAS-Gr28b.B}attP2 |
Genetic reagent (D. melanogaster) | UAS-Gr28b.c | Ni et al., 2013 | FLYB: FBal0291411 | FlyBase symbol: w*; P{UAS-Gr28b.C}attP2 |
Genetic reagent (D. melanogaster) | UAS-Gr28b.d | Ni et al., 2013 | FLYB: FBal0291409 | FlyBase symbol: w*; P{UAS-Gr28b.D}attP2 |
Genetic reagent (D. melanogaster) | UAS-Gr28b.e | Ni et al., 2013 | FLYB: FBal0291408 | FlyBase symbol: w*; P{UAS-Gr28b.E}attP2 |
Genetic reagent (D. melanogaster) | Gr43aGAL4 | Miyamoto et al., 2012 | BDSC:93447; FLYB:FBst0093447 | FlyBase symbol: w1118;Ti{GAL4}Gr43aGAL4 |
Genetic reagent (D. melanogaster) | UAS-VR1E600K | Marella et al., 2006 | FLYB: FBal0215202 | FlyBase symbol: w1118;P{UAS-VR1E600K} |
Genetic reagent (D. melanogaster) | lexAop-rCD2:GFP | Lai and Lee, 2006 | FLYB: FBst0066687 | FlyBase symbol: w*; P{lexAop-rCD2-GFP} |
Genetic reagent (D. melanogaster) | UAS-mCD8:RFP | Bloomington Drosophila Stock Center | BDSC: 32220; FLYB: FBti0131987 | FlyBase symbol: y1w*;P{10XUAS-IVS-mCD8::RFP}su(Hw)attP8 |
Genetic reagent (D. melanogaster) | UAS-GCaMP6m | Bloomington Drosophila Stock Center | BDSC: 42748; FLYB: FBti0151346 | FlyBase symbol: w1118; P{20XUAS-IVS-GCaMP6m}attP40 |
Genetic reagent (D. melanogaster) | UAS-Kir2.1-GFP | Baines et al., 2001; Paradis et al., 2001 | FLYB: FBst0006596 | FlyBase symbol: w*; P{UAS-Hsap\KCNJ2.EGFP}1 |
Genetic reagent (D. melanogaster) | Gr66a-LexA | Thistle et al., 2012 | BDSC: 93024; FLYB: FBst0093024 | FlyBase symbol: w1118; P{Gr66a-lexA.S}2;TM2/TM6B |
Genetic reagent (D. melanogaster) | lexAop-GAL80 | Thistle et al., 2012 | FLYB: FBtp0079728 | FlyBase symbol: w1118; P{lexAop-GAL80. T} |
Genetic reagent (D. melanogaster) | Gr28b.c-LexA | This paper | FlyBase symbol: w1118;P{Gr28b.c-LexA}#8 | |
Sequence-based reagent | Gr28b.c_F | This paper | PCR primers | 5′-AATCTAGGTACCCCGGCTGCTCGTCTCCCTGGATGT-3′ |
Sequence-based reagent | Gr28b.c_R | This paper | PCR primers | 5′-CGTCAAACTAGTGACCGCTTCGTTTGAGCTTCAACC-3′ |
Recombinant DNA reagent | LexA vector CMC105 (plasmid) | This paper Larsson et al., 2004 | Insect expression vector | |
Software, algorithm | NIS-Elements | Nikon | N/A | |
Software, algorithm | Prism software 10.1.0 (264) | GraphPad Software | N/A | |
Software, algorithm | Adobe pPhotoshop 2022 | Adobe | N/A | |
Other | Normal goat serum | SouthernBiotech | Cat# 0060-01 | IF (5%) ‘Materials and methods’ |
Other | Nikon Eclipse Ti inverted microscope | Nikon | N/A | ‘Materials and methods’ |
Other | Nikon A1R confocal microscope system | Nikon | N/A | ‘Materials and methods’ |
Other | PertriPetri dish, 60 × 15 mm | Falcon | REF353004 | ‘Materials and methods’ |
Other | Microscope cover glass, 24 × 50 mm | VWR | 16004-098 | ‘Materials and methods’ |
Other | Microscope cover glass, 12CIR-1 | Thermo Fisher Scientific | 1254580 | ‘Materials and methods’ |
Drosophila stocks
Request a detailed protocolFlies were maintained on standard corn meal food in plastic vials under a 12 hr light/dark cycle at 25°C. The w1118 strain (Bloomington Drosophila Stock Center, number 3605) was used as a wild-type control. Fly strains used: Gr28a-GAL4(SF36S) for Figures 1 and 2B, and SF36E1 for Figure 2B and Figure 2—figure supplement 1, Gr28b.a-GAL4(NT42aC51a), Gr28b.c-GAL4(NT21B1) (Thorne and Amrein, 2008); Gr28b.e-GAL4(Gr28a3AII) and Gr66a-GAL4 (Scott et al., 2001); ΔGr28(54B3) (Mishra et al., 2018); UAS-Gr28a, UAS-Gr28b.a, UAS-Gr28b.b, UAS-Gr28b.c, UAS-Gr28b.d, and UAS-Gr28b.e (Ni et al., 2013); Gr43aGAL4 (Miyamoto et al., 2012); Gr66a-LexA and lexAop-GAL80 (Thistle et al., 2012); UAS-VR1E600K (Marella et al., 2006); UAS-Kir2.1-GFP (Baines et al., 2001; Paradis et al., 2001); lexAop-rCD2:GFP (Lai and Lee, 2006), UAS-GCaMP6m, UAS-mCD8:RFP and Gr66aex83 (Bloomington Drosophila Stock Center, numbers 42748, 32220, and 35528); Gr28b.c-LexA(#8).
