UBP12 and UBP13 negatively regulate the activity of the ubiquitin-dependent peptidases DA1, DAR1 and DAR2
Abstract
Protein ubiquitination is a very diverse post-translational modification leading to protein degradation or delocalization, or altering protein activity. In Arabidopsis thaliana, two E3 ligases, BIG BROTHER (BB) and DA2, activate the latent peptidases DA1, DAR1 and DAR2 by mono-ubiquitination at multiple sites. Subsequently, these activated peptidases destabilize various positive growth regulators. Here, we show that two ubiquitin-specific proteases, UBP12 and UBP13, deubiquitinate DA1, DAR1 and DAR2, hence reducing their peptidase activity. Overexpression of UBP12 or UBP13 strongly decreased leaf size and cell area, and resulted in lower ploidy levels. Mutants in which UBP12 and UBP13 were downregulated produced smaller leaves that contained fewer and smaller cells. Remarkably, neither UBP12 nor UBP13 were found to be cleavage substrates of the activated DA1. Our results therefore suggest that UBP12 and UBP13 work upstream of DA1, DAR1 and DAR2 to restrict their protease activity and hence fine-tune plant growth and development.
Introduction
Ubiquitination plays a prominent role in the signaling cascades of many plant hormones (Santner and Estelle, 2010), such as auxins (Salehin et al., 2015), jasmonates (Nagels Durand et al., 2016), gibberellins (Wang and Deng, 2011), and strigolactones (Marzec, 2016), but also in many plant developmental processes and responses to stress (Shu and Yang, 2017). Therefore, a very tight control of this process and a high substrate specificity, which is mainly determined by the E3 ubiquitin ligases (Shu and Yang, 2017), are required. The tremendous diversity of the ubiquitination system and its potential in post-translational regulation are illustrated by the presence of more than 1400 genes that encode E3 ligases in Arabidopsis (Vierstra, 2009). Furthermore, there is a high diversity of ubiquitination types and combinations with other post-translational modifications (PTMs) (Callis, 2014; Swatek and Komander, 2016), as well as of the fate of the ubiquitinated protein, such as degradation, delocalization or changes in activity (Swatek and Komander, 2016).
In contrast to the more intensively studied action of E3 ligases, insights into the specific roles of deubiquitination enzymes (DUBs) in plant growth and development are only recently emerging. DUBs can generate free ubiquitin from tandem-linear repeats (Callis et al., 1995; Callis et al., 1990), are able to trim ubiquitin chains by hydrolyzing the isopeptide bond between ubiquitin molecules, and can remove covalently bound ubiquitin from proteins (Komander et al., 2009). The Arabidopsis genome codes for around 50 DUBs. As in yeast and mammals, they can be divided into five classes: the ubiquitin C-terminal hydrolases (UHCs), JAB1/MPN/MOV34 (JAMM) domain DUBs that are zinc metalloproteases, ovarian tumor proteases, the Machado-Josephin domain (MJD) DUBs, and the ubiquitin binding proteins (UBPs), which is the largest group (Isono and Nagel, 2014). All UBPs contain specific catalytic Cys- and His-boxes, which are highly conserved in both sequence and length (Zhou et al., 2017). Based on their sequence homology and protein domain organization, these 27 members can be further divided into 14 subfamilies (Yan et al., 2000). UBP12 and UBP13 are the largest UBPs and contain a unique meprin and TRAF homology (MATH) domain. They were first reported to be functional deubiquitinating enzymes that negatively regulate plant immunity (Ewan et al., 2011). Since then, both proteins have been described to be involved in diverse molecular pathways. Mutations in UBP12 and UBP13 result in early flowering and a decreased periodicity of circadian rhythm (Cui et al., 2013). Molecularly, GIGANTEA (GI) recruits UBP12 and UBP13 to the ZEITLUPE (ZTL) photoreceptor complex, which antagonizes the E3 ligase activity of ZTL and hereby stabilizes GI, ZTL and TOC1 [TIMING OF CAB (CHLOROPHYLL A/B-BINDING PROTEIN EXPRESSION) 1] protein levels (Lee et al., 2019). In addition, UBP12 and UBP13 can regulate the expression of several genes by deubiquitinating ubiquitinated H2A (H2Aub) upon associating with LIKE HETEROCHROMATIN PROTEIN 1, a plant-specific polycomb group (PcG) protein (Derkacheva et al., 2016). Polyubiquitination of MYC2 by the PUB10 E3 ligase can be counteracted by UBP12 and UBP13, preventing degradation of MYC2 by the 26S proteasome which then activates jasmonic acid signaling (Jeong et al., 2017). In a similar manner, ROOT GROWTH FACTOR RECEPTOR 1 (RGFR1) and ORESARA 1 (ORE1) are deubiquitinated and therefore stabilized by UBP12 and UBP13, leading to an increased root sensitivity to the RGF1 peptide hormone (An et al., 2018) and an acceleration in leaf senescence (Park et al., 2019), respectively.
Mutations in UBP12 or UBP13 decrease rosette leaf number and double mutants display severe developmental defects (Cui et al., 2013). However, a direct link between these deubiquitinating enzymes and leaf growth and development remains elusive. Here, we found that UBP12 and UBP13 interact with DA1, DAR1 and DAR2 in vivo. DA1, DAR1 and DAR2 have previously been documented to negatively regulate leaf growth. Upon multiple mono-ubiquitination by BIG BROTHER (BB) or DA2, these latent peptidases are activated to cleave growth regulators, such as UBP15, TCP14, TCP15 and TCP22 (Dong et al., 2017). In addition, the activating E3 ligases BB and DA2 are cleaved and BB is subsequently degraded by the N-degron pathway, mediated by PROTEOLYSIS 1 (PRT1) (Dong et al., 2017). Single knock-outs in DA1, DAR1 and DAR2 only have very subtle effects on organ size (Dong et al., 2017; Li et al., 2008). Plant growth is however strongly enhanced in the double mutant da1ko_dar1-1, comparable to da1-1 mutants, which carry a point mutation (DA1R358K) (Li et al., 2008). The latter mutation has a dominant-negative action towards DA1 and DAR1 (Li et al., 2008) and causes a reduction in peptidase activity (Dong et al., 2017). Rosette growth is however severely impaired in the triple mutant da1ko_dar1-1_dar2-1, and the size of leaf cells and the extent of endoreduplication are reduced (Peng et al., 2015). This phenotype can be complemented by ectopic expression of DA1, DAR1 or DAR2, suggesting they work redundantly in leaves (Peng et al., 2015). On the other hand, overexpression of GFP-DA1 results in smaller organs with fewer cells (Vanhaeren et al., 2017). Mutants of UBP15 can abolish the da1-1 phenotype and give rise to smaller organs (Du et al., 2014). Inversely, ectopic expression of UBP15 enhances growth (Du et al., 2014; Liu et al., 2008).
Here, we demonstrate that UBP12 and UBP13 not only bind DA1, DAR1 and DAR2, but can also remove ubiquitin from these proteins, rendering them in an inactive state. Moreover, UBP12 and UBP13 were not found to be proteolytically cleaved by DA1, DAR1 or DAR2, indicating they work upstream in this pathway. In line with these findings, UBP12 and UBP13 mutants and overexpression lines exhibit macroscopic, cellular and molecular phenotypes overlapping with those of 35S::GFP-DA1 overexpression lines and da1ko_dar1-1_dar2-1 mutants, respectively. Our data provide evidence for a pivotal role of UBP12 and UBP13 in restricting the protease activity of DA1, DAR1 and DAR2 during plant growth and development.
