Neural Connectivity: Self-awareness in the retina
The idea that cell adhesion molecules regulate when and where connections form between different neurons has been in place for over 50 years (Sperry, 1963). However, it has become clear that other cues are important to prevent connections such as synapses forming between the branches of an individual neuron (Zipursky and Sanes, 2010). Now, in eLife, Dimitar Kostadinov and Joshua Sanes – both at Harvard University – highlight how this process, which is called self-avoidance, is vital for establishing the neuronal circuitry in the retina of the mammalian eye (Kostadinov and Sanes, 2015).
Self-avoidance can lead to several different arrangements in populations of neurons. For example, cells could be ‘tiled’ so that each cell occupies its own territory (Figure 1A). This presumably requires that the neurons in a given population are ‘repulsed’ by one another, and that the same is true for the branched nerve endings of each individual neuron: this arrangement maximizes the area covered by each cell and minimizes the number of self-crossings.
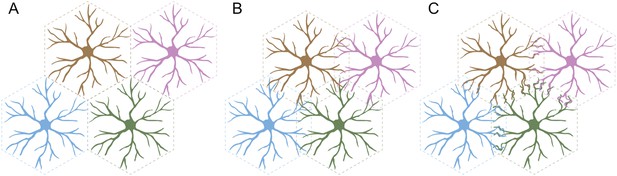
Three arrangements of neurons that require self-avoidance.
(A) Neurons may occupy discrete, non-overlapping territories; this requires repulsion between cells, and self-avoidance to prevent crossings within the branched nerve endings of a single cell. (B) Neurons may avoid their own branches, but be indifferent to those of their neighbors and intermingle without adhering and forming connections. (C) Finally, neurons with self-avoidance may nonetheless bind to their neighbors if they also have self/nonself discrimination. Kostadinov and Sanes studied starburst amacrine cells, which display this anatomy with both self-avoidance and self/nonself discrimination mediated by proteins called gamma-protocadherins.
FIGURE CREDIT: GRAPHICS COURTESY OF JESSE HAMMER
Alternatively, the branches of an individual cell could continue to repel each other, but the repulsion between the branches of different cells could be replaced with indifference. This would lead to some overlap between the different cells, but not the formation of connections between them (Figure 1B). In a third arrangement, the branches of a cell could both overlap with and readily bind to the branches of a neighboring cell (Figure 1C). This would require cells to display two phenomena: self-avoidance at the level of individual cells, and the ability to recognize branches belonging to neighboring cells. This is called ‘self/nonself discrimination’. Kostadinov and Sanes studied neurons called starburst amacrine cells (SACs) that display this type of self-avoidance with self/nonself discrimination.
These two phenomena require a diverse code of molecules that distinctly label each cell. Sanes and colleagues had previously demonstrated that proteins called gamma-protocadherins provide this code for SACs (Lefebvre et al., 2012). By combining different versions (or isoforms) of the protein produced from the gamma-protocadherin gene, cells can make thousand of different adhesive labels, and each label only tends to recognize and interact with other labels made from the same isoforms (Schreiner and Weiner, 2010). Molecules called Dscams perform a similar role in Drosophila (Zipursky and Grueber, 2013).
Now, Kostadinov and Sanes show the importance of self-avoidance and self/nonself discrimination in the development of neural circuits. First, they either eliminated the expression of all gamma-protocadherins, or allowed the expression of only a single working isoform. In the absence of all gamma-protocadherins, individual SACs began to form synapses with themselves, and formed fewer synapses with neighboring SACs than those in wild type retinas. Furthermore, Kostandinov and Sanes discovered that while many of the synapses that form between SACs are actually eliminated during the normal development of the retina, this loss is reduced if gamma-protocadherins are missing. Finally, and perhaps most significantly, the retinal circuitry failed to work properly because of the anatomical changes caused by the elimination of the gamma-protocadherins.
SACs contribute to a phenomenon called ‘direction selectivity’ that involves neurons in the retina only firing when they detect an object moving in a specific direction. The degree of direction selectivity in the cells that receive signals from the SACs was severely reduced when the SACs lacked gamma-protocadherins.
Direction selectivity was also reduced when SACs expressed a single gamma-protocadherin isoform. However, in this case, individual cells still exhibited self-avoidance and formed branched nerve endings. However, self/nonself discrimination was perturbed, which adversely affected the connections with neighboring SACs. As such, the loss of all gamma-protocadherins had a slightly different effect compared to the loss of all but one gamma-protocadherin. This likely reflects the fact that SACs with a single gamma-protocadherin look more like wild type cells than cells with none.
Kostandinov and Sanes also found that either eliminating all gamma-protocadherins, or all but one, did not affect the connections between the SACs and other cell types (which either send signals to, or receive signals from, the SACs). Therefore, the differences in direction selectivity reflect problems within the SAC network itself. Thus, these findings offer a clear and comprehensive characterization of the consequences of losing self-avoidance and self/nonself discrimination, in terms of both how the SACs connect with other cells, and how this affects the way in which they operate.
