Timing mechanism of sexually dimorphic nervous system differentiation
Abstract
The molecular mechanisms that control the timing of sexual differentiation in the brain are poorly understood. We found that the timing of sexually dimorphic differentiation of postmitotic, sex-shared neurons in the nervous system of the Caenorhabditis elegans male is controlled by the temporally regulated miRNA let-7 and its target lin-41, a translational regulator. lin-41 acts through lin-29a, an isoform of a conserved Zn finger transcription factor, expressed in a subset of sex-shared neurons only in the male. Ectopic lin-29a is sufficient to impose male-specific features at earlier stages of development and in the opposite sex. The temporal, sexual and spatial specificity of lin-29a expression is controlled intersectionally through the lin-28/let-7/lin-41 heterochronic pathway, sex chromosome configuration and neuron-type-specific terminal selector transcription factors. Two Doublesex-like transcription factors represent additional sex- and neuron-type specific targets of LIN-41 and are regulated in a similar intersectional manner.
https://doi.org/10.7554/eLife.42078.001eLife digest
In most adult animals, male and female brains are slightly different. For example, in the nematode worm Caenorhabditis elegans, certain neurons exist in one sex but not the other. Nerve cells that are shared in both sexes may also activate different genes, or form different connections in males and females. Most of these differences – which ultimately give rise to sex-specific behaviors – emerge during a period of development called sexual maturation. Yet, the mechanisms that control when sexual differentiation takes place in the brain are largely unknown.
To investigate this, Pereira et al. set out to determine how sex differences arise in the nervous system of C. elegans, a small animal with two sexes, male and hermaphrodite. In particular, Pereira et al. wanted to know which genes cause certain neurons that are present in both sexes to switch to the male-specific form when the worm gets old enough.
The experiments revealed that a genetic pathway formed of three genes, let-7, lin-28 and lin-41, controls when sexual maturation takes place throughout the worm nervous system. When the worm is young, lin-41 is active and represses a gene called lin-29A. As the animal reaches maturity, let-7 ‘switches off’ lin-41, and lin-29A gets activated in a subset of neurons. These brain cells then turn on male-specific genes and acquire a shape only found in males. The anatomy of male mutant worms that lack lin-29A is normal, but the animals show features found in hermaphrodites, for example in the way they crawl across a dish. This shows that activating lin-29A may also trigger male-specific behaviors.
Switching on sex-specific neuronal circuits at the correct time is essential for animals to develop correctly. The lin-7 and let-28 genes also control when sexual maturation takes place in mammals, so studying these genes in C. elegans could help to understand how male and female brains are shaped during development in other species, and why some diseases affect the sexes differently.
https://doi.org/10.7554/eLife.42078.002Introduction
The nervous systems of males and females of many animal species display anatomical and functional differences (Jazin and Cahill, 2010; McCarthy and Arnold, 2011; Portman, 2017; Yang and Shah, 2014). Sex-specific maturation events in the brain are typically triggered at a specific window of postnatal life. Sexual maturation in mammals, called puberty, represents a key developmental transition and is accompanied by substantial sexually dimorphic changes in the brain (Avendaño et al., 2017; Juraska and Willing, 2017; Sisk and Foster, 2004). The onset of puberty in mammals is characterized by the activation of neurons in the hypothalamus that produce hormonal signals (Avendaño et al., 2017). However, the mechanisms that control the activation of these neurons and hence the timing of pubertal onset are poorly understood.
Like in other animals, most of the sex-specific features of the nervous system of the nematode Caenorhabditis elegans are also established during postembryonic development (Barr et al., 2018; Sulston et al., 1980; Sulston and Horvitz, 1977). Sexual dimorphisms in the C. elegans nervous system fall into two broad types: (1) Some neurons are present only in one sex but not the opposite sex (Sulston et al., 1980; Sulston et al., 1983). These sex-specific neurons are directly involved in distinct aspects of sex-specific mating and reproductive behavior (Emmons, 2018). (2) Some embryonically born, postmitotic neurons that are present in both sexes (‘sex-shared’ neurons) can adopt sex-specific features during sexual maturation (Portman, 2017). Sexually dimorphic features of sex-shared neurons include sex-specific synaptic wiring patterns (Jarrell et al., 2012) and sex-specific expression of signaling molecules, such as neurotransmitters, neuropeptides and chemoreceptors (Hilbert and Kim, 2017; Pereira et al., 2015; Ryan et al., 2014; Serrano-Saiz et al., 2017a; Serrano-Saiz et al., 2017b). For example, the AIM interneuron class is glutamatergic in juvenile, pre-sexual maturation stages of both sexes, but during sexual maturation will turn off glutamatergic identity and turn on cholinergic neurotransmitter identity specifically in males (Pereira et al., 2015). In other cases, sexually dimorphic features emerge from a ‘mixed’ juvenile state. For example, juvenile phasmid sensory neurons innervate several distinct interneuron targets in both sexes before sexual maturation, but during sexual maturation specific subsets of these connections get pruned in a sex-specific manner (Oren-Suissa et al., 2016).
Most if not all of these sexually dimorphic features of the C. elegans nervous system terminally differentiate during the fourth larval stage (Desai et al., 1988; Oren-Suissa et al., 2016; Pereira et al., 2015; Serrano-Saiz et al., 2017a; Sulston and Horvitz, 1977). The molecular mechanisms that control the timing of sexual maturation in postmitotic neurons have largely remained obscure. Candidate regulators of sex-specific developmental timing events are so-called heterochronic genes, first identified in C. elegans (Ambros and Horvitz, 1984). Heterochronies refer to offsets of the relative timing of somatic developmental events versus gonadal developmental events (Slack and Ruvkun, 1997). In heterochronic mutants, the timing of cellular cleavage patterns in postembryonic skin cell lineages is disrupted in a highly stereotyped manner; in some mutants, lineage patterning events normally occurring late will occur earlier (‘precocious mutants’) while in other mutants specific cell lineage patterning events will be delayed or never occur (Ambros, 1989; Ambros and Horvitz, 1984; Slack and Ruvkun, 1997). Molecular analysis of these heterochronic mutants revealed a gene regulatory pathway, which contains several deeply conserved post-transcriptional regulatory factors at its core, including the miRNA let-7, the LIN-28 protein, a negative regulator of let-7 processing, and the RNA-binding, posttranscriptional regulator LIN-41, a key target of the let-7 miRNA (Figure 1A) (Ambros, 1989; Ecsedi et al., 2015; Moss et al., 1997; Reinhart et al., 2000; Slack et al., 2000). While these regulatory factors are well known to control cell division patterns in the ectoderm, their role in postmitotic cell types is much less well defined (Del Rio-Albrechtsen et al., 2006; Hallam and Jin, 1998; Howell et al., 2015; Olsson-Carter and Slack, 2010; Zou et al., 2013).
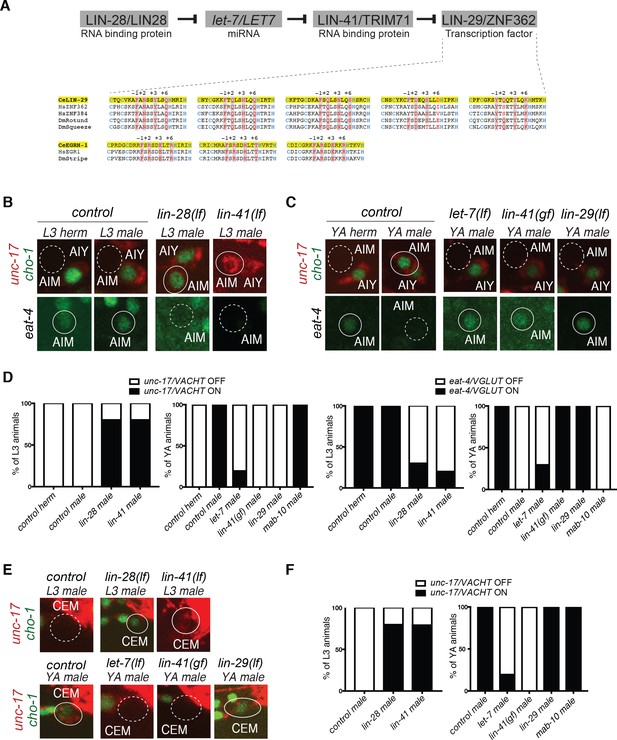
Heterochronic pathway effects on AIM and CEM neuronal differentiation during sexual maturation.
(A) A simplified schematic of the heterochronic pathway, deduced from the genetic analysis of hypodermal cell lineage division events. The LIN-28 RNA binding protein prevents the maturation of miRNA let-7. When LIN-28 protein levels go down at the end of the second larval stage (L2), mature miRNA let-7 levels rise and bind to the 3’UTR of let-7 targets. One let-7 target is the RNA-binding protein LIN-41, whose levels are downregulated at the onset of sexual maturation (L4). This allows for its negative regulator, the zinc-finger transcription factor lin-29, to be expressed at the L4 stage to induce the larval to adult transition. Sequence homology alignment of the five Zn-finger domains shows that LIN-29 is an orthologue of human ZNF362 and ZNF384, while EGRH-1 is an orthologue of human EGR. The Zn fingers of worm, fly and human are shown. Zn coordinating Cys and His residues are colored in blue and DNA-contacting residues (at position −1,+2,+3 and+6 of the helixes preceding the His residues) are colored in red. Conservation is indicated in grey shading. (B) AIM neuronal differentiation is precocious in lin-28(n719lf) and lin-41(bch28lf). unc-17/VACHT (ot907) and cho-1/CHT (otIs534) cholinergic reporter expression (cytoplasmic for unc-17 and nuclear for cho-1) is not observed in AIM in control animals at the L3 stage (dotted circles, top panels). lin-28(n719lf) and lin-41(bch28lf) mutant males show precocious cholinergic gene expression in AIM at the L3 stage (top panels). eat-4/VGLUT (otIs388) glutamatergic reporter expression is observed in AIM in control animals at the L3 stage (circled in white, bottom panels). lin-28(n719lf) and lin-41(bch28lf) mutant males show precocious loss of AIM glutamatergic identity (dotted circles, bottom panels). The AIY cholinergic neuron is located next to AIM and is used as a positional reference. Solid circles indicate expression of the reporter, stippled circle indicates loss of expression. (C) Male-specific AIM differentiation is blocked in let-7(n2853ts), lin-41(xe8gf) and lin-29a/b(n333lf) mutants. unc-17/VACHT (ot907) and cho-1/CHT (otIs354) cholinergic reporter expression (cytoplasmic for unc-17 and nuclear for cho-1) is observed in young adult (YA) control males but not in AIM neurons in hermaphrodites nor let-7(n2853ts), lin-41(xe8gf) and lin-29a/b(n333lf) mutant males (dotted circles, top panels). eat-4/VGLUT (otIs388) glutamatergic reporter expression is observed in hermaphrodites and in let-7(n2853ts), lin-41(xe8gf) and lin-29a/b(n333lf) mutant males, where it fails to be downregulated (circled in white, bottom panels). L1 let-7(n2853ts) animals were shifted to the restrictive temperature (25°C) and imaged after 48hs. The incomplete penetrance of the let-7 mutants is likely because the allele used, n2853, a point mutation in the miRNA seed region, is hypomorphic. The AIY cholinergic neuron is located next to AIM and is used as a positional reference. (D) Quantification for the AIM neurotransmitter switch in heterochronic mutants lin-28(n719lf), lin-41(bch28lf), let-7(n2853ts), lin-41(xe8gf), lin-29a/b(n333lf) and mab-10(xe44) (n = 15). Expression of unc-17/VACHT (ot907) and eat-4/VGLUT (otIs388) was quantified as ON or OFF. (E) CEM neuronal differentiation defects in heterochronic mutants. CEM cholinergic gene expression is precocious in lin-28(n719lf) and lin-41(bch28lf) males compared to control. unc-17/VACHT (ot907) and cho-1/CHT (otIs354) cholinergic reporter expression is not observed in CEM neurons in wild-type males at the L3 stage (dotted circle). lin-28(n719lf) and lin-41(bch28lf) males show precocious cholinergic gene expression in CEM neurons at the L3 stage (circled in white, top panels). CEM cholinergic gene expression is lost in let-7(n2853ts) and lin-41(xe8gf) mutants (dotted circles). lin-29a/b(n333lf) mutants showed no defect in cholinergic gene expression in CEM neurons (circled in white). L1 let-7(n2853ts) animals were shifted to the restrictive temperature (25°C) and imaged after 48hs. The incomplete penetrance of the let-7 mutants is likely because the allele used, n2853, a point mutation in the miRNA seed region, is hypomorphic. (F) Quantification for CEM cholinergic gene expression in heterochronic mutants lin-28(n719lf), lin-41(bch28lf), let-7(n2853ts), lin-41(xe8gf), lin-29a/b(n333lf) and mab-10(xe44) (n = 15). Expression of unc-17/VACHT (ot907) was quantified as ON or OFF.