Results
DA1, DAR1 and DAR2 interact with UBP12 and UBP13 in vivo
Genetic modifier screens previously identified several interactors of DA1 that either activate its peptidase activity or are subjected to proteolytic cleavage by DA1. To gain further insights into the DA1 growth-regulatory pathway, we generated Arabidopsis lines that overexpressed GFP-tagged fusion proteins of DA1, DAR1 and DAR2. Total protein extracts were isolated from eight-day-old seedlings and incubated with anti-GFP beads to purify the bait proteins and their interactors. Label free quantification identified a significant enrichment (p-value<0.01) of the UBIQUITIN-SPECIFIC PROTEASE 12 (UBP12) and UBP13 in the GFP-DA1 and GFP-DAR1 samples (Figure 1A–B) among other interaction candidates (Figure 1—figure supplement 1, Figure 1—source data 1). Despite the much lower levels of DAR2 MS/MS counts and a lower DAR2 bait protein coverage (Figure 1—figure supplements 2–3), we found a significant enrichment of UBP12 at a less stringent threshold (p-value<0.05) and UBP13 at the border of significance (Figure 1C, Figure 1—source data 1). To confirm this interaction, we performed an in vitro pull-down using UBP12 and UBP13 as bait proteins. DA1, DAR1 and DAR2 were expressed and isolated as HIS-MBP fusion proteins and UBP12 and UBP13 as GST fusion proteins; free GST was used as a negative control. We incubated equal amounts of free GST, full-length GST-UBP12 and GST-UBP13 with HIS-MBP-DA1, HIS-MBP-DAR1 and HIS-MBP DAR2. Western blot analysis after purification with anti-GST beads showed that HIS-MBP-DA1, HIS-MBP-DAR1 and HIS-MBP-DAR2 could be co-purified with either GST-UBP12 or GST-UBP13, but not with free GST (Figure 1D–F). Additionally, we co-expressed RFP-DA1, RFP-DAR1 and RFP-DAR2 with either GFP-UBP12 or free GFP from the same vector in Arabidopsis cell suspension cultures. Again, we could confirm the interaction between GFP-UBP12 and RFP-DA1, RFP-DAR1 or RFP-DAR2 (Figure 1G).
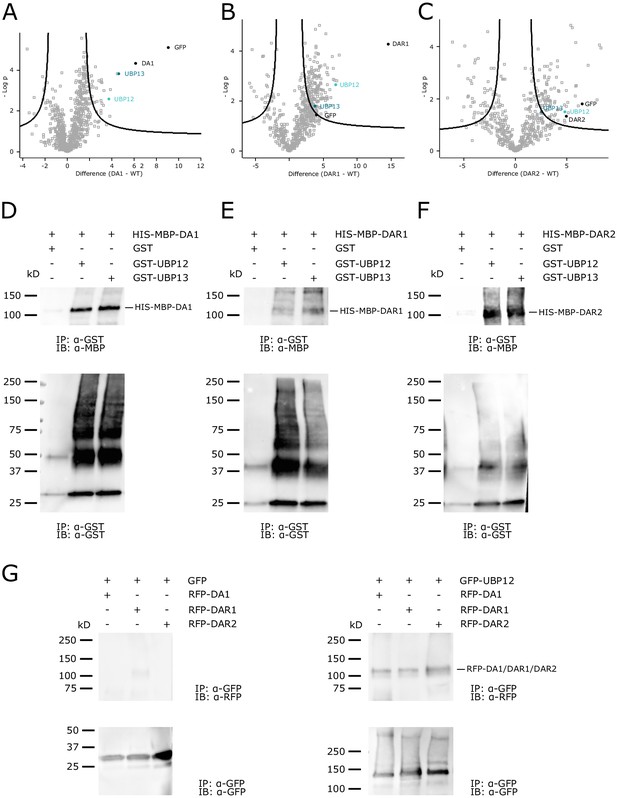
UBP12 and UBP13 interact with DA1, DAR1 and DAR2.
(A–C) Enrichment of the bait, UBP12 and UBP13 compared to the control after immunoprecipitation in (A) 35S::GFP-DA1 (FDR = 0.01, S0 = 1, permutation-based FDR-corrected t-test, Figure 1—source data 1), (B) 35S::GFP-DAR1 (FDR = 0.01, S0 = 1, permutation-based FDR-corrected t-test, Figure 1—source data 1) or (C) 35S::GFP-DAR2 (FDR = 0.05, S0 = 1, permutation-based FDR-corrected t-test, Figure 1—source data 1) seedlings. (D–F) In vitro pull down of (D) HIS-MBP-DA1, (E) HIS-MBP-DAR1 and (F) HIS-MBP-DAR2 with free GST, GST-UBP12 and GST-UBP13. (G) In vivo pull-down of RFP-DA1, RFP-DAR1 and RFP-DAR2 with free GFP and GFP-UBP12.
-
Figure 1—source data 1
List of DA1, DAR1, DAR2 interactors and LFQ intensities by MS/MS.
- https://cdn.elifesciences.org/articles/52276/elife-52276-fig1-data1-v2.xlsx
-
Figure 1—source data 2
MS/MS counts of DA1, DAR1 and DAR2; protein coverage of DA1, DAR1 and DAR2; relative expression levels of DA1, DAR1, DAR2, UBP12 and UBP13 during leaf development.
- https://cdn.elifesciences.org/articles/52276/elife-52276-fig1-data2-v2.xlsx
Next, we measured the expression levels of DA1, DAR1, DAR2, UBP12 and UBP13 in isolated wild-type (Col-0) leaves in a detailed time-course from leaf primordium initiation to maturity. Both UBP12 and UBP13 are highly and evenly expressed throughout leaf development at comparable levels (Figure 1—figure supplement 4). Also DA1, DAR1 and DAR2 are expressed during leaf development as demonstrated before (Peng et al., 2015), albeit DAR2 at a lower level (Figure 1—figure supplement 4). Previous research showed that UBP12 and UBP13 are localized in the nucleus and cytoplasm (Cui et al., 2013). Additional transient expression of GFP-UBP12 or GFP-UBP13 in Nicotiana benthamiana leaves demonstrated that these proteins co-localize in the cytoplasm and the nucleus with RFP-DA1, RFP-DAR1 and RFP-DAR2 (Figure 1—figure supplements 5–10). Taken together, UBP12 and UBP13 co-localize with DA1, DAR1 and DAR2, are co-expressed during leaf development and interact with DA1, DAR1 and DAR2 in vivo and in vitro.
Miss-expression of UBP12 and UBP13 alters leaf size
Because DA1, DAR1 and DAR2 are known to restrict plant organ growth, we examined the role of UBP12 and UBP13 in regulating leaf size by generating several independent transgenic 35S::UBP12 and 35S::UBP13 lines. All overexpression lines showed a reduction in rosette area (Figure 2A). In addition, leaves appeared to be rounder than those of Col-0, a phenotype that can also be observed in da1-1 plants, and the petioles were found to be shorter. To quantify this, we measured the leaf blade height and width of Col-0, da1-1, 35S::UBP12 and 35S::UBP13 plants and calculated the leaf blade index, which is the ratio of leaf height to width and hence a measure of leaf shape (Tsukaya, 2002). We could observe a significantly lower leaf blade index in da1-1 mutants (Figure 2—figure supplement 1) and in two independent 35S::UBP12 and 35S::UBP13 lines compared to Col-0 (Figure 2—figure supplement 2), meaning the leaves had a rounder shape. For several 35S::UBP13 lines, we were unable to produce stable seed stocks because the homozygous plants were stunted in growth (Figure 2A) and failed to produce a flower stalk and seeds. We continued with two independent lines for further phenotypic analysis: two homozygous UBP12 lines (35S::UBP12_3.1 and 35S::UBP12_3.2) and two UBP13 lines from which heterozygous plants were selected (35S::UBP13_1.1 and 35S::UBP13_2.3) (Figure 2A). These lines all had a significant increase in their respective transgene expression compared to Col-0 (Figure 2—figure supplement 3).