Chemicals
Caffeine (Cat# C0750), capsaicin (Cat# M2028), denatonium benzoate (Cat# D5765), lobeline hydrochloride (Cat# 141879), D-(-)-ribose (Cat# R7500), and quinine hydrochloride dihydrate (Cat# Q1125) were purchased from MilliporeSigma, with a purity of >95%. Fructose (Cat# F1092) and agarose (Cat# 20-102) were purchased from Spectrum chemical and Apexbio, respectively. Sucrose (mfr. no. 8360-06) and charcoal (Cat# 1560-01) were purchased from Macron Fine Chemicals and J.T. Baker, respectively. A stock solution for capsaicin (20 mM) was prepared in 70% ethanol and stored at 4°C protected from light for up to 1 y. Stock solutions for bitter chemicals were prepared in Millipore Q water and stored at –20°C. Stock solutions for sugars were prepared in Millipore Q water and stored at 4°C for up to 1 mo. A stock solution for ribose was treated with charcoal (10% of the weight of ribose used for stock solution) overnight at 4°C and sterile-filtrated (0.45 μm) to remove unrelated odor. Stock solutions were diluted to the final concentration using Millipore Q water prior to each experiment.
Immunofluorescence
Request a detailed protocolImmunofluorescence of larval heads was performed based on the protocol described in Croset and colleagues (Croset et al., 2016) with minor modification. Heads of third-instar larvae were dissected using microscissors in phosphate-buffered saline (PBS) and immediately fixed in PBS with 4% paraformaldehyde for 1 hr at 4°C. They were washed six times in washing buffer (PBS with 0.1% Triton X-100) for 20 min and blocked for 1 hr in washing buffer containing 5% heat-inactivated goat serum (SouthernBiotech, Cat# 0060-01), followed by incubation with the primary antibodies (rabbit anti-GFP, 1:1000 dilution; rat anti-mCD8, 1:200 dilution, Thermo Fisher Scientific) at 4°C overnight. The next day, heads were washed six times for 20 min in washing buffer and blocked in washing buffer containing 5% heat-inactivated goat serum for 1 hr, followed by incubation with the secondary antibodies (goat anti-rabbit Alexa 488, 1:500 dilution, Thermo Fisher Scientific; goat anti-rat Cy3, 1:300 dilution, Jackson ImmunoResearch Laboratories Inc) at 4°C overnight. Finally, heads were washed six times in washing buffer for 20 min each at room temperature under gentle agitation. Heads were then mounted with VectaShield (Vector Lab, Cat# H-1200) on a microscope slide and images were obtained using a Nikon A1R confocal microscope system. Adobe Photoshop 2022 was used further to process images.
Larval two-choice preference assay
Request a detailed protocolTwo-choice preference assay of larvae was conducted as described in Mishra et al., 2013 with minor modifications. Flies were placed on standard corn meal food in plastic vials and allowed to lay eggs for 24 hr under a 12 hr light/dark cycle at 25°C. Flies were removed from food vials and feeding-stage third-instar larvae were collected. Agarose food dishes for two-choice preference assay were prepared just prior each experiment as follows: Petri dishes (60 × 15 mm, Falcon, Cat# REF353004) with two halves marked on the bottom were filled with melted plain 1% agarose or 1% agarose containing 1.75% ethanol (for capsaicin preference). After the agarose solidified, one half was removed and replaced with 1% agarose solution containing taste ligands (capsaicin or bitter compound). For each experiment, 15 larvae from food vials were briefly rinsed twice with Millipore Q water and placed along the middle separating pure and ligand containing agarose. After 16 min, images were taken for record keeping and used to calculate larval preference indices. Larvae that crawled onto the wall of a dish or dug in the agarose were excluded. The preference index (P.I.) was calculated as follow: PI = (Ntastant – Nplain)/NTotal, whereby N is the number of larvae in the tastant sector, the plain agarose sector, and the total number, respectively. Positive values indicate a preference for capsaicin or bitter compound while negative values indicate repulsion (avoidance).