Within the nervous system, the impact of heterochronic genes on sex-specific differentiation events during sexual maturation at the juvenile to adult transition has been poorly defined. Such a role is not an obvious matter because heterochronic genes do not control timing in all tissues. For example, heterochronic genes are not involved in controlling the timing of gonadal maturation (Ambros and Horvitz, 1984; Euling and Ambros, 1996). We show here that the phylogenetically conserved core heterochronic pathway genes lin-28, let-7 and lin-41 control the timing of maturation of sexual dimorphisms in different neuron classes. We show that these broadly expressed timing genes act through distinct cell-specific effector modules, among them a specific isoform of the lin-29 Zn finger transcription factor and the two DM domain (DMD) containing transcription factors mab-3 and dmd-3. These temporally regulated effector genes are expressed in a sexually dimorphic manner in distinct sets of sex-shared neurons to control specific aspects of sexually dimorphic features and functions. Remarkably, genome-wide association studies have linked one component of the heterochronic pathway to aberrant onset of puberty in humans (Faunes and Larraín, 2016). Our findings therefore suggest the emergence of a phylogenetically conserved mechanism to control the timing of sexual maturation.
Results
Effects of the heterochronic lin-28/let-7/lin-41 pathway on neuron-specific sexual maturation
As an entry point to identify the molecular mechanisms required for the correct timing of nervous system sexual maturation, we used the previously described neurotransmitter switch of the AIM interneurons (Figure 1B–D), a sex-shared neuron class that is required for mate searching behavior (Barrios et al., 2012; Pereira et al., 2015). A fosmid-based reporter of the eat-4/VGLUT vesicular glutamate transporter is expressed during juvenile stages in the AIM interneuron of both sexes. At the onset of sexual maturation, during the fourth larval stage, AIM neurons of the male turn off the glutamatergic gene reporter and turn on expression of fosmid-based reporters for the cholinergic genes unc-17/VACHT and cho-1/CHT (Pereira et al., 2015). The acquisition of AIM cholinergic identity during sexual maturation only in males was confirmed by analyzing the expression of the cholinergic unc-17/VACHT locus, tagged with mKate2 using CRISPR/Cas9 genome engineering (Figure 1—figure supplement 1).
Sexual maturation in many animal species is controlled by gonadal hormones (Avendaño et al., 2017). In C. elegans, the gonad has been shown to be required for the establishment of sex-specific adult behaviors (Arantes-Oliveira et al., 2002; Fujiwara et al., 2016; Kleemann et al., 2008; Lipton et al., 2004). Therefore, we first examined whether signals from the germline or gonad may affect the AIM neurotransmitter switch. We found that neither genetic removal of the germ cells nor laser ablation of gonadal precursor cells (which results in the loss of both gonad and germline) affects the execution of the AIM neurotransmitter switch (Figure 1—figure supplement 2).
Temporal control of cell lineage decisions during early larval stages in C. elegans has been shown to depend on the activity of the evolutionary conserved heterochronic pathway (Ambros and Horvitz, 1984; Moss and Romer-Seibert, 2014; Slack and Ruvkun, 1997). To test if members of the heterochronic pathway were required for the correct timing of nervous system differentiation during sexual maturation, we examined the impact of heterochronic mutants on the male-specific AIM neurotransmitter switch. We observed no defects in the timing of cholinergic gene battery induction in L4 in lin-4 null mutant animals which controls the timing of L2-specific aspects of cellular patterning (Ambros and Horvitz, 1984) (data not shown). However, we observed a premature onset of both unc-17/VACHT and cho-1/CHT expressions in AIM neurons of lin-28 mutant males (Figure 1B,D), mirroring the precocious cellular cleavage defects of lin-28 in epidermal tissues (Ambros and Horvitz, 1984). Matching the precocious induction of cholinergic identity, lin-28 mutant males also showed a precocious downregulation of the glutamatergic marker eat-4/VGLUT in the AIM interneurons (Figure 1B,D).
We also found that in males carrying a loss-of-function mutation in the miRNA let-7, whose processing is negatively controlled by lin-28 (Lehrbach et al., 2009), the neurotransmitter switch of the AIM interneurons is eliminated, that is, male AIM neurons remain glutamatergic (Figure 1C–D). Since let-7 is a repressor of gene expression, the loss of the neurotransmitter switch suggests that let-7 may normally act to repress a factor that prevents the neurotransmitter switch. We found that lin-41, the first of many let-7 targets to be identified (Slack et al., 2000) encodes this repressor factor. In lin-41 loss-of-function mutants (bch28), the neurotransmitter switch was prematurely executed, while in lin-41 gain-of-function mutants (xe8), in which let-7 is unable to downregulate lin-41 activity due to a deletion of the let-7-binding sites in the lin-41 3’UTR (Ecsedi et al., 2015), the neurotransmitter switch of AIM was abrogated and AIM neurons remained glutamatergic (Figure 1B,D).
The heterochronic pathway genes also controlled the onset of differentiation of the male-specific CEM sensory neurons. Even though these neurons are already born in the embryo (and removed by apoptosis in hermaphrodites [Sulston et al., 1983]), the CEM neurons only fully differentiate at the fourth larval stage (Pereira et al., 2015). By analyzing the expression of cholinergic reporters, we found that this L4-specific maturation event is also precisely timed by the heterochronic pathway genes. In lin-28 mutants, CEM neurons prematurely expressed cholinergic genes while in let-7 mutants CEM neurons’ terminal differentiation was blocked (Figure 1E,F). As we had seen for the AIM neurotransmitter switch, lin-41 mutants showed precocious cholinergic gene expression in CEM neurons and lin-41 gain-of-function mutants (in which lin-41 expression fails to be downregulated) also fail to show CEM cholinergic gene expression during sexual maturation suggesting that let-7 miRNA acts through regulating lin-41 for the correct timing of this process (Figure 1E,F). However, as in the case of the AIM neurotransmitter switch, lin-4 mutants show no defect in the timing of CEM differentiation (data not shown). In conclusion, mutant analysis of the heterochronic pathway genes showed that the lin-28/let-7/lin-41 regulatory pathway is required for the correct timing and for the acquisition of male-specific features of two distinct neuron classes; however, this regulatory cassette may couple to upstream regulatory inputs other than lin-4.
The lin-29 Zn finger transcription factor is the relevant target of lin-41 in controlling the AIM neurotransmitter switch
lin-41 encodes a versatile regulatory protein with RING and NHL domains, involved in both post-transcriptional RNA regulation (including translation) and protein ubiquitination (Ecsedi and Großhans, 2013). To ask which of the many previously described effectors of LIN-41 (Aeschimann et al., 2017; Ecsedi and Großhans, 2013) are relevant in the context of the AIM neurotransmitter switch, we turned to the lin-29 Zn finger transcription factor, whose expression is translationally inhibited by lin-41 in the context of timing of skin cell proliferation (Aeschimann et al., 2017; Ambros and Horvitz, 1984; Rougvie and Ambros, 1995; Slack et al., 2000)(Figure 1A). Orthology analysis with several tools (Kim et al., 2018; Wang et al., 2017) as well as a detailed analysis of predicted DNA binding features suggests that LIN-29 is an ortholog of the uncharacterized vertebrate ZNF362 and ZNF384 proteins (Figure 1A). During the larva-to-adult transition, let-7 inhibition of lin-41 derepresses lin-29 expression and induces adult gene expression programs in the epidermis. Consistent with lin-29 being a functional effector of lin-41 in the AIM interneurons, we find that the AIM neurotransmitter switch is entirely lost in lin-29 null mutants (Figure 1C,D). In contrast, we found that the onset of differentiation of the male-specific CEM neurons in the L4 stage, as measured by the induction of cholinergic identity, is unaffected in lin-29 null mutants (Figure 1E,F). These findings indicate that lin-41 controls distinct effector genes in different neuron types.
The transcriptional co-factor mab-10/NAB has been shown to act with lin-29 to control epidermal cell differentiation during the larval to adult transition (Harris and Horvitz, 2011). Moreover, biochemical studies revealed that the mab-10 transcript, like lin-29, is a direct target of the LIN-41 protein (Aeschimann et al., 2017). We confirmed this notion by CRISPR/Cas9-mediated tagging of the mab-10 locus with mCherry, finding that ubiquitous mab-10 expression initiates at the L4 stage (Figure 2A). However, analyzing the effects of a newly generated mab-10 null allele, we found that in the absence of mab-10, the AIM neurotransmitter switch and CEM cholinergic gene expression occurred normally (Figure 1D,F). Hence, while mab-10 functions with lin-29 in seam cell differentiation, it is not required for lin-29 function in AIM interneurons in males.
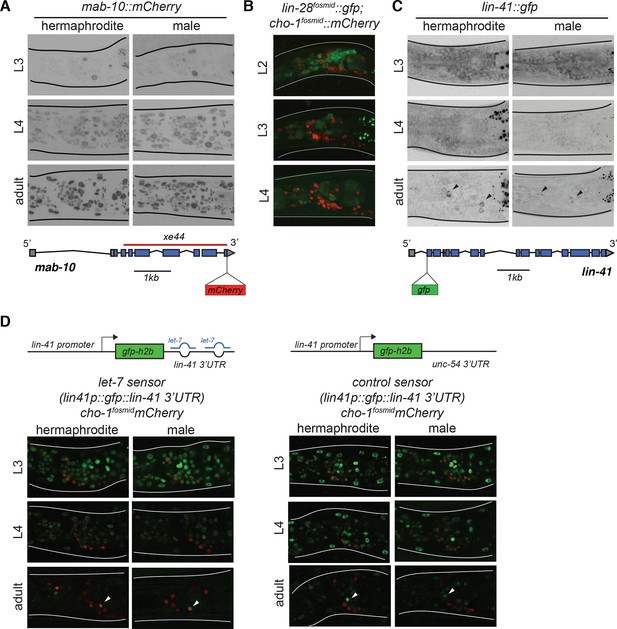
Temporal expression pattern of heterochronic pathway genes in the nervous system.
(A) mab-10::mCherry expression pattern during larval development in both sexes. An endogenously tagged mab-10 allele (xe75) showed ubiquitous expression starting in the L4 stage, including in the nervous system. Reporter expression was maintained in the adult. No sexually dimorphic differences were observed for mab-10 expression. A cartoon for the mab-10 locus is shown below the images. (B) lin-28::gfp expression pattern during early larval development. A lin-28::gfp fosmid-based reporter (wgIs535) showed ubiquitous expression at the L2 stage, including in the nervous system. Reporter expression was broadly downregulated at the L3 and L4 stages. The fosmid-based cho-1/CHT reporter (otIs544) was used to label cholinergic neurons. No sexually dimorphic differences were observed for lin-28 expression. (C) lin-41 expression pattern during larval development in both sexes. An endogenously tagged lin-41 allele (tn1541) showed ubiquitous expression at the L3 stage, including in the nervous system. Reporter expression is broadly downregulated at the L4 stage. Expression of lin-41::gfp was maintained in two neuronal pairs in the head (black arrows). No sexually dimorphic differences were observed for lin-41 expression. A cartoon for the lin-41 locus is shown below the images. (D) let-7 activity sensor and control sensor expression during larval development in both sexes. A lin-41 promoter fusion driving gfp and fused to the lin-41 3’UTR (xeSi182), which is directly targeted by let-7 miRNA, showed ubiquitous expression of gfp in the nervous system from the L1 to the L3 stage. At the L4 stage, reporter expression is broadly downregulated and only a few cells are labeled by GFP in the adult head, recapitulating the lin-41::gfp expression. The same lin-41 promoter fusion driving gfp and fused to the unc-54 3’ UTR (xeSi202) failed to be normally downregulated at the L4 stage showing that lin-41 downregulation requires its 3’UTR being bound by miRNA let-7. Cholinergic neurons were labeled by a cho-1/CHT fosmid reporter (otIs544) driving mCherry allowing us to correlate the lin-41 downregulation with AIM neurotransmitter switch in males at the L4 stage. No sexually dimorphic differences in gfp expression were observed for the let-7 sensor and its control. Cartoons for each construct are shown above the images.