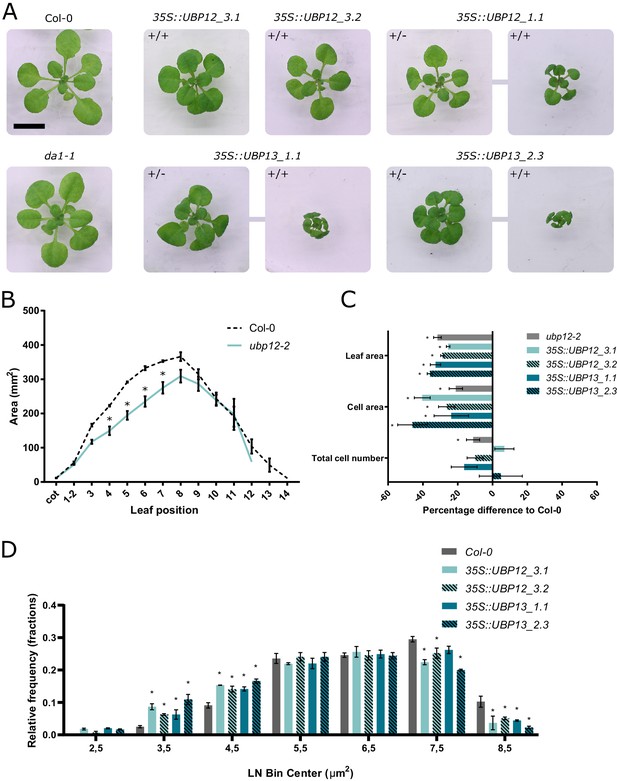
Regulation of leaf size by UBP12 and UBP13.
(A) Twenty-one-day-old plants of Col-0, da1-1 and homozygous (+/+) or heterozygous (+/-) UBP12 and UBP13 overexpression lines. Scale bar represents 1 cm, homozygous and heterozygous plants of the same single locus line are linked in the figure. (B) Leaf area measurements of Col-0 and ubp12-2, n = 3 biological repeats with >10 plants per repeat. (C) Percentage differences of leaf area, cell area and cell number of ubp12-2, 35S::UBP12_3.1, 35S::UBP12_3.2, 35S::UBP13_1.1 and 35S::UBP13_3.2 compared to Col-0, n = 3 biological repeats with three representative leaves per repeat. (D) Relative frequencies of LN transformed cell area distribution of Col-0, 35S::UBP12_3.1, 35S::UBP12_3.2, 35S::UBP13_1.1 and 35S::UBP13_3.2 in 1-µm2 bin sizes, n = 3 biological repeats with three representative leaves per repeat. Bars represent the SEM; * indicates p-value<0.05, ANOVA (Figure 2—source data 1).
-
Figure 2—source data 1
Leaf area analysis and statistics of Col-0 and ubp12-2; leaf area analysis and statistics of Col-0, 35S::UBP12_3.1, 35S::UBP12_3.2, 35S::UBP13_1.1 and 35S::UBP13_3.2; cellular analysis and statistics of Col-0, 35S::UBP12_3.1, 35S::UBP12_3.2, 35S::UBP13_1.1 and 35S::UBP13_3.2; cellular analysis of ubp12-2, relative frequency of the pavement cell area and statistics of Col-0, 35S::UBP12_3.1, 35S::UBP12_3.2, 35S::UBP13_1.1 and 35S::UBP13_3.2.
- https://cdn.elifesciences.org/articles/52276/elife-52276-fig2-data1-v2.xlsx
-
Figure 2—source data 2
Q-RT-PCR data and statistics of UBP12 and UBP13 expression in Col-0, da1-1, 35S::UBP12_3.1, 35S::UBP12_3.2, 35S::UBP13_1.1 and 35S::UBP13_3.2 overexpression lines; leaf area data and statistics of ubp12-1; leaf area data and statistics of ubp13-1; leaf area data and statistics of ubp13-2; leaf area data and statistics of ubp13-3; relative frequency of ubp12-2 pavement cell area data and statistics.
- https://cdn.elifesciences.org/articles/52276/elife-52276-fig2-data2-v2.xlsx
In parallel, we screened two independent UBP12 T-DNA insertion lines [ubp12-1 (GABI_244E11) and ubp12-2 (GABI_742C10)] and three independent UBP13 T-DNA lines [ubp13-1 (SALK_128312), ubp13-2 (SALK_024054) and ubp13-3 (SALK_132368)]. After leaf area measurements, we could observe a decrease in leaf area in the ubp12-2 mutants (Figure 2B), in which the levels of both UBP12 and UBP13 transcripts were previously shown to be downregulated (Cui et al., 2013). Mutant lines in which only the expression of either UBP12 or UBP13 was downregulated, displayed no differences in leaf size compared to the control (Figure 2—figure supplements 4–7).
The final leaf size is determined by cell proliferation and cell expansion. To identify which of these processes were affected in the ubp12-2 mutant and in the UBP12 and UBP13 overexpression lines, we performed a cellular analysis on the abaxial pavement cells of mature leaves. In all overexpression lines, the significant decrease in leaf area (29%, 26%, 33% and 36% for 35S::UBP12_3.1, 35S::UBP12_3.2, 35S::UBP13_1.1 and 35S::UBP13_2.3, respectively) was caused by a strong reduction in cell area (26%, 40%, 24% and 46% for 35S::UBP12_3.1, 35S::UBP12_3.2, 35S::UBP13_1.1 and 35S::UBP13_2.3, respectively), whereas the decrease in ubp12-2 leaf size (32%) resulted from a reduction in cell area (21%) and cell number (11%) (Figure 2C). Remarkably, besides a general decrease in pavement cell area, we could observe a larger proportion of very small cells in the UBP12 and UBP13 overexpression lines (Figure 2—figure supplement 8), which was even more pronounced in homozygous 35S::UBP13_1.1 plants (Figure 2—figure supplement 9). A cell area distribution plot confirmed that indeed all independent UBP12 and UBP13 overexpression lines harbored a larger proportion of these small cells in addition to a general decrease in mature pavement cell size (Figure 2D). For the ubp12-2 mutant, no differences in cell area distribution compared to Col-0 could be observed (Figure 2—figure supplement 10).