Calcium imaging
Request a detailed protocolCalcium imaging was performed in Gr28b.c GRNs expressed in the terminal organ of feeding-stage, third-instar larvae, reared as described for the larval two-choice preference assay. For each experiment, larvae from food vials were briefly rinsed twice with Millipore Q water and were mounted dorsally on a large microscope cover glass (24 × 50 mm, VWR, Cat# 16004-098) using double-sided scotch tape and covered with a small microscope cover glass (12CIR-1, Thermo Fisher Scientific, Cat# 1254580). Millipore Q water (40 µl) was applied to the tip of the larval head, and the preparation was placed on the stage of a Nikon eclipse Ti inverted microscope. Images were obtained every 500 ms, starting 15 s before application and ending 105 s after ligand application. Each recording was initiated by applying water (40 μl) to set a baseline. The first ligand solution (40 μl of bitter chemical or sugar) was applied thereafter, followed by five washes with carrier (100 μl of water). After a 3 min pause to allow the preparation to recalibrate, a second ligand solution (40 μl bitter chemical or sugar) was applied. To assure validity in experiments with Gr28 mutants and rescues, each recording was concluded with application of caffeine, and recordings were included only if caffeine generated a positive response. Baseline fluorescence, which was determined from the average of five frame measurements from a region next to the cell immediately before ligand application, was subtracted from the actual measurements. ∆F/F (%) = (fluorescence light intensity of the cell body – baseline/baseline) × 100. ∆F/F (max %) is the maximum value within 40 s after ligand application.
Generation of transgenic Gr28b.c-LexA flies
Request a detailed protocolTo generate the Gr28b.c-LexA driver, a 1.3 kb DNA fragment immediately upstream of the Gr28b.c start codon was amplified from w1118 flies using a forward (5′-AATCTAGGTACCCCGGCTGCTCGTCTCCCTGGATGT-3′) and a reverse (5′- CGTCAAACTAGTGACCGCTTCGTTTGAGCTTCAACC-3′) primer. Acc65I and SpeI sites included in the primer sequence (underlined) were incorporated such that the amplified fragment was amenable to directional cloning into the LexA vector CMC105 (Larsson et al., 2004). The clone chosen was confirmed by DNA sequence analysis. Transgenic flies were generated by standard P-element transformation of w1118 embryos (Rainbow Transgenic Flies Inc, Camarillo, CA).
Statistical analysis
Request a detailed protocolStatistical analyses were conducted using Prism software 9.5.1 (GraphPad Software). Larval two-choice preference assay and Ca2+ imaging data were analyzed for normal distribution using D’Agostino–Pearson omnibus and Shapiro–Wilk normality tests. When groups did not meet the assumption for normal distribution, nonparametric statistics was used. For comparison between multiple groups, one-way ANOVA or Kruskal–Wallis test by ranks (nonparametric one-way ANOVA) was performed to test for difference of mean or rank distribution. As a post hoc test, Bonferroni’s or Dunn’s (nonparametric) multiple comparison tests were employed to compare two specific groups. One-way ANOVA with Bonferroni’s multiple comparison tests were used in Figure 2B. Kruskal–Wallis test by ranks with Dunn’s multiple comparison tests were used in Figures 3—5F and G. For comparison between two groups, Mann–Whitney U test (nonparametric t -test, Figures 4A,, 5D and E, Figure 2—figure supplement 1B, and Figure 4—figure supplement 1) with two-tailed P- value were used. The sample size for larval two-choice preference assays and Ca2+ imaging experiments werewas based on Mishra et al., 2018.
Data availability
All data generated or analysed during this study are included in the manuscript and supporting file. Source data files have been provided for Figures 2, 3, 4 and 5, and Figure 2—figure supplement 1 and Figure 4—figure supplement 1.