Transition from ubiquitous temporal regulators (lin-28/let-7/lin-41) to a sex- and neuron-type specific regulator (lin-29)
To address in which cells the heterochronic pathway acts to control the AIM neurotransmitter switch, we first examined the expression pattern of lin-28, let-7, lin-41 and lin-29 in the nervous system. While expression of these genes in other cells, specifically, the epidermis, has previously been examined in detail (Moss et al., 1997; Rougvie, 2001; Slack et al., 2000), much less is known about their neuronal expression. We analyzed heterochronic gene expression relative to either a panneuronally expressed RFP marker or a cholinergic RFP marker (cholinergic labeling of the AIM neuron allowed us to correlate the timing of heterochronic gene expression relative to the AIM neurotransmitter switch). Consistent with previous reporter gene analysis (Moss et al., 1997), we found that a fosmid-based reporter construct of the lin-28 gene is expressed very broadly in the nervous system in a sexually non-dimorphic manner and downregulated by the end of the L2 stage (Figure 2B).
To analyze lin-41 expression, we used an endogenously gfp tagged lin-41 allele (Spike et al., 2014). This lin-41 reporter allele showed broad expression in the nervous system from the L1 until the L4 stage in both sexes, when it becomes downregulated (Figure 2C). Previous work showed that lin-41 downregulation in hypodermal tissue depends on let-7 (Ecsedi et al., 2015; Slack et al., 2000) and to corroborate this notion in the context of the nervous system, we analyzed let-7 activity using an ‘activity sensor’ construct in which the lin-41 promoter is fused to gfp and to the let-7 responsive 3’UTR of the lin-41 locus. This reporter shows ubiquitous expression, including in the nervous system, from the L1 stage to L4, when GFP levels start to drop and disappear from most tissues, including neurons, by the adult stage (Figure 2D). A control construct carrying the unc-54 3’ UTR is not downregulated at the L4 and young adult stages (Figure 2D). We conclude that let-7 activity is, as expected, temporally controlled in the nervous system. We did not observe any sexual dimorphisms of lin-41 or let-7 sensor expression in the nervous system.
To determine the expression pattern of the lin-41 effector lin-29 in the nervous system, we used strains in which the lin-29 locus was tagged with gfp, using CRISPR/Cas9-mediated genome engineering. lin-29 produces two distinct transcripts, lin-29a and lin-29b that differ at their 5’end; both contain five DNA-binding Zn finger domains (Rougvie and Ambros, 1995) (Figure 3A). We used a strain in which both isoforms were simultaneously tagged by introducing gfp at the 3’ end of the locus (Aeschimann et al., 2017) (Figure 3A). In addition, we examined the expression pattern exclusively of the b-isoform, by introducing the same 3’ terminal gfp tag in the a-isoform-specific mutant allele (xe40) via CRISPR/Cas9-mediate genome engineering (Figure 3A). To examine the expression pattern of the a-isoform, we inserted gfp at the 5’ end of the a-isoform (Figure 3A). Generally, the expression pattern of the gfp allele that tags both isoforms matches the expression pattern reported using an antibody directed against both LIN-29 isoforms (Figure 3B) (Bettinger et al., 1996; Euling et al., 1999). As previously reported, we observed expression in head neurons and the ventral nerve cord. However, we found that this neuronal expression is almost completely restricted to the male and this male-specific expression can be entirely ascribed to the a-isoform (Figure 3B–D). The male-specific neuronal expression of lin-29a is not observed in male-specific neurons but is, interestingly, entirely restricted to sex-shared neurons (i.e. neurons that are generated in both sexes). Intriguingly, most of these neurons had not been previously reported to show sexually dimorphic gene expression patterns or functions. Specifically, 21 out of 116 sex-shared neuron classes express lin-29a, including seven sensory neuron classes in the head and tail (AWA, ASG, ASJ, ASK, ADF, PHB, PLM), seven interneuron classes (AVA, RIA, AIM, AVG, RIF, PVC, PVN), a few head and tail motor neurons (SAB, PDA, PDB) and most of the sex-shared ventral nerve cord motor neurons (dorsal and ventral A, B and D-type and AS neurons). Many of these LIN-29A expressing neurons are synaptically connected to one another (White et al., 1986) and some of the synaptic connections are known to be sexually dimorphic (Jarrell et al., 2012).
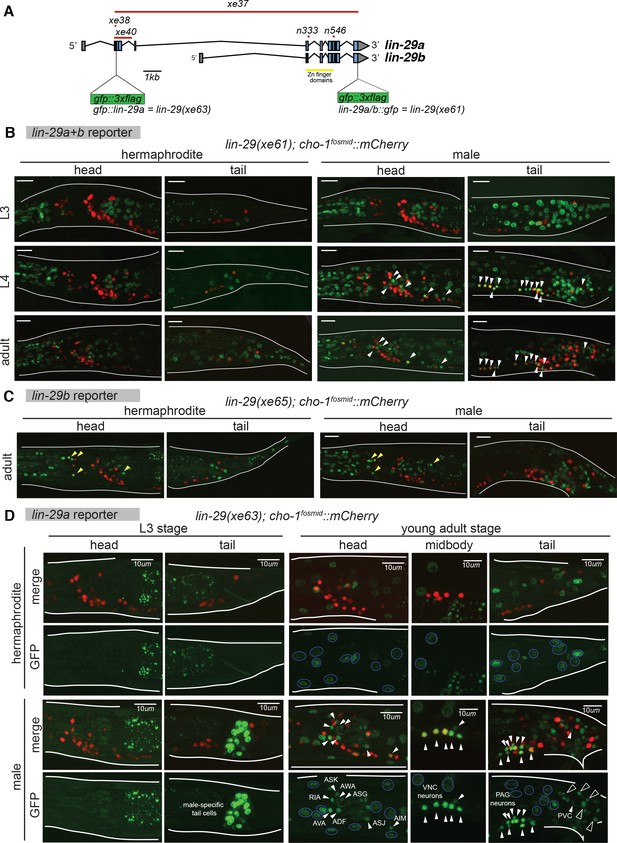
Temporal expression pattern of lin-29 in the nervous system of both sexes.
(A) Cartoon showing the lin-29 locus. Alternative promoter usage generates two LIN-29 protein isoforms. Exons 1 – 4 are LIN-29A specific while exons 5 – 11, that include the Zn-finger DNA binding domain, are shared by both -A and -B isoforms. The lin-29 locus was tagged using CRISPR/Cas9 genome engineering. gfp was inserted either at the C-terminal end (lin-29a/b::gfp; xe61 allele) to tag both LIN-29A and B protein isoforms or at the N-terminal end (gfp::lin-29a; xe63 allele) to tag only the LIN-29A isoform. Canonical alleles n333 and n546 as well as the newly generated alleles xe37 (null), xe38 (A-specific) and xe40 (A-specific) are indicated. (B) lin-29a/b expression pattern during larval development in both sexes. Confocal images for endogenously tagged lin-29a/b::gfp (xe61) show that GFP is expressed in the pharynx at the L3 stage and onwards in both sexes (examination of young animals showed that pharyngeal expression starts at the L1 stage). Hypodermal GFP expression all along the body started at the end of the L3 stage in both sexes. At the L4 stage, we also detected GFP expression in neurons only in the male, many of which were also labeled by a cholinergic marker, a cho-1/CHT mCherry expressing fosmid (marked by arrows). Expression in these male neurons persisted in the adult stage. No neuronal expression was observed in the head nor tail in hermaphrodite animals. Scale bar: 10 µm. (C) lin-29b expression pattern at the young adult stage in both sexes. Confocal images for gfp tagged lin-29b(xe40) (this allele is given a new name, xe65, since it contains the xe40 lesion plus the gfp insertion) show that GFP is expressed in head glia in both sexes (marked by yellow arrowheads). GFP is also observed in the pharynx and in tail cells in both sexes. Cholinergic cho-1/CHT mCherry expressing fosmid (otIs544) did not co-localize with GFP showing that LIN-29B is not expressed in neurons in the head nor tail in either sex (Note that LIN-29B is expressed in midbody neurons in both sexes not shown in this image). Scale bar: 10 µm. (D) gfp::lin-29a expression pattern during larval development in both sexes. Confocal images for endogenously tagged gfp::lin-29a (xe63) show that GFP is expressed in male-specific non-neuronal tail cells at the end of the L3 stage and onwards. No expression was observed in the head or tail of the hermaphrodite at this stage. No overlap between GFP and the cholinergic fosmid based reporter cho-1/CHT (otIs544) was observed at this stage. At the young adult stage, we observed GFP expression in neurons only in males (expression in male neurons started at the L4 stage), indicated by white arrows. No neuronal expression was observed in hermaphrodite neurons. Many GFP positive neurons in the male were also labeled by the cho-1/CHT mCherry expressing fosmid (otIs544). Cholinergic neurons expressing lin-29a included AIM, ASJ, AVA, ASK, AWA, ASG, RIA and ADF in the head, ventral nerve cord (VNC) motor neurons of the A, B, D and AS classes and the PVC interneuron in the tail. Neuronal GFP expression is indicated by arrows and neuronal identity is indicated for head and tail neurons in the GFP panels. GFP expression was observed throughout the hypodermis in both sexes, indicated with blue circles in the GFP panels. Hypodermis nuclei are larger. Cholinergic retrovesicular ganglion (RVG), ventral nerve cord (VNC) and pre-anal ganglion (PAG) neurons expressing lin-29a are shown in the head, midbody and tail respectively. Male-specific tail cells expressing lin-29a are marked with black arrows. Scale bar: 10 µm.
Expression of lin-29a in all these neurons in the male nervous system is precisely temporally controlled; it is first observed in the early L4 stage and persists throughout adulthood (Figure 3D). In addition to the male-specific neuronal expression of the lin-29a isoform, we also found that the lin-29b isoform is expressed in three postembryonically generated touch neurons (AVM, PVM, PDE), where its expression is not temporally regulated, that is, its expression is induced right after the neurons are born in the first two larval stages and expression is observed in both sexes (data not shown).
The late larval onset of expression of lin-29 in non-neuronal cells has been reported to be controlled by the heterochronic pathway (Bettinger et al., 1996; Moss et al., 1997). To assess whether onset of male-specific, neuronal expression of the lin-29a isoform is controlled by lin-41 and let-7, we analyzed lin-29a neuronal expression in these mutant backgrounds. Indeed, we found that in lin-41(lf) mutants lin-29a::gfp expression is observed prematurely including in the sex-shared neurons of the male (Figure 4A). As expected, in let-7(lf) and lin-41(gf) mutants (both of which maintain expression of LIN-41), lin-29a::gfp expression levels are significantly reduced compared to wild-type adult males (Figure 4B). This decrease in lin-29a expression is consistent with the similarity of loss-of-function phenotypes of let-7 and lin-29 null mutants and lin-41 gain-of-function mutants in which the AIM neurotransmitter switch does not occur (Figure 1). Together with our previous biochemical analysis (Aeschimann et al., 2017), we conclude that let-7-controlled LIN-41 directly represses lin-29a translation to control the precise timing of LIN-29A protein accumulation in the nervous system.
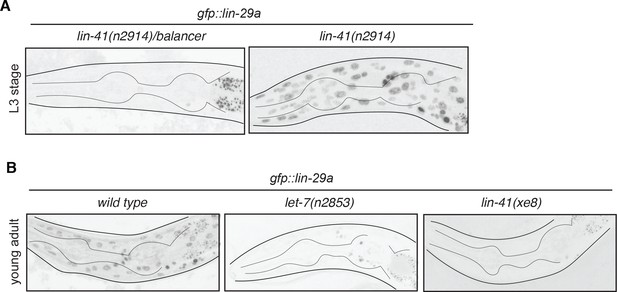
lin-29a neuronal expression is temporally regulated by lin-41 and let-7.
(A) gfp::lin-29a expression in the lin-29a(xe63) strain is precocious in the absence of lin-41. Expression was examined in synchronized L3 animals and compared between lin-41(n2914)/balancer control versus lin-41(n2914) loss-of-function males. Neuronal nuclei are more compact than the larger hypodermal nuclei. (B) gfp::lin-29a expression in the lin-29a(xe63) strain is downregulated in let-7(lf) and lin-41(gf) mutants. Expression was examined at the young adult stage in let-7(n2853ts) loss-of-function mutants and lin-41(xe8) gain-of-function mutants, compared to control males. While control males showed expression of gfp::lin-29a in the hypodermis and nervous system (more compact nuclei), both mutants showed a severe downregulation of gfp::lin-29a expression in the hypodermis and neurons at the young adult stage. L1 let-7(n2853ts) animals were shifted to the restricted temperature (25°C) and imaged as adults, after 48hs.