Overexpression of UBP12 or UBP13 delays the onset of endoreduplication and cell differentiation in leaves
A strong reduction in cell size is often correlated with decreased levels of endoreduplication. To explore this into more detail, we harvested a time-course of the first leaf pair of Col-0, 35S::UBP12_3.1 and 35S::UBP13_1.1 (hereafter referred to as 35S::UBP12 and 35S::UBP13) plants, spanning all major developmental time points. At nine days after stratification (DAS), all leaf cells of the three lines exhibited a 2C or 4C content, demonstrating the majority of cells are still in the mitotic cell cycle (Figure 3A). From 12 DAS onwards, cells with 8C started to appear, indicating the onset of endoreduplication. At 12 DAS, leaves of 35S::UBP12 plants contained a significantly larger proportion of 2 C cells (53%) than those of the Col-0 (43%) and a significantly lower amount of 4 C cells (44% and 52%, respectively). At 15 DAS, the amount of 8C nuclei was significantly lower in 35S::UBP12 (28%) than in the control (35%) (Figure 3A). Similar, but more pronounced observations were found in 35S::UBP13 leaves, in which a significantly higher amount of 2 C cells was detected at 12 DAS (61%) and 15 DAS (42%) compared to Col-0 (43% and 25%, respectively). In addition, a lower level of 4 C cells was found at 12 DAS (35% in 35S::UBP13, 52% in Col-0) and fewer cells with 8C were present at 15, 18, 21 and 27 DAS in 35S::UBP13 (22%, 33%, 35% and 35%, respectively) compared to Col-0 (35%, 42%, 42% and 46%, respectively) (Figure 3A). An alternative way to illustrate endoreduplication levels is the endoreduplication index, representing the average amount of endocycles a nucleus underwent. Generally, slightly lower endoreduplication levels could be observed in 35S::UBP12 leaves (Figure 3—figure supplement 1), whereas a stronger effect was clear in 35S::UBP13 leaves, with significant differences at 12, 15 and 21 DAS (Figure 3—figure supplement 2).
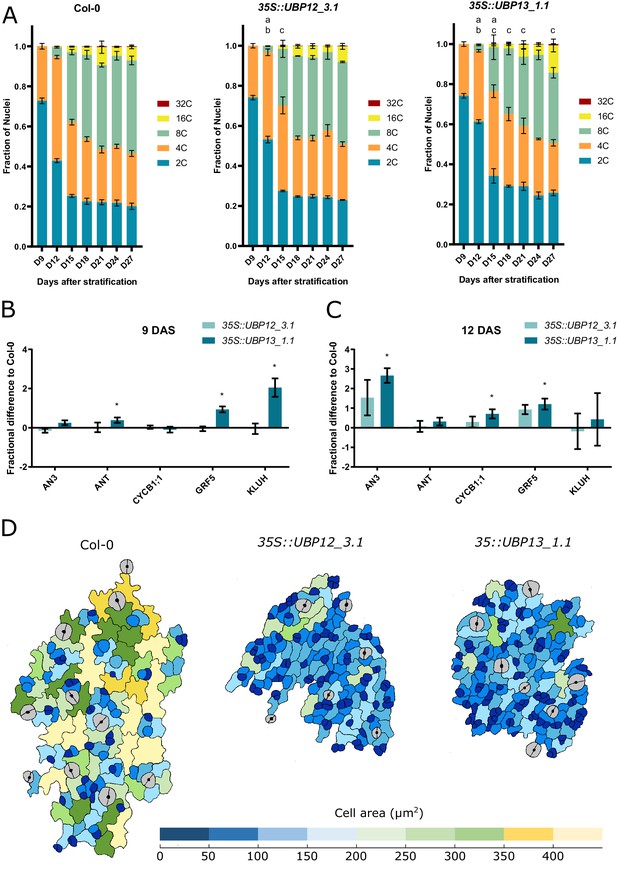
UBP12 and UBP13 regulate the onset of endoreduplication.
(A) Ploidy distribution of nuclear DNA in Col-0, 35S::UBP12_3.1 and 35S::UBP13_1.1. (B–C) Fractional difference in expression of cell proliferation markers in 35S::UBP12_3.1 and 35S::UBP13_1.1 compared to Col-0 at (B) nine and (C) 12 DAS. (D) Representations of abaxial leaf epidermal cells at the tip of the third leaf of Col-0, 35S::UBP12_3.1 and 35S::UBP13_1.1 at 12 DAS. Bars represent the SEM, n = 3 biological repeats with >3 leaves per repeat; a, b and c indicate a significant difference in 2C, 4C and 8C, respectively; * indicates p-value<0.05, ANOVA, (Figure 3—source data 1).
-
Figure 3—source data 1
Flow cytometry counts and statistics; Q-RT-PCR data and statistics of proliferation markers in developing leaves (9 DAS and 12 DAS).
- https://cdn.elifesciences.org/articles/52276/elife-52276-fig3-data1-v2.xlsx
-
Figure 3—source data 2
Endoreduplication index calculations and statistics; Q-RT-PCR data and statistics of proliferation markers in developing leaves (15 DAS and 18 DAS).
- https://cdn.elifesciences.org/articles/52276/elife-52276-fig3-data2-v2.xlsx
Because the strongest effects were visible in the younger time points, we subsequently prepared RNA from the younger third leaf until 18 DAS. Considering the higher 2C levels in 35S::UBP12 and 35S::UBP13 (Figure 3A) and the presence of larger proportions of small cells in the leaf epidermis at 21 DAS (Figure 2D, Figure 2—figure supplements 8–9), we measured the expression of several genes that are highly expressed during cell proliferation and whose expression goes down during the transition to differentiation and endoreduplication in leaves: ANGUSTIFOLIA3 (AN3) (Horiguchi et al., 2005), AINTEGUMENTA (ANT), (Mizukami and Fischer, 2000), GROWTH-REGULATING FACTOR 5 (GRF5) (Horiguchi et al., 2005; Vercruyssen et al., 2015), KLUH (Anastasiou et al., 2007) and the cell division marker CYCLINB1;1 (CYCB1;1) (Doerner et al., 1996; Shaul et al., 1996). At 9 DAS, when all epidermal cells are actively dividing (Andriankaja et al., 2012), we could observe a significantly higher expression of ANT, GRF5 and KLUH in 35S::UBP13 lines compared to the Col-0 (Figure 3B). Later in development at 12 DAS, a large portion of the Col-0 cells should have exited the mitotic division cycle and start to differentiate (Andriankaja et al., 2012; Donnelly et al., 1999; Kazama et al., 2010). Still, there was a significant higher expression of AN3 and GRF5 and the cell division marker CYCB1;1 in 35S::UBP13 leaves (Figure 3C). On 15 DAS, the expression levels of ANT and CYCB1;one were elevated in 35S::UBP13 leaves (Figure 3—figure supplement 3) and finally, at 18 DAS, ANT was still significantly higher expressed in 35S::UBP13 compared to the Col-0 (Figure 3—figure supplement 4). To further explore these observations, we characterized the epidermal cells of tips of the third leaf of 12 day old seedlings, which should be undergoing cell differentiation at this stage in Col-0 (Andriankaja et al., 2012). In Col-0 leaf tips, we could indeed observe that the epidermal cells had started to expand and differentiate (Figure 3D). On the contrary, similar leaf tips of 35S::UBP12 or 35S::UBP13 plants contained almost exclusively undifferentiated cells (Figure 3D). These results, together with our observation that cell number was not significantly altered in UBP12 an UBP13 overexpression lines, indicate that especially during the early developmental stages of leaf growth, endoreduplication and cell differentiation are delayed in 35S::UBP12 and 35S::UBP13 leaves.