References
-
Gustatory receptors in lepidoptera: chemosensation and beyondInsect Molecular Biology 25:519–529.https://doi.org/10.1111/imb.12246
-
BookChapter 14 - mechanism of taste perception in DrosophilaIn: Zufall F, Munger SD, editors. Chemosensory Transduction. Academic Press. pp. 245–269.https://doi.org/10.1016/B978-0-12-801694-7.00014-7
-
Taste processing in Drosophila larvaeFrontiers in Integrative Neuroscience 9:50.https://doi.org/10.3389/fnint.2015.00050
-
Caffeine taste signaling in Drosophila larvaeFrontiers in Cellular Neuroscience 10:193.https://doi.org/10.3389/fncel.2016.00193
-
Altered electrical properties in Drosophila neurons developing without synaptic transmissionThe Journal of Neuroscience 21:1523–1531.https://doi.org/10.1523/JNEUROSCI.21-05-01523.2001
-
A pair of pharyngeal gustatory receptor neurons regulates caffeine-dependent ingestion in Drosophila larvaeFrontiers in Cellular Neuroscience 10:181.https://doi.org/10.3389/fncel.2016.00181
-
Evolutionary history of chemosensory-related gene families across the arthropodaMolecular Biology and Evolution 34:1838–1862.https://doi.org/10.1093/molbev/msx147
-
RNA taste is conserved in dipteran insectsThe Journal of Nutrition 153:1636–1645.https://doi.org/10.1016/j.tjnut.2023.03.010
-
Molecular and cellular organization of the taste system in the Drosophila larvaThe Journal of Neuroscience 31:15300–15309.https://doi.org/10.1523/JNEUROSCI.3363-11.2011
-
Genetic mosaic with dual binary transcriptional systems in DrosophilaNature Neuroscience 9:703–709.https://doi.org/10.1038/nn1681
-
The molecular basis of sugar sensing in Drosophila larvaeCurrent Biology 23:1466–1471.https://doi.org/10.1016/j.cub.2013.06.028
-
A taste receptor required for the caffeine response in vivoCurrent Biology 16:1812–1817.https://doi.org/10.1016/j.cub.2006.07.024
-
Structural model for ligand binding and channel opening of an insect gustatory receptorThe Journal of Biological Chemistry 298:102573.https://doi.org/10.1016/j.jbc.2022.102573
-
A map of sensilla and neurons in the taste system of Drosophila larvaeThe Journal of Comparative Neurology 525:3865–3889.https://doi.org/10.1002/cne.24308
-
Gustatory processing in Drosophila melanogasterAnnual Review of Entomology 63:15–30.https://doi.org/10.1146/annurev-ento-020117-043331
-
Design of the larval chemosensory systemAdvances in Experimental Medicine and Biology 628:69–81.https://doi.org/10.1007/978-0-387-78261-4_5
-
Evolution of gustatory receptor gene family provides insights into adaptation to diverse host plants in nymphalid butterfliesGenome Biology and Evolution 10:1351–1362.https://doi.org/10.1093/gbe/evy093
-
Atypical expression of Drosophila gustatory receptor genes in sensory and central neuronsThe Journal of Comparative Neurology 506:548–568.https://doi.org/10.1002/cne.21547
Article and author information
Author details
Funding
National Institute on Deafness and Other Communication Disorders (R01 DC018403-01A1)
- Hubert Amrein
National Institute on Deafness and Other Communication Disorders (1R21 DC015327)
- Hubert Amrein
National Institute on Deafness and Other Communication Disorders (1RO1GMDC05606-01)
- Hubert Amrein
The funders had no role in study design, data collection and interpretation, or the decision to submit the work for publication.
Acknowledgements
We thank Tetsuya Miyamoto, Shinsuke Fujii, and Sheida Hedjazi for valuable suggestions throughout the duration of this project and Raquel Sitcheran for comments on the manuscript. We are grateful to Paul Garrity for the UAS-Gr28 reporter strains and the Bloomington Stock Center for numerous Drosophila strains. This work was supported by NIH grants1 R01 DC018403, R21 DC015327, and R01GMDC05606 to H Amrein. Drs. Ahn and Amrein conceived the experiments, Dr. Ahn conducted all experiments, and Dr. Amrein wrote the paper.
Version history
- Sent for peer review:
- Preprint posted:
- Reviewed Preprint version 1:
- Reviewed Preprint version 2:
- Version of Record published:
Cite all versions
You can cite all versions using the DOI https://doi.org/10.7554/eLife.89795. This DOI represents all versions, and will always resolve to the latest one.
Copyright
© 2023, Ahn and Amrein
This article is distributed under the terms of the Creative Commons Attribution License, which permits unrestricted use and redistribution provided that the original author and source are credited.
Metrics
-
- 1,480
- views
-
- 133
- downloads
-
- 5
- citations
Views, downloads and citations are aggregated across all versions of this paper published by eLife.
Citations by DOI
-
- 4
- citations for umbrella DOI https://doi.org/10.7554/eLife.89795
-
- 1
- citation for Version of Record https://doi.org/10.7554/eLife.89795.3