The lin-29a isoform is required cell-autonomously and is sufficient to define the male-specific neurotransmitter switch in the AIM interneuron
To assess the functional relevance of the lin-29a isoform in controlling the AIM neurotransmitter switch, we generated two lin-29a-isoform specific alleles (xe38 and xe40) using CRISPR/Cas9 genome engineering (Figure 3A) (Aeschimann et al., 2018). We indeed find that in both isoform-specific alleles the male-specific AIM neurotransmitter switch failed to occur (Figure 5A). A newly generated lin-29 null allele that removes both isoforms (Aeschimann et al., 2017) also confirmed the results previously reported with the canonical null alleles tested (Figure 5A).
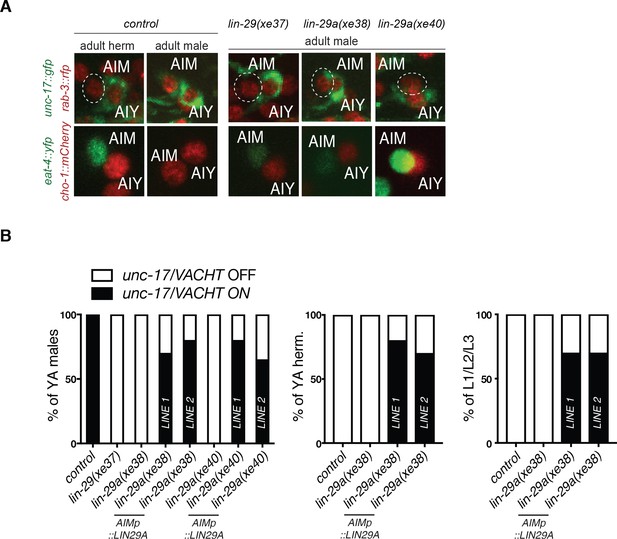
Cell-autonomy and sufficiency of lin-29a for the AIM neurotransmitter switch.
(A) The neurotransmitter switch is blocked in three newly generated lin-29 mutants. In adult control animals, the fosmid-based cholinergic reporters unc-17/VACHT (otIs576) and cho-1/CHT (otIs544) are expressed in AIM neurons of adult males but not hermaphrodites while the eat-4/VGLUT (otIs518) glutamatergic reporter is expressed in AIM neurons of adult hermaphrodites but not males. The AIM neurotransmitter switch is blocked in a newly generated lin-29a/b(xe37) null allele and in two lin-29a-specific mutants (xe38 and xe40). In these lin-29 mutant males, AIM fails to turn on cholinergic markers unc-17/VACHT (top panels) and cho-1/CHT (bottom panels) and expresses a pan neuronal maker rab-3::rfp (top panels) and the glutamatergic marker eat-4/VGLUT (bottom panels). A pan-neuronal rab-3::rfp (otIs355) reporter was used to label all neurons in the top panels. (B) AIM-specific LIN-29A expression is sufficient to cell-autonomously rescue the neurotransmitter switch in males and induce it in young larvae and the opposite sex. LIN-29A expression was driven under an AIM-specific promoter in lin-29a mutant animals: LIN-29A was expressed in lin-29a(xe38) (otEx7316 and otEx7317) and in lin-29a(xe40) (otEx7318 and otEx7319). Expression of cholinergic gene reporter unc-17/VACHT (otIs576) was examined to assay rescue and ectopic induction of the AIM neurotransmitter switch (n = 15).
To assess whether lin-29a acts cell-autonomously in AIM to control its neurotransmitter switch, we expressed lin-29a under an AIM-specific promoter (Serrano-Saiz et al., 2017a). This driver is not only active in both sexes, but it is already active in embryos and early larval stages, hence allowing us to assess three key issues: (1) whether LIN-29A acts cell-autonomously, (2) whether LIN-29A can induce the neurotransmitter switch prematurely in males and (3) whether LIN-29A can ectopically induce the AIM neurotransmitter switch in hermaphrodites. We generated two independent transgenic lines that cell type-specifically express lin-29a in a lin-29a (xe38 and xe40) mutant background. We found that in adult male animals, the loss of the AIM neurotransmitter switch (as assayed by cho-1 and unc-17 induction and eat-4 downregulation in AIM) is rescued by the transgene, thereby demonstrating cell autonomy of LIN-29A function (Figure 5B).
Examining juvenile males, before the onset of overt sexual maturation, we observed that the neurotransmitter switch is executed prematurely already by the first larval stage in these transgenic animals ectopically expressing LIN-29A in AIM neurons (Figure 5B). This sufficiency experiment indicates that the temporal control of lin-29a expression, and specifically the repression of lin-29a by the heterochronic pathway is required to prevent lin-29a from triggering the neurotransmitter switch precociously. Lastly, examining adult hermaphrodite animals expressing this construct, we found that lin-29a is sufficient to induce the neurotransmitter switch in the opposite sex (Figure 5B). We conclude that lin-29a is required and sufficient to control the AIM neurotransmitter switch.
To assess whether lin-29a function in AIM goes beyond controlling AIM’s neurotransmitter switch, we examined another molecular marker of sexually dimorphic AIM differentiation, the orphan GPCR, srj-54, which is induced in the AIM neurons upon male sexual differentiation (Portman, 2007). We found that srj-54 fails to be expressed in lin-29a mutants (Figure 6A) adding another AIM male-specific molecular feature that fails to be normally induced in the absence of lin-29. As we will describe further below, lin-29a mutants are also defective in an AIM-mediated sexual behavioral paradigm.
lin-29a controls sexually dimorphic molecular features of other sex-shared neurons
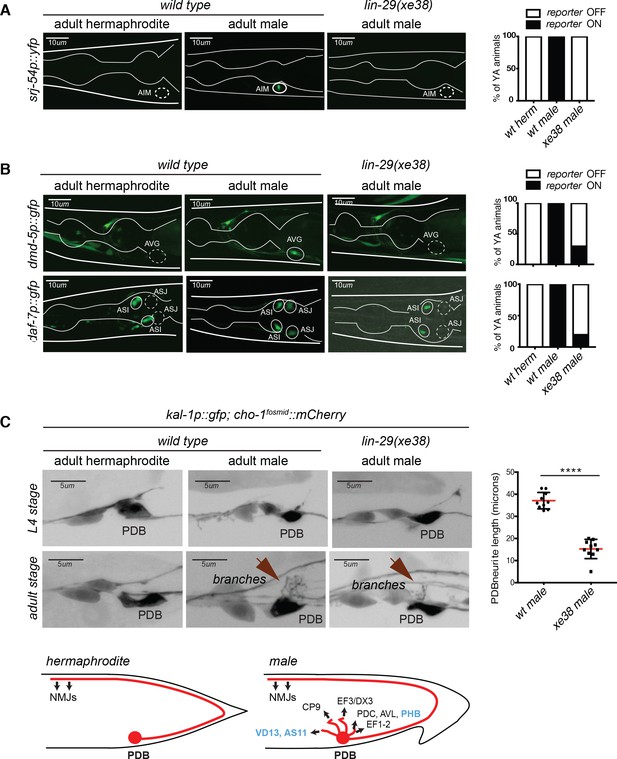
Male-specific molecular differentiation programs are lost in lin-29a mutants.
(A) Male-specific expression of srj-54 in AIM requires lin-29a. Expression of the GPCR srj-54 (fsIs5) promoter fusion is observed in adult males but not hermaphrodites in the AIM interneurons. Expression of srj-54 was lost in all the lin-29a(xe38) mutant males. Quantification is shown on the right (n = 15). Scale bar: 10 µm. (B) Male-specific expression of dmd-5 in AVG requires lin-29a. Expression of the DM-containing transcription factor promoter fusion dmd-5 (pUL#JS9B3) is observed in adult males but not hermaphrodites in the AVG interneuron. In the absence of lin-29a, expression is lost from AVG in 80% of the male worms (top panels); quantification is shown on the right (n = 15). The expression of the daf-7/TGFβ-like molecule promoter fusion is observed in adult males but not hermaphrodites and this expression is lost in the lin-29a(xe38) mutant males (bottom panels). Quantification is shown on the right (n = 15). Scale bar: 10 µm. (C) lin-29a is required for normal PDB branching. A cis-regulatory element from the kal-1 locus is expressed in a number of neurons in the tail region in both sexes, including the PDB –inter and motorneuron (Wenick and Hobert, 2004). kal-1::gfp reporter expression showed that the PDB neuron process exhibits elaborate branching in the male after sexual maturation but not at earlier larval stages. In the absence of lin-29a, PDB branching is lost from adult males (bottom panels). Quantification of total neurite length is shown on the right (n = 10). PDB neurites establish extensive electrical and chemical synapses with sex-shared and male-specific neurons exclusively in adult males (schematized based on data from (Jarrell et al., 2012); synaptic partners of PDB present in both sexes are in blue and in black if sex-specific) . Scale bar: 5 µm.
The expression of lin-29a in a number of distinct neuron types indicated that lin-29a may play additional roles in controlling sexually dimorphic, that is, male-specific nervous system features. To this end, we assessed two other molecular markers that were previously found to be expressed in a sexually dimorphic manner in two distinct, sex-shared neuron classes, both of which express lin-29a. First, the dmd-5 transcription factor was reported to be expressed in AVG neurons in adult males but not in hermaphrodites (Oren-Suissa et al., 2016). By analyzing the expression of a dmd-5 reporter in wild type versus lin-29a mutants, we found that lin-29a is required for dmd-5 expression in AVG in males (Figure 6B). Second, the daf-7 gene, encoding a neuroendocrine TGFβ-like signal, is turned on during sexual maturation in the sex-shared ASJ neurons only in males (Hilbert and Kim, 2017). This induction is also abrogated in lin-29a mutants (Figure 6B). Non-sexually dimorphic expression of dmd-5 and daf-7 in other neuron types was not affected by lin-29a.
The electron micrographical reconstruction of the nervous system of C. elegans male revealed sexually dimorphic neuronal morphology and connectivity of several sex-shared neurons (Jarrell et al., 2012; White et al., 1986). Specifically, the lin-29a-expressing PDB motorneuron located in the pre-anal ganglion grows elaborate branches off from its cell body and initial segment of its posteriorly directed main process. This was only observed in the male but not in the hermaphrodite (Jarrell et al., 2012; White et al., 1986). These male-specific branches make extensive chemical and electrical synaptic contacts with both male-specific neurons, as well as sex-shared neurons (Jarrell et al., 2012). We corroborated the existence and reproducibility of male-specific branches by visualizing PDB with a reporter transgene (Figure 6C). We found that these branches grow specifically during sexual maturation in the L4 stage (Figure 6C), co-incident with the onset of lin-29a expression. Growth of these branches is disrupted in lin-29a mutants (Figure 6C). These findings demonstrate a role for lin-29a in regulating the temporal and sexually dimorphic acquisition of male-specific neuronal morphologies and predict that PDB fails to make its branch-specific synaptic contacts in the absence of lin-29a. We conclude that lin-29a affects multiple distinct types of sexual maturation events, from gene expression to anatomy, in distinct neuron classes.
lin-29a mutant males display defects in mating behavior
We next sought to broaden our understanding of lin-29a function in the nervous system by testing if lin-29a was required for the establishment of adult-specific sexually dimorphic behaviors. Critical for such an analysis is the fact that lin-29a mutant males do not display the obvious morphological and patterning defects displayed by the lin-29 null allele. Specifically, the male tail, whose overall morphogenesis is disrupted in lin-29 null mutants (Euling et al., 1999) appears morphologically normal in lin-29a mutants (Figure 7A). We precisely quantified other morphological differences between hermaphrodites and males using an automated visualization/measurement tool (Yemini et al., 2013) and found no overt morphological differences between wild-type and lin-29a mutant males (Figure 7B). The normal overall appearance of lin-29a mutant males allowed us to first ask whether lin-29a mutant males displayed mating-related behavioral defects. One aspect of mate attraction relates to the mating process itself, in which the male detects various sensory cues from the hermaphrodites to engage in direct contact (Liu and Sternberg, 1995). Previous work with the lin-29 null mutant revealed multiple defects in these direct interactions and these defects were ascribed to spicule patterning defects (Euling et al., 1999). In spite of spicule patterning appearing normal in lin-29a isoform-specific mutants, we observed that these mutants were unable to mate (Figure 7C). We found that one aspect of mating behavior defective in these mutants is vulva location behavior (Figure 7C).