UBP12 and UBP13 can deubiquitinate activated DA1, DAR1 and DAR2
The latent peptidases DA1, DAR1 and DAR2 can be activated upon ubiquitination by the E3 ligases BB or DA2. Considering the enzymatic function of UBP12 and UBP13, they might counteract this by deubiquitinating these activated peptidases. To test this, we performed an in vitro ubiquitination assay to generate ubiquitinated DA1, DAR1 an DAR2, followed by a deubiquitination step with UBP12 or UBP13, or their respective catalytic mutants, UBP12C208S and UBP13C207S (Cui et al., 2013; Ewan et al., 2011). DA1, DAR1 and DAR2 were expressed and isolated as HIS-MBP fusion proteins and UBP12, UBP13, UBP12C208S and UBP13C207S as GST fusion proteins. To mediate the ubiquitination, recombinant HIS-DA2 was purified as the E3 ligase. The ubiquitinated peptidases were incubated with equal amounts of either GST-UBP12, GST-UBP12C208S, GST-UBP13 or GST-UBP13C207S. Figure 4A shows that ubiquitinated HIS-MBP-DA1 can be deubiquitinated by GST-UBP12 or GST-UBP13, but their respective catalytic mutants fail to do so. Similar deubiquitination activities could be observed for the substrates DAR1 and DAR2 (Figure 4B–C). Because the peptidase activity of DA1, DAR1 and DAR2 is similarly activated upon ubiquitination and they function redundantly to regulate leaf size and ploidy, we chose to focus on DA1 in our next experiments.
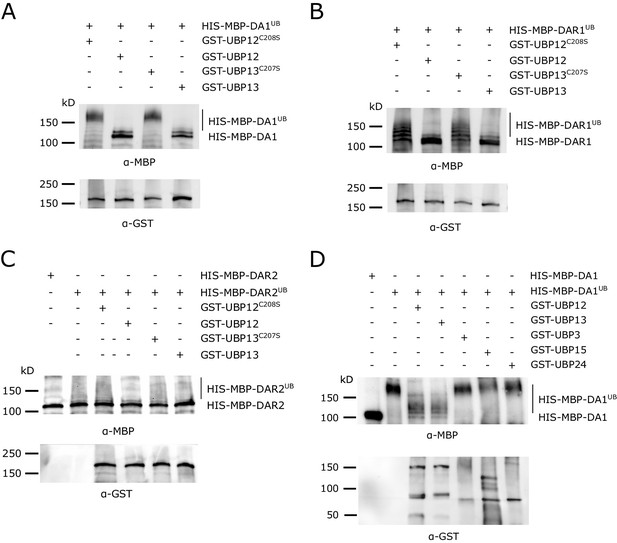
In vitro deubiquitination specificity of DA1, DAR1 and DAR2 by UBP12 and UBP13.
(A–C) In vitro deubiquitination assays with GST-UBP12, GST-UBP12C208S, GST-UBP13 or GST-UBP13C207S of (A) HIS-MBP-DA1, (B) HIS-MBP-DAR1 and (C) HIS-MBP-DAR2. (D) Deubiquitination assay with GST-UBP12, GST-UBP13, GST-UBP3, GST-UBP15 and GST-UBP24 of HIS-MBP-DA1.
To demonstrate the specificity of UBP12 and UBP13, we performed additional deubiquitination experiments with several UBPs from different subfamilies (Yan et al., 2000): GST-UBP3, GST-UBP24 and GST-UBP15, of which the latter had already been demonstrated to function in the same pathway of DA1 (Du et al., 2014) as its cleavage substrate (Dong et al., 2017). Ubiquitinated HIS-MBP-DA1 was incubated with equal amounts of GST-UBP12, GST-UBP13, GST-UBP3, GST-UBP15 and GST-UBP24. We found again that both GST-UBP12 and GST-UBP13 could strongly deubiquitinate HIS-MBP-DA1, but no such effect could be observed upon addition of GST-UBP3, GST-UBP15 or GST-UBP24 (Figure 4D).
To further consolidate these observations, we evaluated the effect of different in vivo levels of UBP12 and UBP13 on the ubiquitination status of DA1. To realize this, we produced ubiquitinated DA1 in vitro and incubated it with equal amounts of cell-free extracts of Col-0 and ubp12-2 seedlings, the latter containing lower protein levels of UBP12 and UBP13. Already after 1 hr, we could observe a shift in molecular weight in the ubiquitinated HIS-MBP-DA1 samples that were incubated with Col-0 extract (Figure 5A), and this shift did not occur in the sample that was incubated with the ubp12-2 extract (Figure 5B). In addition, the intensity of ubiquitinated HIS-MBP-DA1 bands faded faster in the presence of Col-0 extract than in the presence of ubp12-2 extract (Figure 5A–C). We performed a similar experiment with extracts of 35S::UBP12 and 35S::UBP13 plants, which contain higher levels of UBP12 or UBP13 proteins respectively. First, we could confirm the faster loss in molecular weight and intensity of ubiquitinated HIS-MBP-DA1 in Col-0 (Figure 5D) than in ubp12-2 after 2 hr and 4 hr of incubation (Figure 5E). In addition, when ubiquitinated DA1 proteins were incubated with equal extracts of 35S::UBP12 (Figure 5F) or 35S::UBP13 (Figure 5G) plants, the intensity of ubiquitinated HIS-MBP-DA1 disappeared at a faster rate (Figure 5D–H). At 6 hr or 8 hr of incubation, no HIS-MBP-DA1 bands could be distinguished anymore from the background, these results could therefore not be interpreted (Figure 5D–H). In addition, we extracted proteins of 35S::GFP-DA1, 35S::GFP-DA1_35S::UBP12 and 35S::GFP-DA1_35S::UBP13 plants and performed a purification with anti-GFP beads from equal protein inputs. After purification, we submitted the samples to Western Blot and detected the abundance of GFP-DA1 proteins. We could not identify increases in protein abundance in the 35S::GFP-DA1_35S::UBP12 and 35S::GFP-DA1_35S::UBP13 samples compared to those of 35S::GFP-DA1 (Figure 5—figure supplement 1), suggesting UBP12 and UBP13 do not prevent potential removal of polyubiquitination of DA1 and hereby prevent its degradation by the proteasome.
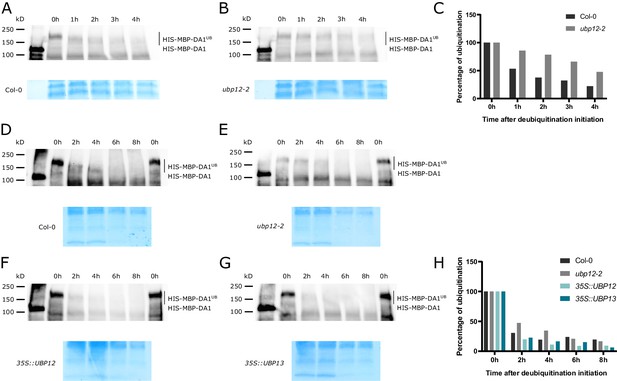
Deubiquitination of DA1, DAR1 and DAR2 by UBP12 and UBP13 in vivo.
(A–B) Cell-free deubiquitination assay of ubiquitinated HIS-MBP-DA1 proteins incubated with (A) Col-0 or (B) ubp12-2 extract. (C) Quantification of deubiquitination (Figure 5—source data 1). (D–G) Cell-free deubiquitination assay of ubiquitinated HIS-MBP-DA1 with (D) Col-0, (E) ubp12-2, (F) 35S::UBP12 or (G) 35S::UBP13 extracts. (H) Quantification of deubiquitination (Figure 5—source data 1).