While the importance of self-avoidance and self/nonself discrimination in SACs is clear, many questions remain. First, how many cell types use gamma-protocadherins in this way? It has been suggested that cerebellar Purkinje cells might do so too (Lefebvre et al., 2012), but both SACs and Purkinje cells are rather specialized cells and may not represent a typical neuron. Furthermore, several other proteins can act as cues to promote self-avoidance and control the shaping of neural circuits (Fuerst et al., 2008; Matsuoka et al., 2012); could these molecules also be involved and could the same molecules have different roles in specific cell types? Finally, how do ‘adhesion molecules’ recognize each other in a way that, depending on the context, sometimes results in avoidance and not adhesion (Yamagata and Sanes, 2008; Schreiner and Weiner, 2010)? These and other questions have now become all the more important for understanding how the formation of neural circuits is optimized.
References
-
Combinatorial homophilic interaction between gamma-protocadherin multimers greatly expands the molecular diversity of cell adhesionProceedings of the National Academy of Sciences of USA 107:14893–14898.https://doi.org/10.1073/pnas.1004526107
-
Chemoaffinity in the orderly growth of nerve fiber patterns and connectionsProceedings of the National Academy of Sciences of USA 50:703–710.https://doi.org/10.1073/pnas.50.4.703
-
The molecular basis of self-avoidanceAnnual Review of Neuroscience 36:547–568.https://doi.org/10.1146/annurev-neuro-062111-150414
Article and author information
Author details
Publication history
Copyright
© 2015, Garrett and Burgess
This article is distributed under the terms of the Creative Commons Attribution License, which permits unrestricted use and redistribution provided that the original author and source are credited.
Metrics
-
- 1,302
- views
-
- 158
- downloads
-
- 0
- citations
Views, downloads and citations are aggregated across all versions of this paper published by eLife.
Download links
Downloads (link to download the article as PDF)
Open citations (links to open the citations from this article in various online reference manager services)
Cite this article (links to download the citations from this article in formats compatible with various reference manager tools)
Further reading
-
- Chromosomes and Gene Expression
- Developmental Biology
Differentiation of female germline stem cells into a mature oocyte includes the expression of RNAs and proteins that drive early embryonic development in Drosophila. We have little insight into what activates the expression of these maternal factors. One candidate is the zinc-finger protein OVO. OVO is required for female germline viability and has been shown to positively regulate its own expression, as well as a downstream target, ovarian tumor, by binding to the transcriptional start site (TSS). To find additional OVO targets in the female germline and further elucidate OVO’s role in oocyte development, we performed ChIP-seq to determine genome-wide OVO occupancy, as well as RNA-seq comparing hypomorphic and wild type rescue ovo alleles. OVO preferentially binds in close proximity to target TSSs genome-wide, is associated with open chromatin, transcriptionally active histone marks, and OVO-dependent expression. Motif enrichment analysis on OVO ChIP peaks identified a 5’-TAACNGT-3’ OVO DNA binding motif spatially enriched near TSSs. However, the OVO DNA binding motif does not exhibit precise motif spacing relative to the TSS characteristic of RNA polymerase II complex binding core promoter elements. Integrated genomics analysis showed that 525 genes that are bound and increase in expression downstream of OVO are known to be essential maternally expressed genes. These include genes involved in anterior/posterior/germ plasm specification (bcd, exu, swa, osk, nos, aub, pgc, gcl), egg activation (png, plu, gnu, wisp, C(3)g, mtrm), translational regulation (cup, orb, bru1, me31B), and vitelline membrane formation (fs(1)N, fs(1)M3, clos). This suggests that OVO is a master transcriptional regulator of oocyte development and is responsible for the expression of structural components of the egg as well as maternally provided RNAs that are required for early embryonic development.
-
- Developmental Biology
Over the past several decades, a trend toward delayed childbirth has led to increases in parental age at the time of conception. Sperm epigenome undergoes age-dependent changes increasing risks of adverse conditions in offspring conceived by fathers of advanced age. The mechanism(s) linking paternal age with epigenetic changes in sperm remain unknown. The sperm epigenome is shaped in a compartment protected by the blood-testes barrier (BTB) known to deteriorate with age. Permeability of the BTB is regulated by the balance of two mTOR complexes in Sertoli cells where mTOR complex 1 (mTORC1) promotes the opening of the BTB and mTOR complex 2 (mTORC2) promotes its integrity. We hypothesized that this balance is also responsible for age-dependent changes in the sperm epigenome. To test this hypothesis, we analyzed reproductive outcomes, including sperm DNA methylation in transgenic mice with Sertoli cell-specific suppression of mTORC1 (Rptor KO) or mTORC2 (Rictor KO). mTORC2 suppression accelerated aging of the sperm DNA methylome and resulted in a reproductive phenotype concordant with older age, including decreased testes weight and sperm counts, and increased percent of morphologically abnormal spermatozoa and mitochondrial DNA copy number. Suppression of mTORC1 resulted in the shift of DNA methylome in sperm opposite to the shift associated with physiological aging – sperm DNA methylome rejuvenation and mild changes in sperm parameters. These results demonstrate for the first time that the balance of mTOR complexes in Sertoli cells regulates the rate of sperm epigenetic aging. Thus, mTOR pathway in Sertoli cells may be used as a novel target of therapeutic interventions to rejuvenate the sperm epigenome in advanced-age fathers.