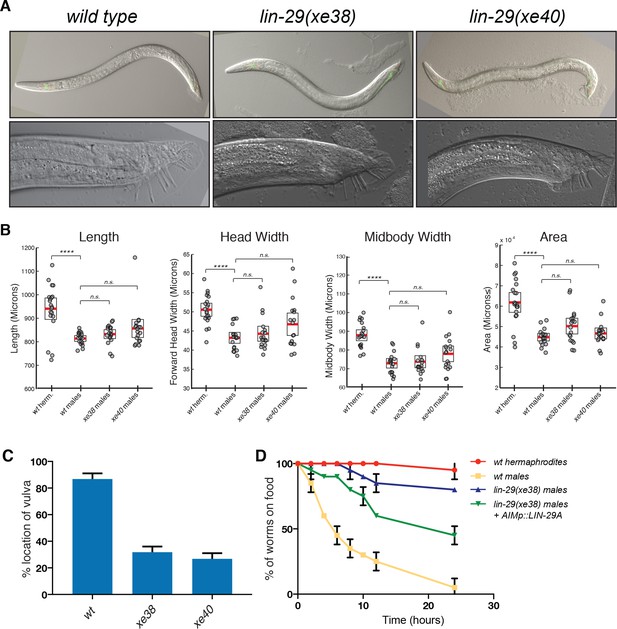
lin-29a is required for male mating behaviors.
(A) lin-29A mutants show a normal male tail morphology. DIC images for young adult wild type and lin-29a mutants xe38 and xe40. No morphological defects were detected in the young adult lin-29a mutant males compared to wild type. 20x images for young adult males are shown on the top panels. 63x images of the male tail are shown on the bottom panels. (B) WormTracker analysis of sexually dimorphic postural features showed no difference between wild-type and lin-29a mutant males. Hermaphrodites and males were tracked for 5 min and features describing body posture were analyzed using the WormTracker software. Adult hermaphrodites and males showed sex-specific differences in posture including length, head width, midbody width and area. When wild-type males were compared to lin-29a xe38 and xe40 mutant males no significant differences were found. (C) lin-29a is required for male mating. The ability of the of lin-29a(xe38) and lin-29a(xe40) mutant males to locate the hermaphrodite vulva is significantly reduced compared to lin-29(+) control males (n = 15). (D) lin-29a is required for male-specific mate searching behavior. Young adult males are followed over time and scored for movement beyond 3 cm away from the food source. lin-29a(xe38) mutant males failed to leave the food and search for mates and behaved similarly to wild-type hermaphrodites. lin-29a mate-searching defect could be partially rescued by restoring LIN-29A expression in AIM interneurons (otEx7316). Values plotted are an average of two independent experiments (n = 15 for each experiment).
The AIM and ASJ neurons, both of which express lin-29a specifically in males, were previously implicated in mate-searching, which constitutes another aspect of mating behavior (Barrios et al., 2012; Hilbert and Kim, 2017). In this behavioral paradigm, well-fed single adult males leave a source of food to explore their environment in search for mating partners. We indeed found that lin-29a mutants are defective in mate searching behavior (Figure 7D). These defects are similar to the defects caused by loss of daf-7/TGFβ (Hilbert and Kim, 2017) and, consistent with this phenotypic similarity, we had described above that daf-7/TGFβ expression is lost in the ASJ neurons of lin-29a mutants. Nevertheless, we could partially rescue the mate searching defects of the lin-29a mutant males by restoring LIN-29A expression exclusively in the AIM neurons (Figure 7D), indicating that lin-29a may function in several distinct neurons to control mate searching behavior.
Feminization of locomotor patterns of lin-29a mutant males
The male-specific expression of lin-29a in ventral nerve cord and head motor neurons and in a command interneuron pair made us consider whether lin-29a may be involved in controlling aspects of sexually dimorphic locomotor behavior. It has previously been shown that adult male and hermaphrodites display distinct locomotor behavior when crawling on a lawn of bacteria (Mowrey et al., 2014). We extended the analysis of sexually dimorphic adult-specific behavior using an automated worm tracker system which quantifies several hundred distinct postural and locomotory features of an animal (Yemini et al., 2013). We found a large number of sexually dimorphic features. While many of them were not affected in lin-29a mutant animals (Figure 8 and Supplementary file 1), we found that lin-29a mutants displayed a striking feminization of a number of body posture and locomotory features. Postural differences included head bend, neck bend, midbody bend, hips bend, amplitude ratio and primary wavelength. (Figure 8). Other lin-29a-dependent locomotory features included midbody crawling amplitude and tail crawling amplitude (Figure 8). The lack of morphological differences between wild-type and lin-29a mutant males indicates that these locomotory phenotypes are the result of neuronal defects rather than being a secondary consequence of overall body morphology. Taken together, these results show that lin-29a is required for the acquisition of male-specific postural and locomotory features and that the absence of lin-29a results in a feminization of these behavioral parameters.
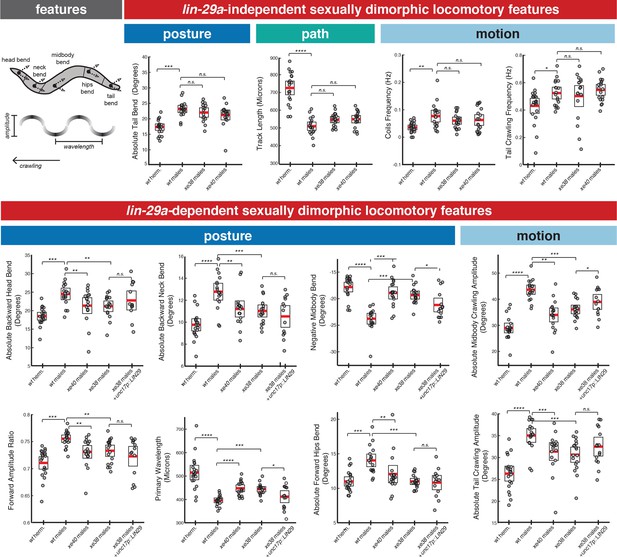
lin-29a is required for the establishment of male-specific features of locomotor behavior.
There are lin-29a dependent and lin-29a independent adult-specific sexually dimorphic locomotory features. Cartoon of an adult C. elegans depicts the location of the body parts used for feature computation. The arrows for each body part represent the bend angle. Wavelength and amplitude while crawling are shown. Adult hermaphrodites and males showed sex-specific differences in posture, path and locomotory features that were not affected by lin-29a absence (top panel). A complete list of sexually dimorphic features not affected by lin-29a is provided in Supplementary file 1. In lin-29a mutant males, a number of sexually dimorphic features were feminized compared to wild-type males (bottom panel), including posture and locomotory features. Restoration of lin-29a expression in command interneuron AVA and ventral nerve cord motor neurons (otEx7320) partially rescued the feminization of two postural features (midbody bend and wavelength) as well as one locomotory feature (midbody crawling amplitude) (n = 20).
We sought to determine in which of the neurons that express lin-29a in a sexually dimorphic manner lin-29a acts to control aspects of the locomotor behaviors described above. We particularly considered the so-called ‘motor circuit’, composed of a set of command interneurons and ventral nerve cord motor neurons (Von Stetina et al., 2006). Expression of lin-29a in the motor circuit, using a cis-regulatory element from the unc-17/VAChT locus rescued a defined subset of the feminized behavioral features (wavelength, crawling amplitude and midbody bend) (Figure 8). Other lin-29a-expressing neurons may be involved in controlling locomotory and postural features not rescued by this transgene.
Intersectional control of the temporal, sexual and spatial specificity of lin-29a expression
Having established the function of lin-29a in sexually dimorphic development and function of the C. elegans nervous system, we sought to better understand the mode of lin-29a regulation. lin-29a shows a striking specificity of expression in three different dimensions. Within the nervous system, lin-29a is expressed in a temporally controlled manner (onset in embryonically born neurons only in the L4 stage), in a sex-specific manner (only in males) and it is expressed in a highly neuron type-specific manner. In as far as the first dimension, time, is concerned, we have described above that lin-29a expression in the nervous system is temporally controlled by the lin-28/let-7/lin-41 heterochronic pathway. To assess the control of sex-specificity of lin-29a expression, we genetically removed tra-1, the master regulatory transcription factor of sex determination which is normally expressed in all hermaphroditic cells (XX sex chromosome genotype) where it suppresses male identities (Schvarzstein and Spence, 2006). We found that in tra-1(e1488) mutants, lin-29a expression becomes derepressed in XX animals (Figure 9A). Moreover, we induced the degradation of tra-1 exclusively in the nervous system by force-expressing fem-3, which promotes TRA-1 protein destruction (Starostina et al., 2007), under the control of a panneuronal driver. We found that lin-29a expression is derepressed in the nervous system of the hermaphrodite, with the same pattern of cellular specificity seen normally in males (Figure 9A).
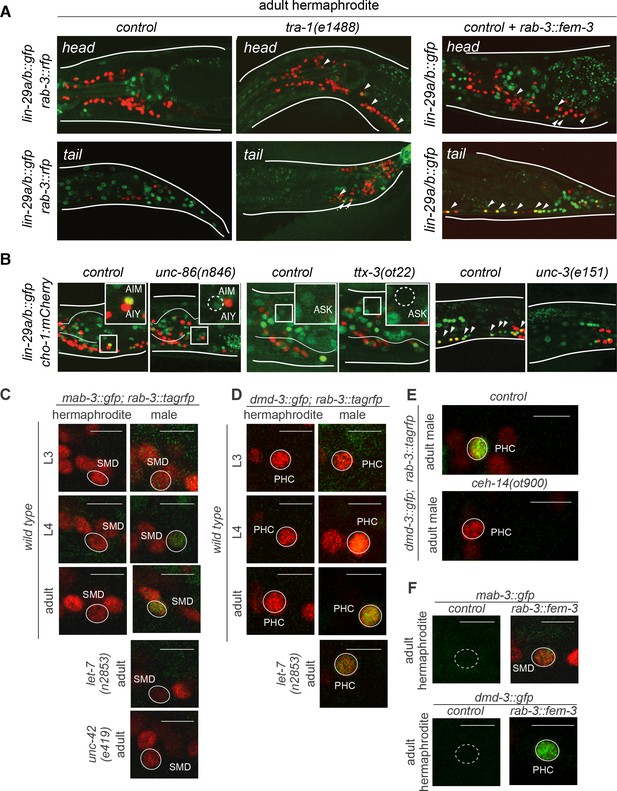
Intersectional control of distinct lin-41 effectors via heterochronic, sexual (tra-1) and spatial (terminal selector) inputs.
(A) LIN-29 neuronal expression is regulated by the sex-determination pathway. Mutant hermaphrodites for the downstream effector of the sex-determination pathway tra-1 showed lin-29a/b::gfp (xe61) expression in hermaphrodite neurons in L4 and adult stages. Masculinization of the hermaphrodite nervous system by a pan-neuronal promoter driving fem-3 (otEx7321), that represses tra-1 function, induced lin-29a/b::gfp (xe61) expression in hermaphrodite neurons in L4 and onwards. (B) lin-29a/b::gfp neuronal expression is lost in neuron-specific transcription factor mutants. The POU homeobox transcription factor unc-86 is required for lin-29a/b::gfp expression in AIM interneurons. The LIM-HD transcription factor ttx-3 is required for lin-29a/b::gfp expression in ASK. The COE/EBF transcription factor unc-3 is required for lin-29a/b::gfp expression in AVA, SAB, DA, DB, VA, VB, PDA, PDB and PVC neurons. Cholinergic neurons are labeled with the cho-1/CHT mCherry fosmid reporter. (C) mab-3::gfp expression in the SMD neurons in control hermaphrodites, males and mutant animals. mab-3::gfp (ot931) expression was determined during larval development. GFP was first observed in the SMD neurons at the L4 stage and onwards, only in males. mab-3::gfp expression was lost from SMD neurons in a let-7(n2853ts) mutant male. L1 let-7(n2853ts) animals were shifted to the restricted temperature (25°C) and imaged as adults, after 48hs. mab-3::gfp expression was lost from SMD neurons in an unc-42(e419) mutant male. Scale bar: 5 µm. (D) dmd-3::gfp expression in the PHC neuron in control hermaphrodites, males and mutant animals. GFP was first observed in the PHC neurons at the L4 stage and onwards, only in males. dmd-3::gfp expression was decreased from PHC neurons in a let-7(n2853) mutant male. L1 let-7(n2853) animals were shifted to the restricted temperature (25°C) and imaged as adults, after 48hs. (E) dmd-3::gfp expression in PHC was lost in ceh-14 null mutant males and in let-7(n2853ts) mutant males. L1 let-7(n2853ts) animals were shifted to the restricted temperature (25°C) and imaged as adults, after 48hs. (F) Masculinization of the hermaphrodite nervous system by a pan-neuronal promoter driving fem-3 (otEx7322 and otEx7323, respectively), that promotes TRA-1 degradation, induced mab-3::gfp (ot931) expression in hermaphrodite SMD neurons and dmd-3::gfp (ot932) expression in hermaphrodite PHC neurons in L4 and onwards.