-
Figure 5—source data 1
Calculation of in vivo deubiquitination.
- https://cdn.elifesciences.org/articles/52276/elife-52276-fig5-data1-v2.xlsx
These experiments demonstrate the deubiquitination specificity by UBP12 and UBP13 in vitro and the positive effects of high in vivo UBP12 or UBP13 protein levels on the deubiquitination of DA1. In addition, high levels of UBP12 or UBP13 do not seem to affect the stability of DA1 in vivo.
UBP12 and UBP13 are not substrates of the activated DA1
Our observation that UBP12 and UBP13 can deubiquitinate DA1, DAR1 and DAR2 suggests they work upstream in this growth-regulatory pathway. Considering the peptidase activity of DA1, DAR1 and DAR2, we tested whether these deubiquitinating enzymes could in their turn be substrates. For this purpose, we incubated GST-UBP12, GST-UBP12C208S, GST-UBP13 and GST-UBP13C207S with ubiquitinated HIS-MBP-DA1 or the peptidase-deficient HIS-MBP-DA1H418A,H422A (Dong et al., 2017). The catalytic UBP mutants were added to the assay because they are unable to deubiquitinate DA1 (Figure 4A) and, hence, are exposed for a longer time to the activated peptidase. However, after 4 hr of incubation, the intensities of all GST-tagged UBP proteins were equal and no additional cleaving fragments could be observed in the HIS-MBP-DA1 samples compared to those with HIS-MBP-DA1H418A,H422A (Figure 6A). Similar results were observed in the reactions in which the catalytic mutants of UBP12 or UBP13 were incubated with ubiquitinated HIS-MBP-DA1 or HIS-MBP-DA1H418A,H422A (Figure 6A).
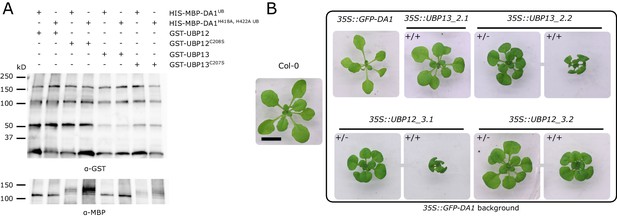
Cleaving assay on UBP12 and UBP13.
(A) In vitro cleaving assay on GST-UBP12, GST-UBP12C208S, GST-UBP13 and GST-UBP13C207S by HIS-MBP-DA1 and the peptidase-deficient HIS-MBP-DA1H418A,H422A. (B) Twenty-one-day-old plants of Col-0, 35S::GFP-DA1, 35S::UBP12_3.1/35S::GFP-DA1, 35S::UBP12_3.2/35S::GFP-DA1, 35S::UBP13_2.1/35S::GFP-DA1 and 35S::UBP13_2.2/35S::GFP-DA1 homozygous (+/+) and heterozygous (+/-) double overexpression lines. Scale bar represents 1 cm.
-
Figure 6—source data 1
Q-RT-PCR data and statistics of DA1, UBP12 and UBP13 expression in Col-0, 35S::GFP-DA1, 35S::UBP12_3.1/35S::GFP-DA1, 35S::UBP12_3.2/35S::GFP-DA1, 35S::UBP13_2.1/35S::GFP-DA1 and 35S::UBP13_2.2/35S::GFP-DA1.
- https://cdn.elifesciences.org/articles/52276/elife-52276-fig6-data1-v2.xlsx
Previously, it has been demonstrated that the dwarfed phenotype of strong BB overexpression lines could largely be rescued by ectopic co-expression of DA1, as a result of the cleavage and destabilization of BB proteins (Dong et al., 2017). Similarly, we generated double overexpression lines of 35S::GFP-DA1 and 35S::UBP12 or 35S::UBP13 (Figure 6—figure supplement 1). Compared to Col-0, leaf areas were reduced in 35S::GFP-DA1 (Figure 6B), as described before (Vanhaeren et al., 2017). In all double overexpression lines, we could observe similar phenotypes as in the 35S::UBP12 and 35S::UBP13 lines in the Col-0 background (Figure 6B, Figure 2A), suggesting that the UBP12 and UBP13 overexpression phenotype is dominant and UBP12 and UBP13 are not destabilized by DA1.
Thus, our biochemical, genetic and phenotypic analyses show that UBP12 and UBP13 are not cleavage substrates, but act upstream of DA1, DAR1 and DAR2 to counteract their ubiquitination. By limiting their peptidase activity, UBP12 and UBP13 fine-tune leaf growth, cell size and endoreduplication.
Discussion
Ubiquitination is an important post-transcriptional modification that comes in various forms of complexity, which can lead to diverse effects on the fate of the substrate protein (Callis, 2014; Swatek and Komander, 2016). The latent peptidases DA1, DAR1 and DAR2 are activated upon multiple mono-ubiquitinations by BB and DA2 and can subsequently cleave several growth regulators (Dong et al., 2017). In this study, we identified two ubiquitin-specific proteases, UBP12 an UBP13, that interact with DA1, DAR1 and DAR2 in vitro and in vivo. Our experiments demonstrate that these UBPs work antagonistically to BB and DA2. Incubation of ubiquitinated DA1, DAR1 or DAR2 with either UBP12 or UBP13 resulted in strong deubiquitination. Interestingly, our in vitro immunoprecipitation (IP) shows that UBP12 and UBP13 can also interact with the unubiquitinated forms of DA1, DAR1 and DAR2. Similar deubiquitination experiments with other UBPs, such as UBP3, UBP15 and UBP24, demonstrated that this activity was specific for UBP12 and UBP13. Interestingly, UBP15 has already been shown to genetically interact with DA1 (Du et al., 2014) and UBP15 proteins can be cleaved by activated DA1 peptidases (Dong et al., 2017). Our results indicate that UBP15 has no deubiquitination activity towards DA1 and acts therefore downstream in this pathway. On the other hand, UBP12 and UBP13 are not substrates of DA1, unlike UBP15 (Dong et al., 2017; Du et al., 2014), which suggests they work more upstream in this signaling cascade. Most probably, UBP15 deubiquitinates and alters the fate of other downstream growth-regulating proteins. UBP12 and UBP13 have recently been described to deubiquitinate various poly-ubiquitinated proteins, preventing their proteasomal degradation (An et al., 2018; Jeong et al., 2017; Lee et al., 2019; Park et al., 2019). In addition, they can decrease the levels of mono-ubiquitinated H2A (Derkacheva et al., 2016) and remove multiple mono-ubiquitinations from DA1, DAR1 and DAR2 as described here. This demonstrates the flexibility of these UBPs towards different types of ubiquitination (Clague et al., 2019). Here, we could not find evidence that besides the removal of the multiple mono-ubiquitination sites of DA1, DAR1 and DAR2, potential degradation of these proteins by removal of poly-ubiquitination was mediated by UBP12 or UBP13.