To address the cellular specificity of lin-29a expression, we considered the possibility that neuron class-specific terminal selector-type transcription factors that control the expression of neuron-class specific identity programs (Hobert, 2016) may also control the neuronal specificity of lin-29a expression. For example, the terminal differentiation program of the lin-29a expressing ASK neurons is controlled by the LIM homeobox gene ttx-3/LHX2 and the identity of the AIM neuron is defined by the unc-86/BRN3 POU homeobox gene (Serrano-Saiz et al., 2013). We found that lin-29a expression is eliminated in the ASK neurons of ttx-3 mutants and in the AIM neurons of unc-86 mutants (Figure 9B). The unc-3/COE-type transcription factor controls the identity of multiple neurons of the motor circuit, including the lin-29a expressing ventral nerve cord motorneurons, the SAB head motor neurons, the PDA and PDB tail motor neurons and the command interneurons AVA and PVC (Kratsios et al., 2011; Pereira et al., 2015). We found that in unc-3 mutants lin-29a expression is lost in all these neurons (Figure 9B). We conclude that the complex lin-29a expression pattern can be explained by an intersectional mechanism. Temporal control is exerted via the heterochronic pathway, sexual specificity by the sex determination pathway and cellular specificity by terminal selector-type neuronal identity factors.
Other male-specific effectors of lin-41 are also controlled in an intersectional manner
The sexually dimorphic expression pattern of lin-29a in the male nervous system does not extend to all sex-shared neurons that are known to display sexually dimorphic features. For example, the sex-shared PHC sensory neuron functionally and anatomically remodels during sexual maturation (Serrano-Saiz et al., 2017a), but does not express lin-29a. This suggests that lin-29a is possibly just one of several targets of LIN-41 in the context of male-specific nervous system differentiation. Previous whole animal RNA profiling of let-7 and lin-41 mutants, as well as RNA-binding assays demonstrated that LIN-41 also represses mab-3 and dmd-3 mRNA (Aeschimann et al., 2017). Both genes are members of the DMD family of sex-specifically expressed transcription factors (Mason et al., 2008; Yi and Zarkower, 1999) and were previously shown to be expressed in male-specific neurons and in a male-specific manner in distinct, but sex-shared neurons (Mason et al., 2008; Serrano-Saiz et al., 2017a; Yi et al., 2000). Their timing of expression had not been previously examined.
We gfp-tagged the endogenous mab-3 and dmd-3 loci using CRISPR/Cas9 genome engineering. Within the sex-shared nervous system, we found that mab-3 is not only male-specifically expressed in the ADF neuron, as previously reported (Yi et al., 2000), but also in additional head neurons, including the embryonically generated SMD head motor neurons (Figure 9C). We found that like the male-specific, neuronal expression of lin-29a, male-specific neuronal expression of mab-3 is precisely timed to commence at the L4 stage (Figure 9C). Similarly, the gfp-tagged dmd-3 locus is expressed in a sex-shared neuron class, the PHC neurons (Figure 9D), where dmd-3 acts to control the sexually dimorphic remodeling of PHC (Mason et al., 2008; Serrano-Saiz et al., 2017a). dmd-3::gfp expression in PHC also commences at the L4 stage (Figure 9D), at the time when PHC neurons remodel in a male-specific manner (Serrano-Saiz et al., 2017a). We corroborated our previous data of LIN-41 protein affecting mab-3 and dmd-3 expression (Aeschimann et al., 2017) by showing that the temporal specificity of nervous system expression of mab-3 and dmd-3 is indeed lost in let-7 mutants (Figure 9C–D).
Like lin-29a, the male-specificity of mab-3 and dmd-3 expression in the nervous system is controlled by the tra-1 transcription factor (Figure 9E,G) Moreover, the neuronal specificity of mab-3 and dmd-3 expression is, like lin-29a, also controlled by neuron-type specific terminal selectors. unc-42 is a terminal selector transcription factor that controls terminal differentiation of the RMD and SMD neurons (Pereira et al., 2015), and we found that mab-3 expression in these neurons fails to be induced in an unc-42 mutant background (Figure 9C). Similarly, ceh-14 is a terminal selector of PHC neuron identity (Serrano-Saiz et al., 2017a) and is required for dmd-3 expression in PHC (Figure 9F). We conclude that lin-41 operates through multiple distinct, male-specific effector genes and that the temporal, sexual and spatial specificity of expression of these distinct effectors is controlled by a similar, intersectional regulatory logic (Figure 10A,B).
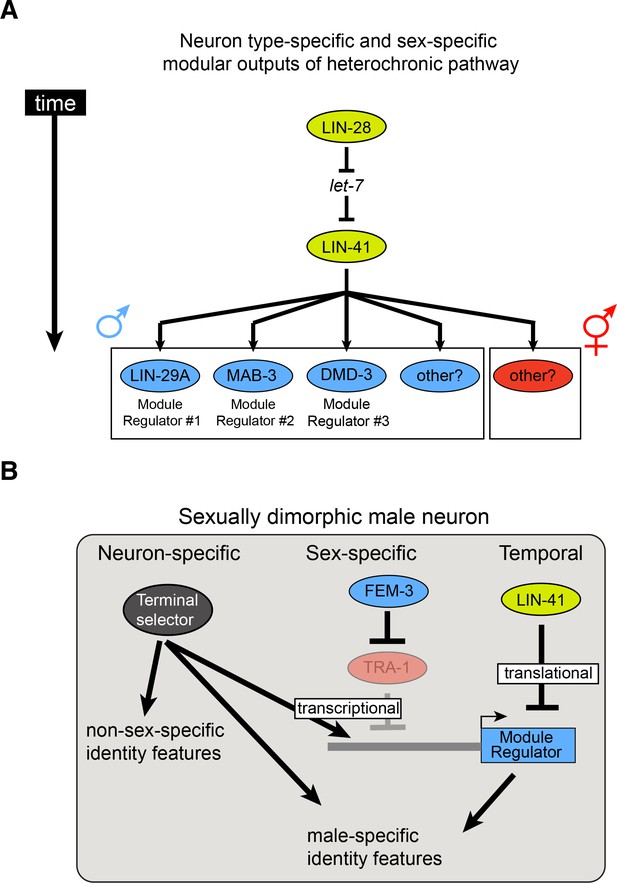
Timing mechanisms controlling sexually dimorphic nervous system differentiation during sexual maturation.
(A) The evolutionarily conserved lin-28/let-7/lin-41 heterochronic pathway controls the timing of the expression of multiple neuron-specific effectors in the male nervous system. The RNA-binding protein LIN-28 controls the expression of the miRNA let-7, which in turn controls expression of the RNA-binding protein LIN-41. LIN-41 let-7-mediated downregulation at the onset of sexual maturation de-represses LIN-41 targets LIN29A, MAB-3 and DMD-3. Expression of these LIN-41 effectors in specific neurons in males, at the onset of sexual maturation, constitute effector modules directing the precisely timed nervous system masculinization. We hypothesize that similar LIN-41 effectors, not identified yet, could be acting in other neurons in the male nervous system and in the hermaphrodite nervous system. (B) Temporal, sex-specific and neuron-specific intersectional control of nervous system differentiation during sexual maturation. A hypothetical male neuron is shown. As discussed above, LIN-41 repression of the heterochronic pathway is relieved at the onset of sexual maturation, allowing neuron-specific effector proteins to be expressed. In males, degradation of the ubiquitously expressed transcriptional repressor TRA-1 allows expression of male-specific regulatory effectors (blue boxes same as panel A). The neuron type-specificity of regulatory effector gene expression is determined by terminal selector transcription factors that control the expression of many other neuronal identity features. Hence, terminal selectors define a neuron type-specific, but permissive state whose sex- and time-specificity is controlled by factors that antagonize the effect of terminal selectors on regulatory effector genes (transcriptionally – TRA-1; translationally – LIN-41). Note that features that are controlled by sex/time-specific regulatory effectors, exemplified by LIN-29A regulation of neurotransmitter pathway genes, are also controlled by terminal selectors, thereby constituting a ‘feedforward’ regulatory architecture.
Discussion
We have described here that the timing of multiple distinct, neuron-type specific sexually dimorphic maturation events in the nervous system of C. elegans are controlled by a phylogenetically conserved regulatory cassette composed of ubiquitously expressed and temporally controlled genes, lin-28 (a regulator of let-7 miRNA processing), let-7 (a miRNA) and its target lin-41 (a translational regulator). The conservation of the deployment of this pathway in the timing of sexual maturation is remarkable. Genetic manipulations in mice, as well as genome-wide association studies of humans with abnormally timed puberty demonstrate that lin-28 and let-7 also control timing of sexual maturation (puberty) onset in mammals (Chen et al., 2017; Corre et al., 2016; He et al., 2009; Ong et al., 2009; Perry et al., 2009; Sulem et al., 2009; Zhu et al., 2010). This conclusion was further corroborated by the finding that mutations in human makorin, the orthologue of lep-2, a regulator of lin-28 activity in C. elegans (Herrera et al., 2016), also affect the timing of puberty in humans (Abreu et al., 2013; Bulcao Macedo et al., 2014). Similarly, in the fly nervous system, lin-28 and let-7 are involved in controlling the timing of pupal to adult transitions (González-Itier et al., 2018; Sempere et al., 2003; Wu et al., 2012) that are accompanied by sexual maturation in the nervous system, as assessed by expression of the male-specific isoform of Fruitless, a master regulator of male-specific nervous system differentiation (Lee et al., 2000). However, a regulatory linkage between the heterochronic pathway and transcriptional regulators of sexual differentiation (hormone receptors in vertebrates or Fruitless in flies) has not yet been established in these systems.
Apart from suggesting a remarkable conservation of timing mechanisms of sexual maturation, we have provided insights into the mechanisms by which the lin-28/let-7 axis affects the timing of sexual maturation. The let-7 miRNA is known to have a plethora of distinct mRNA targets (Büssing et al., 2008), but the one that we define here as being relevant in the context of sexual maturation is the translational regulator lin-41 (Slack et al., 2000). C. elegans lin-41 had previously been implicated as an output of let-7 in the timing of skin cell maturation events (Slack et al., 2000) as well as vulval patterning (Ecsedi et al., 2015). lin-41 is also highly conserved in vertebrates where its expression appears to be temporally regulated during embryogenesis with its expression being complementary to let-7, as observed in C. elegans (Schulman et al., 2005). The dynamics of lin-28, let-7 and lin-41 expression has not yet been comprehensively examined in the context of sexual maturation in the vertebrate brain, but notably, the expression of Lin28 and Let7 in the hypothalamus, a site of release of peptides that control pubertal onset, appears to be temporally controlled (Sangiao-Alvarellos et al., 2013). In Drosophila, let-7 expression has also been found to be temporally regulated; its expression increases during late pupal stages (Faunes and Larraín, 2016). This is the time at which at least some parts of the Drosophila nervous system sexually mature (Lee et al., 2000), but a role of Drosophila let-7 in the timing of sexual maturation has not yet been assessed.
One key remaining question, raised by our studies in C. elegans, is how lin-28/Lin28 function is precisely timed in the nervous system. Surprisingly, we found that a previously described upstream regulator of lin-28, the lin-4 miRNA (Moss et al., 1997), does not affect male-specific sexual maturation in the nervous system, suggesting the existence of alternative pathways that determine when lin-28’s function in controlling let-7 processing is downregulated. Recent work in C. elegans has discovered LIN-28 protein degradation, mediated by the conserved LEP-2/Makorin protein, as another means to control lin-28 function (Herrera et al., 2016). It remains to be explored how LEP-2/Makorin-mediated LIN-28 degradation is temporally controlled.
We showed here that the key target of let-7, the lin-41 translational regulator, relays temporal information in a modular manner to distinct, neuron class-specific outputs (Figure 10A). Among them is the phylogenetically conserved LIN-29 Zn finger transcription factor (whose vertebrate orthologs ZNF362 and ZNF384 are completely unstudied), which we identified as being sexually dimorphically expressed in one fifth of all sex-shared neuron classes. Two other targets of lin-41 that are relevant for sexual maturation are two male-specifically expressed DM domain transcription factors (Aeschimann et al., 2017) (this paper). Neurons that express these sexually dimorphic output regulators (lin-29, mab-3, dmd-3) act at distinct levels of information processing, from sensory, to inter- to motorneurons. Intriguingly, many of the sex-shared, LIN-29(+) interneurons are synaptically connected and some of these connections are sexually dimorphic. Some of the neurons that express LIN-29 or MAB-3 in a male-specific manner were not previously shown to display any sexual dimorphisms, demonstrating that the C. elegans nervous system may be even more sexually dimorphic than previously appreciated.
lin-29, mab-3 and dmd-3 expression mark many, but not quite all sex-shared neurons with sexually dimorphic features. We indeed found evidence for two other dmd genes whose expression is both sexually and temporally controlled in yet distinct neuron classes (Oren-Suissa et al., 2016) (unpubl. data). Altogether, these findings suggest a scenario in which a ubiquitously expressed temporal coordinate system (heterochronic pathway), intersects with a ubiquitously expressed sexual coordinate system (sex determination pathway) to control the sexual and temporal specificity of a number of modular, neuron-type specific output systems that control sexual dimorphisms in distinct cellular circuit modules (Figure 10A,B). This modular organization displays striking conceptual similarities with the modular organization of sexual dimorphic control mechanisms in vertebrates (Yang and Shah, 2014).
How is the neuron type-specificity of the modular outputs (lin-29, mab-3, dmd-3 etc.) of the temporal and sex-determination system controlled? We provided evidence here that the neuron-type specificity of the sex-specific regulator is controlled by neuron-type specific terminal selector transcription factors which define the overall differentiated state of individual neuron classes (Hobert, 2016) (Figure 10B). For example, unc-3 is required for many (non-sexually dimorphic) aspects of motor neuron differentiation in the ventral nerve cord and we find that unc-3 also is required to target lin-29 expression specifically in ventral nerve cord neurons. Like the regulation of lin-29 by the temporal cue lin-41, the neuron-type specific regulation of lin-29 could potentially be direct since we noted several phylogenetically conserved binding sites for UNC-3 protein in the lin-29 locus. The regulation of sex-specific output regulators, like lin-29, by terminal selectors reveals the potential existence of a specific gene regulatory network architecture. For example, in the male AIM neurons, unc-86 and ceh-14 are required for the induction of unc-17 and cho-1 expression (Pereira et al., 2015). Both are apparently not sufficient to induce unc-17 and cho-1 expression in hermaphrodites, but via the unc-86-mediated induction of lin-29 expression in the AIM neurons in males, unc-86 and ceh-14 are now apparently able to induce, with the help of lin-29, the expression of unc-17 and cho-1. Further biochemical studies will be required to corroborate the existence of such feedforward architecture (Figure 10B). In conclusion, we have identified an intersectional regulatory mechanism in which temporal cues, sexual cues and neuron-type specific cues define the onset of expression of sex-specific, neuron-type specific ‘output regulators’ which define sexual dimorphic features of the C. elegans nervous system (Figure 10).
Materials and methods
C. elegans strains
Request a detailed protocolWorm strains used in this study are listed in Table 1. The wild-type strain was Bristol N2. Worms were grown on nematode growth media (NGM) seeded with bacteria (OP50) as a food source. To synchronize worms 10 adult gravid hermaphrodites were transferred to a new plate with OP50. Worms were allowed to lay eggs for approximately two hours and adult gravid hermaphrodites were removed afterwards.
Strain list.
https://doi.org/10.7554/eLife.42078.015Strain name | Genotype | Relevant DNA on array or single copy transgene |
---|---|---|
DR466 | him-5(e1490) | |
MT1524 | lin-28(n719) | |
MT7897 | lin-41(n2914)/unc-29(e1072); lin-11(n1281) | |
MT7626 | let-7(n2853) | |
HW1814 | lin-41(xe8/bch28); him-5(e1490) 1 | |
VT132 | lin-29a/b(n333)/mnC1; sqt-1(sc13) | |
CB4037 | glp-1(e2141) | |
HW1672 | lin-29a/b(xe37) | |
HW1693 | lin-29a(xe38) | |
HW1695 | lin-29a(xe40) | |
HW1698 | mab-10(xe44) | |
CB2823 | tra-1(e1488)/eDp6 | |
MT1859 | unc-86(n846) | |
OH161 | ttx-3(ot22) | |
CB151 | unc-3(e151) | |
CB419 | unc-42(e419) | |
OH15422 | ceh-14(ot900) 2 | |
OH15568 | unc-17(ot907[unc-17::mKate2::3xflag]) | |
DG3913 | lin-41(tn1541[lin-41::gfp]) | |
HW1822 | lin-29(xe61[lin-29a/b::gfp::3xflag]) | |
HW2224 | lin-29(xe63 [gfp::3xflag::lin-29a]); him-5(e1490) | |
HW1835 | lin-29(xe65 [lin-29b::gfp::3xflag; lin-29(xe40)]) | |
HW2047 | mab-10(xe75[mab-10::flag::mCherry]) | |
HW2225 | lin-29(xe63); him-5(e1490); let-7(n2853) | |
HW2342 | lin-29(xe63); him-5(e1490); lin-41(n2914)/unc-29(e1072); lin-11(n1281) | |
HW2344 | lin-29(xe63); him-5(e1490); lin-41(xe8/bch28) | |
OH15732 | mab-3(ot931[mab-3::3xflag::gfp]) | |
OH15733 | dmd-3(ot932[dmd-3::3xflag::gfp]) | |
OH10689 | otIs355 | rab-3::nls::tag_rfp |
OH12543 | otIs534 | cho-1 fosmid::sl2::yfp::h2b |
OH12655 | otIs544 | cho-1 fosmid::sl2::mCherry::h2b |
OH13083 | otIs576 | unc-17 fosmid::gfp |
OH11124 | otIs388 | eat-4 fosmid::sl2::yfp::h2b |
OH12496 | otIs518 | eat-4 fosmid::sl2::mCherry::h2b |
OP535 | wtIs535 | lin-28 fosmid::gfp |
HW2172 | xeSi182 | plin-41::gfp(pest)::h2b::lin-41 3’UTR |
HW2173 | xeSi202 | plin-41::gfp(pest)::h2b::unc-54 3’UTR |
UR219 | fsIs5 | srj-54p::yfp |
UL2497 | Ex(dmd-5p::gfp) | dmd-5p::gfp (pUL#JS9B3 plasmid) |
FK181 | ksIs2 | daf-7p::gfp |
EB2509 | dzIs75 II | kal-1p::gfp 3 |
OH15741 | lin-29(xe38) + otEx7316 | eat-4p::lin-29a |
OH15742 | lin-29(xe38) + otEx7317 | eat-4p::lin-29a |
OH15743 | lin-29(xe40) + otEx7318 | eat-4p::lin-29a |
OH15744 | lin-29(xe40) + otEx7319 | eat-4p::lin-29a |
OH15745 | lin-29(xe38) + otEx7320 | unc-17p::lin-29a |
OH15746 | lin-29(xe61) + otEx7321 | rab-3p::fem-3::mCherry |
OH15748 | mab-3(ot931) + otEx7322 | rab-3p::fem-3::mCherry |
OH15749 | dmd-3(ot921) + otEx7323 | rab-3p::fem-3::mCherry |
-
1The xe8 gain of function and bch28 null alleles have been described in Ecsedi et al. (2015) and in Katic et al. (2015). These alleles were maintained in trans to improve health of the animals. Offspring homozygous for either allele were scored for phenotypic traits
-
3The kal-1 gfp promoter fusion corresponds to ‘promoter G’ from Wenick and Hobert, 2004
srj-54::yfp is a PCR fragment that was constructed using standard PCR fusion methods by fusing fragment A, comprising 664 bp upstream of the predicted ATG of srj-54 and the first four codons of srj-54 coding sequence, to fragment B, comprising yfp fused to the unc-54 3´ UTR. srj-54::yfp was injected with unc-122::gfp into him-5(e1490) to generate a stable extrachromosomal array. This array was integrated into the genome using standard UV irradiation methods followed by several rounds of backcrossing to him-5(e1490) to generate fsIs5.
For neuron-specific expression of lin-29a, promoter constructs driving lin-29a cDNA were generated by Gibson assembly or RF cloning. cDNA was amplified from pDH06, kindly provided by Dr. Horvitz (Harris and Horvitz, 2011). A 1 kb of the unc-17/VACHT promoter that drives expression in AVA, SAB, DA, DB, VA, VB, PDA and PDB neurons (our unpublished data) was amplified by PCR from the fosmid construct (FW AATGAAATAAGCTTGCATGGTATACACCAATCATTTCTCC and REV TCCTCTAGAGTCGACCTGCAGATAATTTAATTAAAATTGAGTTCCAAC). The PCR fragments were assembled into pPD95.75 by Gibson. For AIM-specific expression a fragment of the eat-4 locus was used to drive LIN-29A expression. The lin-29a cDNA was inserted to replace the fem-3 cDNA in this construct (Pereira et al., 2015). For nervous system masculinization, a rab-3 pan-neuronal promoter driving fem-3::mCherry was used (White et al., 2007). Transgenic lines carrying extrachromosomal arrays were generated by germline injection with 20 ng/ul of plasmid DNA and unc-122::gfp as a co-injection marker, also at 20 ng/ul.
Genome engineering
Request a detailed protocolIsoform-specific gfp::3xflag tagging of endogenous lin-29 using CRISPR/Cas9. In order to obtain the lin-29(xe65) allele, the following mix was injected into lin-29a(xe40) mutant worms: 50 ng/µl pIK155, 100 ng/µl of pIK198 with a cloned sgRNA (atattatttatcagtgattg), 2.5 ng/µl pCFJ90, 5 ng/µl pCFJ104 and 10 ng/µl pFA26 (pDD282 with cloned homology arms). Recombinants were isolated according to the protocol by Dickinson et al. (Dickinson et al., 2015), verified by DNA sequencing and outcrossed three times. The plasmid for homologous recombination (pFA26) was previously described (Aeschimann et al., 2017). The lin-29(xe63) allele specifically tags lin-29a with GFP::3xFLAG at the N-terminus (Aeschimann et al., 2018).
Tagging of endogenous mab-10 with flag::mCherry using CRISPR/Cas9. In order to tag mab-10 with flag::mCherry at the C-terminus, the following mix was injected into unc-119(ed3) worms (Dickinson et al., 2015; Katic et al., 2015): 25 ng/ul pIK155, 65 ng/ul of pIK198 with a cloned sgRNA (gctcccggaatcttgaagct), 10 ng/ul pIK127 (Peft-3::gfp::h2b), 5 ng/ul Pmyo-3::gfp and 65 ng/ul pIK284. The plasmid serving as a template for homologous recombination (pIK284) was obtained using the SapTrap protocol (Schwartz et al., 2016), by combining SapTrap component vectors pMLS257, pMLS291 and pMLS382 with two pairs of annealed oligos (oIK1052/oIK1053, oIK1054/oIK1055). Wild-type moving recombinants were isolated, verified by DNA sequencing and outcrossed three times. The mab-10::mCherry allele is not fully functional since mab-10(xe75) and mab-10(xe44) both enhance the bursting phenotype of let-7(n2853ts) (data not shown).
lin-29, lin-29a and mab-10 null mutant alleles. In order to specifically mutate lin-29a without affecting expression of lin-29b, we introduced deletions into the coding exons specific to lin-29a, at the same time introducing a frame-shift in the downstream lin-29a reading frame. The lin-29a(xe38) allele is a 10 bp deletion in exon 2, resulting in a frame-shift and a stop codon in exon 3. It was obtained by injecting a single sgRNA (gctggaaccaccactggctc) and has the following flanking sequences: 5’ gaatagctggaaccaccact – xe38 deletion – ctaccacccattttggtgtt 3’. The lin-29a(xe40) allele is a 1102 bp deletion covering exons 2–4, introducing a frame-shift in the lin-29a reading frame with a predicted stop codon in exon 6 (Aeschimann et al., 2018). We used deletions of almost the entire coding regions as null alleles for lin-29 and mab-10: The lin-29(xe37) allele is a 14.8 kbp deletion spanning lin-29 exons 2–11 and the mab-10(xe44) allele is a 2.9 kbp deletion spanning mab-10 exons 3–9 (Aeschimann et al., 2018).