In contrast to the early flowering time-related genes (Cui et al., 2013), downregulation of either UBP12 or UBP13 did not alter leaf size, indicating that these genes work redundantly in controlling leaf size. The leaf area was only reduced in ubp12-2 mutants, in which levels of both UBP12 and UBP13 are decreased (Cui et al., 2013). The subsequently decreased deubiquitination activity towards DA1, DAR1 and DAR2 could result in an accumulation of the activated peptidases in ubp12-2 mutants, leading to a decrease in cell number and leaf area. A similar phenotype is also observed in GFP-DA1 overexpressing plants (Vanhaeren et al., 2017) and in the ubp15 mutant (Du et al., 2014; Liu et al., 2008). In contrast, high ectopic expression of either UBP12 or UBP13 would result in very low levels of ubiquitinated DA1, DAR1 and DAR2, leading to a severe disturbance of leaf development. Indeed, 35S::UBP12 and 35S::UBP13 plants were strongly reduced in growth in a similar manner as da1ko_dar1-1_dar2-1 triple mutants (Peng et al., 2015). A more detailed cellular and molecular analysis of 35S::UBP12 and 35S::UBP13 leaves revealed more parallels, such as a strong reduction in cell area and a decrease in ploidy levels at the early stages of leaf development, which were also observed in da1ko_dar1-1_dar2-1 plants (Peng et al., 2015). The complete absence of DA1, DAR1 and DAR2 proteins in da1ko_dar1-1_dar2-1 plants could however explain its stronger reduction in endoreduplication than that of UBP12 and UBP13 overexpression lines, in which ubiquitination of DA1, DAR1 and DAR2 can still occur, but is likely kept at a very low level. In addition, several markers of cell proliferation were found to be more highly expressed in 35S::UBP12 and 35S::UBP13 during early stages of leaf development, similarly as observed in plants that contain a DA1R358K mutation (Vanhaeren et al., 2017), which reduces the peptidase activity of DA1 (Dong et al., 2017). However, the final cell number in the UBP12 and UBP13 overexpression lines was not increased, suggesting that a strong reduction of DA1 activity rather delays development but does not increase the duration of cell proliferation and hence the final number of cells.
Previously, it has been shown that DA1, DAR1 and DAR2 negatively regulate their own activity by cleaving their activating E3-ligases BB and DA2, resulting in an activation-repression system (Dong et al., 2017). UBP12 and UBP13 form an additional layer of post-translational regulation to limit the activity of DA1, DAR1 and DAR2 by specifically removing ubiquitin, and hence further fine-tune organ growth in this growth-regulatory pathway. This dual system to limit and fine-tune the activity of DA1, DAR1 and DAR2 demonstrates the importance of keeping a correct balance between the active and inactive pool of these proteases during leaf development. This is illustrated by the phenotype of plants in which this balance is altered. In wild-type conditions, a correct balance between an inactive and active (ubiquitinated) DA1, DAR1 and DAR2 results in an intact exit from mitosis and standard ploidy levels in leaf cells, which leads to normal plant growth (Figure 7A). High levels of UBP12 or UBP13 can however shift the balance to a depletion of activated DA1, DAR1 and DAR2, which results in a delayed endoreduplication, a severe reduction in cell size and stunted plant growth (Figure 7B), which is highly similar to the phenotype of da1ko_dar1-1_dar2-1 mutants (Peng et al., 2015). Low levels of UBP12 and UBP13 might on the other hand lead to an increase in activated DA1, DAR1 and DAR2 levels, more destabilization of its substrates and can hence limit plant growth by reducing both cell area and cell number (Figure 7C). Higher levels of DA1 and mutations in UBP15 have previously been reported to decrease leaf area and cell number (Du et al., 2014; Liu et al., 2008; Vanhaeren et al., 2017). In ubp12-2 mutants and overexpression lines of UBP12 and UBP13, leaf size and the average area of pavement cells are reduced. Because both very low and high levels of DA1 result in growth reduction (Peng et al., 2015; Vanhaeren et al., 2017), a tight balance between active and inactive DA1, DAR1 and DAR2 is crucial for normal plant development. With UBP12 and UBP13 expression levels being relatively constant throughout leaf development, unknown post-translational modifications of UBP12 and UBP13 are likely to alter the activity or specificity towards DA1, DAR1 and DAR2, as is the case with other deubiquitinating enzymes (Huang and Cochran, 2013). Additionally, it remains also unclear if this reduction in cell area in the ubp12-2 mutants or UBP12 and UBP13 overexpression lines results only from altered levels of DA1 activity, or if UBP12 and UBP13 regulate cell expansion through other growth regulators.
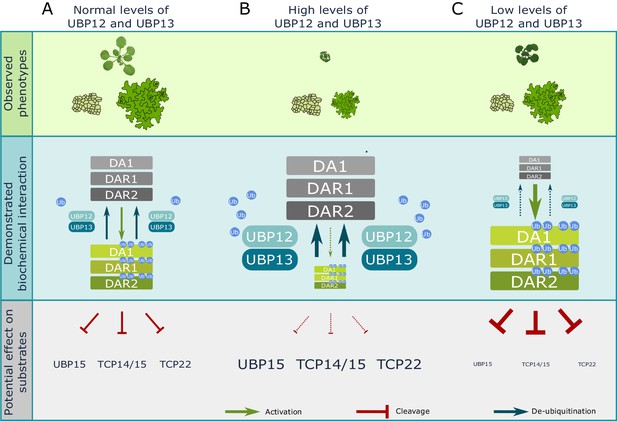
Model of UBP12 and UBP13 levels on leaf area and cellular phenotypes.
Molecular balance and leaf phenotypes in (A) wild-type conditions, (B) high UBP12 and UBP13 expression lines and (C) lower levels of UBP12 and UBP13.
Over the years, it has become increasingly clear that DUBs play a greater role in the development of eukaryotes than just processing and recycling free ubiquitin. Many DUBs are involved in fine-tuning molecular pathways by stabilizing proteins (An et al., 2018; Jeong et al., 2017; Lee et al., 2019; Park et al., 2019), through controlling protein endocytosis (Crespo-Yàñez et al., 2018; Row et al., 2006), by mediating DNA damage repair (Nijman et al., 2005) and by regulating transcription (Derkacheva et al., 2016; Joo et al., 2007; Zhu et al., 2007) to name a few. Potentially, DUBs could remove ubiquitin of existing chains so other chain types can be formed or other PTMs can be added.
Although our knowledge of organ growth has increased substantially over the last years (Figure 7—figure supplement 1), the developmental and environmental triggers that initiate the ubiquitination and deubiquitination of DA1, DAR1 and DAR2, the dominant-negative nature of DA1R358K, additional potential substrates of the ubiquitin-activated peptidases and other post-translational regulatory elements remain some of the many compelling mysteries of this intriguing growth-regulatory pathway.