To obtain mutant worm lines, wild-type worms were injected with a mix containing 50 ng/µl pIK155, 100 ng/µl of each pIK198 with a cloned sgRNA, 5 ng/µl pCFJ90 and 5 ng/µl pCFJ104, as previously described (Katic et al., 2015). Single F1 progeny of injected wild-type worms were picked to individual plates and the F2 progeny screened for deletions using PCR assays. After analysis by DNA sequencing, the alleles were outcrossed three times to the wild-type strain.
Tagging of endogenous unc-17 with 3xflag::mKate2 using CRISPR/Cas9. The unc-17 locus was tagged at the C-terminus as previously described (Dickinson et al., 2015; Katic et al., 2015).The plasmid serving as a template for homologous recombination was cloned using Gibson and included 700 bp-long homology arms. Wild-type moving recombinants were isolated, verified by DNA sequencing and outcrossed three times.
Tagging of endogenous mab-3 and dmd-3 with 3xflag::gfp using CRISPR/Cas9. The mab-3 and dmd-3 loci were tagged at the C-terminus as previously described (Dickinson et al., 2015; Katic et al., 2015). The plasmid serving as a template for homologous recombination was cloned using Gibson and included 700 bp-long homology arms. Wild-type moving recombinants were isolated, verified by DNA sequencing and outcrossed three times.
Neuron identification
Request a detailed protocolExpression analysis of the lin-29 endogenously tagged alleles with neuron-specific resolution was done by assessing nuclear position and size using Nomarski optics and crossing these strains with neuronal landmark reporter strains eat-4 (otIs518) (Serrano-Saiz et al., 2017a) and cho-1 (otIs544) (Pereira et al., 2015).
Laser ablation
Request a detailed protocolLaser ablation of Z1/Z4 was performed at the L1 stage as previously described (Hsin and Kenyon, 1999) using a MicroPoint Laser System Basic Unit (N2 pulsed laser (dye pump), ANDOR Technology) attached to a Zeiss Axio Imager Z2 microscope (Objective EC Plan-Neofluar 63X/1.30 Oil). Worms were recovered by washing with M9 buffer and imaged at the young adult stage. Control animals were treated in the same manner, but not subjected to laser exposure. Gonad and germline removal were confirmed by DIC microscopy.
Microscopy
Request a detailed protocolWorms were anesthetized using 100 mM of sodium azide and mounted on 5% agarose on glass slides. All images were acquired using a Zeiss confocal microscope (LSM880; Zeiss [Carl Zeiss], Thornwood, NY). Image reconstruction was performed using the ZEN software tool. Maximum intensity projections of representative images are shown. For neurite tracing, confocal Z-stacks were opened using FIJI, and loaded into the Simple Neurite Tracer plugin. All neurites emerging from the soma or posterior process of PDB were traced. The simple neurite tracer plugin was used to analyze the skeletons for neurite length, which were summed to calculate total neurite.
Mating assays
Request a detailed protocolAnimals were synchronized at the L1 stage and grown to the early L4 stage group housed. The day before the mating assay experiment, males at the early L4 stage were separated to a new plate. Male mating behavior was assayed by direct observation of the mating process. A single young adult male was placed with 10–15 L4 unc-3(e151) hermaphrodites on a mating plate. The male mating behavior was recorded as previously described (Euling et al., 1999). Males were tested for their ability to locate vulva in a mating assay, calculated as location efficiency. The number of passes or hesitations at the vulva until the male firs stops were counted: location efficiency = 1/number of encounters to stop, expressed as percentage.
Mate-searching assay
Request a detailed protocolAnimals were synchronized at the L1 stage and grown to the early L4 stage . The day before the mating assay experiment, hermaphrodites and males at the early L4 stage were separated to a new plate. The mate-searching assay was performed as previously described (Lipton et al., 2004). A single animal was placed in the center of a 15 µl patch of food per 10 cm plate. Each animal that had left the food was scored blindly at 7 time points for a period of 24 hr. A worm was considered a leaver if it was 3 cm away from the food source at the scoring time.
Automated worm tracking
Request a detailed protocolAutomated single worm tracking was performed using the Wormtracker 2.0 system at room temperature (Yemini et al., 2013). Animals were synchronized at the L1 stage and grown to the early L4 stage . The day before the tracking experiments, hermaphrodites and males were separated to same-sex group housed plates. Animals were recorded at the young adult stage for 5 min to ensure sufficient sampling of locomotion related behavioral features. All animals were tracked on NGM plates uniformly covered with food (OP50). To avoid potential variability arising due to room conditions, all strains that were compared in a single experiment were recorded simultaneously in identical room condition, along with the controls. Analysis of the tracking videos was performed as previously described (Yemini et al., 2013). After correction for multiple testing (Bonferroni correction), features shown in Figure 8 and Supplementary file 1 emerged as the ones with most significantly different q-value among the test groups. For the rescue experiment, we only measured these features, permitting us to use the p-value.
Data availability
All data generated or analysed during this study are included in the manuscript and supporting files.
References
-
Central precocious puberty caused by mutations in the imprinted gene MKRN3New England Journal of Medicine 368:2467–2475.https://doi.org/10.1056/NEJMoa1302160
-
Disentangling puberty: novel neuroendocrine pathways and mechanisms for the control of mammalian pubertyHuman Reproduction Update 23:737–763.https://doi.org/10.1093/humupd/dmx025
-
PDF-1 neuropeptide signaling modulates a neural circuit for mate-searching behavior in C. elegansNature Neuroscience 15:1675–1682.https://doi.org/10.1038/nn.3253
-
A novel null allele of C. elegans gene ceh-14microPublication Biology.https://doi.org/10.17912/G434-3D85
-
Stage-specific accumulation of the terminal differentiation factor LIN-29 during Caenorhabditis elegans developmentDevelopment 122:2517–2527.
-
New causes of central precocious puberty: the role of genetic factorsNeuroendocrinology 100:1–8.https://doi.org/10.1159/000366282
-
let-7 microRNAs in development, stem cells and cancerTrends in Molecular Medicine 14:400–409.https://doi.org/10.1016/j.molmed.2008.07.001
-
Association study of LIN28B in girls with precocious pubertyJournal of Pediatric Endocrinology and Metabolism 30:663–667.https://doi.org/10.1515/jpem-2016-0101
-
Sex-specific regulation of weight and puberty by the Lin28/let-7 axisJournal of Endocrinology 228:179–191.https://doi.org/10.1530/JOE-15-0360
-
LIN-41/TRIM71: emancipation of a miRNA targetGenes & Development 27:581–589.https://doi.org/10.1101/gad.207266.112
-
The let-7 microRNA directs vulval development through a single targetDevelopmental Cell 32:335–344.https://doi.org/10.1016/j.devcel.2014.12.018
-
Neural Circuits of Sexual Behavior in Caenorhabditis elegansAnnual Review of Neuroscience 41:349–369.https://doi.org/10.1146/annurev-neuro-070815-014056
-
Terminal Selectors of Neuronal IdentityCurrent topics in developmental biology 116:455–475.https://doi.org/10.1016/bs.ctdb.2015.12.007
-
Sex differences in molecular neuroscience: from fruit flies to humansNature Reviews Neuroscience 11:9–17.https://doi.org/10.1038/nrn2754
-
LIN-28 and the poly(U) polymerase PUP-2 regulate let-7 microRNA processing in Caenorhabditis elegansNature Structural & Molecular Biology 16:1016–1020.https://doi.org/10.1038/nsmb.1675
-
Mate searching in Caenorhabditis elegans: a genetic model for sex drive in a simple invertebrateJournal of Neuroscience 24:7427–7434.https://doi.org/10.1523/JNEUROSCI.1746-04.2004
-
Reframing sexual differentiation of the brainNature Neuroscience 14:677–683.https://doi.org/10.1038/nn.2834
-
Cell-intrinsic timing in animal developmentWiley Interdisciplinary Reviews: Developmental Biology 3:365–377.https://doi.org/10.1002/wdev.145
-
Distributed effects of biological sex define sex-typical motor behavior in Caenorhabditis elegansJournal of Neuroscience 34:1579–1591.https://doi.org/10.1523/JNEUROSCI.4352-13.2014
-
Genetic variation in LIN28B is associated with the timing of pubertyNature Genetics 41:729–733.https://doi.org/10.1038/ng.382
-
Sexual modulation of sex-shared neurons and circuits in Caenorhabditis elegansJournal of Neuroscience Research 95:527–538.https://doi.org/10.1002/jnr.23912
-
The heterochronic gene lin-29 encodes a zinc finger protein that controls a terminal differentiation event in Caenorhabditis elegansDevelopment 121:2491–2500.
-
Control of developmental timing in animalsNature Reviews Genetics 2:690–701.https://doi.org/10.1038/35088566
-
Reciprocal expression of lin-41 and the microRNAs let-7 and mir-125 during mouse embryogenesisDevelopmental dynamics : an official publication of the American Association of Anatomists 234:1046–1054.https://doi.org/10.1002/dvdy.20599
-
The neural basis of puberty and adolescenceNature Neuroscience 7:1040–1047.https://doi.org/10.1038/nn1326
-
Temporal pattern formation by heterochronic genesAnnual Review of Genetics 31:611–634.https://doi.org/10.1146/annurev.genet.31.1.611
-
The Caenorhabditis elegans male: postembryonic development of nongonadal structuresDevelopmental Biology 78:542–576.https://doi.org/10.1016/0012-1606(80)90352-8
-
Post-embryonic cell lineages of the nematode, Caenorhabditis elegansDevelopmental Biology 56:110–156.https://doi.org/10.1016/0012-1606(77)90158-0
-
The embryonic cell lineage of the nematode Caenorhabditis elegansDevelopmental Biology 100:64–119.https://doi.org/10.1016/0012-1606(83)90201-4
-
The motor circuitInternational review of neurobiology 69:125–167.https://doi.org/10.1016/S0074-7742(05)69005-8
-
MARRVEL: Integration of Human and Model Organism Genetic Resources to Facilitate Functional Annotation of the Human GenomeThe American Journal of Human Genetics 100:843–853.https://doi.org/10.1016/j.ajhg.2017.04.010
-
The structure of the nervous system of the nematode Caenorhabditis elegansPhilosophical Transactions of the Royal Society B: Biological Sciences 314:1–340.https://doi.org/10.1098/rstb.1986.0056
-
The sensory circuitry for sexual attraction in C. elegans malesCurrent Biology 17:1847–1857.https://doi.org/10.1016/j.cub.2007.09.011
-
A database of Caenorhabditis elegans behavioral phenotypesNature Methods 10:877–879.https://doi.org/10.1038/nmeth.2560
-
Mab-3 is a direct tra-1 target gene regulating diverse aspects of C. elegans male sexual development and behaviorDevelopment 127:4469–4480.
-
Similarity of DNA binding and transcriptional regulation by Caenorhabditis elegans MAB-3 and Drosophila Melanogaster DSX suggests conservation of sex determining mechanismsDevelopment 126:873–881.
Article and author information
Author details
Funding
Howard Hughes Medical Institute
- Oliver Hobert
National Institutes of Health (2R37NS039996)
- Oliver Hobert
Schweizerischer Nationalfonds zur Förderung der Wissenschaftlichen Forschung (31003A_163447)
- Helge Großhans
Novartis Research Foundation
- Helge Großhans
The funders had no role in study design, data collection and interpretation, or the decision to submit the work for publication.
Acknowledgements
We thank Qi Chen for generating transgenic strains, Emily Bayer for generating and providing a ceh-14 null allele, Bob Horvitz and Hannes Bülow for providing strains and DNA, and members of the Hobert lab for comments on the manuscript. We thank Iskra Katic, Lan Xu, Jun Liu, Magdalene Rausch and Chiara Azzi for their help in generating strains. This work was supported by the NIH (2R37NS039996 to OH), by a Birch/Derchin Fellowship to CW, by the Swiss National Science Foundation (Grant # 31003A_163447 to HG) and by the Novartis Research Foundation through FMI (to HG) and the Howard Hughes Medical Institute (OH).
Copyright
© 2019, Pereira et al.
This article is distributed under the terms of the Creative Commons Attribution License, which permits unrestricted use and redistribution provided that the original author and source are credited.
Metrics
-
- 4,132
- views
-
- 534
- downloads
-
- 54
- citations
Views, downloads and citations are aggregated across all versions of this paper published by eLife.
Citations by DOI
-
- 54
- citations for umbrella DOI https://doi.org/10.7554/eLife.42078