Materials and methods
Reagent type (species) or resource | Designation | Source or reference | Identifiers | Additional information |
---|---|---|---|---|
Gene (Arabidopsis thaliana) | DA1 | TAIR | AT1G19270 | |
Gene (Arabidopsis thaliana) | DAR1 | TAIR | AT4G36860 | |
Gene (Arabidopsis thaliana) | DAR2 | TAIR | AT2G39830 | |
Gene (Arabidopsis thaliana) | UBP12 | TAIR | AT5G06600 | |
Gene (Arabidopsis thaliana) | UBP13 | TAIR | AT3G11910 | |
Gene (Arabidopsis thaliana) | UBP3 | TAIR | AT4G39910 | |
Gene (Arabidopsis thaliana) | UBP15 | TAIR | AT1G17110 | |
Gene (Arabidopsis thaliana) | UBP24 | TAIR | AT4G30890 | |
Gene (Arabidopsis thaliana) | DA2 | TAIR | AT1G78420 | |
Cell line (Arabidopsis thaliana) | Landsberg erecta | TAIR/ABRC | Germplasm:6530492727 NASC stock number: N84840 | https://www.arabidopsis.org/servlet/TairObject?id=4502009498&type=stock |
Strain, strain background (Escherichia coli) | DH5 α | Thermo-fisher | 18258012 | Chemically competent cells |
Strain, strain background (Escherichia coli) | BL21(DE3) | Thermo-fisher | EC0114 | Chemically competent cells |
Plant material and growth conditions
Request a detailed protocolAll Arabidopsis thaliana mutants and overexpression lines that were used in this study were from the Col-0 background. ubp12-1 (GABI_244E11), ubp12-2 (GABI_742C10), ubp13-1 (SALK_128312), ubp13-2 (SALK_024054) and ubp13-3 (SALK_132368) were kindly provided by Dr. Xia Cui (Chinese Academy of Agricultural Sciences, Beijing, China). The respective T-DNA insertions were verified by PCR. The 35S::GFP-DA1, 35S::GFP-DAR1 and 35S::GFP-DAR2 lines were generated by Gateway cloning using the pK7WGF2 destination vector, the 35S::UBP12 and 35S::UBP13 lines using the pFAST-G02. Because the latter overexpression lines contained the OLE1::GFP construct (Shimada et al., 2010), we could select positive seeds using a fluorescence binocular. The T-DNA lines were grown in soil for 25 days at 21°C and 16-h day/8-hr night cycles for the phenotyping experiments. All overexpression lines and the da1-1 mutant were grown in vitro on plates containing half-strength Murashige and Skoog (MS) medium supplemented with 1% sucrose with a density of one plant per 4 cm2. These plants were grown for 21 days at 21°C and 16-h day/8-hr night cycles. Seedlings for the immunoprecipitation followed by tandem mass spectrometry (IP-MS/MS) were grown for 8 days in liquid half-strength MS medium supplemented with 1% sucrose under shaking conditions (100 rpm) at 21°C and 16-h day/8-hr night cycles. For all experiments, the seeds were stratified in the dark for 2 days at 4°C before being placed in the respective growth rooms. Each quantitative experiment was performed in at least three independent biological repeats, meaning they were sown and harvested at a different time. All genotyping and cloning primers that were used in this study are listed in Supplementary file 1.
Leaf measurements and cellular analysis
Request a detailed protocolLeaves were dissected from the rosette and placed on a square plate containing 1% agar. The plants were imaged and the leaf area was analyzed using ImageJ v1.45 ((RRID:SCR_003070, NIH; http://rsb.info.nih.gov/ij/). For the cellular analysis, samples of leaf 3 (overexpression lines) and leaf 5 (ubp12-2) were cleared in 70% ethanol and mounted in lactic acid on a microscope slide. The total leaf blade area was measured for at least ten representative leaves under a dark-field binocular microscope. For mature leaves, abaxial epidermal cells at the center of the leaf blade, avoiding major veins, were drawn with a microscope equipped with differential interference contrast optics (DM LB with 403 and 633 objectives; Leica) and a drawing tube for at least three leaves. Photographs of leaves and scanned cell drawings were used to measure leaf and individual cell area, respectively, as described by Andriankaja et al. (2012). The statistical analysis of the cellular data was performed in R 3.5.2 (www.r-project.org, RRID:SCR_001905). For the cellular profiling during the transition of cell proliferation to cell expansion, abaxial cells were drawn at the tip of the third leaf at 12 DAS. Scans of cellular drawings were analyzed using ImageJ v1.45 (RRID:SCR_003070, NIH; http://rsb.info.nih.gov/ij/) and cells were colored according to their area in Inkscape (https://inkscape.org/, RRID:SCR_014479).
In vitro deubiquitination and cleaving assays
Request a detailed protocolThe coding sequences of UBP3, UBP12, UBP13, UBP15 and UBP24 were inserted in the pDEST15 (Thermo Fisher, RRID:SCR_008452) destination vector using Gateway cloning to generate GST-UBP3, GST-UBP12, GST- GST-UBP13, GST-UBP15 and GST-UBP24. The coding sequences of DA1, DAR1 and DAR2 were cloned into pDEST-HIS-MBP using Gateway cloning to generate HIS-MBP-DA1, HIS-MBP-DAR1 and HIS-MBP-DAR2. The HIS-DA2 (pET24a) construct was kindly provided by Prof. Michael Bevan (JIC, Norwich, UK). To generate the respective catalytic mutants, we performed site-directed mutagenesis on the entry clones of the respective genes by performing a PCR with primers containing the mutation. After the PCR, 5 µl of Buffer B and 1 µl of DpnI (Promega, RRID:SCR_006724) were added to each reaction. After an overnight incubation, competent DH5α E. coli cells were transformed and the presence of the mutation was checked by sequencing. All expression vectors were transformed into competent BL21 (DE3) E. coli cells. For each protein, the optimal conditions to obtain sufficient soluble proteins were determined (Supplementary file 2). GST-tagged proteins were purified from the bacterial lysate with Glutathione Sepharose 4B beads (GE Healthcare, 17075601) and HIS-tagged proteins with NI-NTA agarose beads (QIAGEN, 30210). Purified proteins were loaded on 4–15% Mini-PROTEAN TGX Precast Protein Gels (Biorad, 4561083DC), stained overnight with Instant Blue (Sigma-Aldrich, ISB1L-1L) and quantified using a BSA standard curve in Image Lab (RRID:SCR_014210, Biorad). The ubiquitination of DA1, DAR1 and DAR2 and the cleaving assays were performed as described before (Dong et al., 2017). After the ubiquitination step, a 1:1 ratio of deubiquitinating enzymes to the ubiquitinated proteins were added and the reaction mix was incubated for 4 hr at 30°C. Reactions were stopped by adding 5x SDS sample buffer and boiled for ten min at 90°C. The samples were loaded on 4–15% or 7.5% Mini-PROTEAN TGX Precast Protein Gels (Biorad, 4561083DC). The proteins in the gels were transferred to a PVDF membrane using Trans-blot turbo transfer packs (Biorad, 170–4156) and the membranes were incubated overnight in a 3% skimmed milk (Difco) 1x TBST solution. After blocking, GST-tagged proteins were detected with Anti-GST HRP Conjugate (Sigma-Aldrich, GERPN1236, RRID:AB_2827942) and MBP-tagged proteins with Anti-MBP Monoclonal Antibody (NEB, E8030S, RRID:AB_1559728) and subsequently with a secondary Rabbit IgG HRP Linked antibody (Sigma-Aldrich Cat# GENA934, RRID:AB_2722659). The antibodies were used following the manufacturer’s instructions.
RNA extraction, cDNA preparation and q-RT-PCR
Request a detailed protocolTotal RNA was extracted from flash-frozen seedlings or isolated leaves with TRIzol reagent (Invitrogen). Young seedlings (until 14 DAS) from which the leaves were isolated using a binocular were submerged overnight in RNA Later (Ambion) to prevent RNA degradation. To eliminate the residual genomic DNA present in the preparation, the RNA was treated by RQ1 RNAse-free DNase according to the manufacturer's instructions (Promega) and purified with the RNeasy Mini kit (Qiagen). Complementary DNA was made with the QScript cDNA supermix kit (Quantabio, 95048–100) according to the manufacturer's instructions. Q-RT-PCR was done on a LightCycler 480 (Roche) in 384-well plates with LightCycler 480 SYBR Green I Master mix (Roche) according to the manufacturer's instructions. Primers were designed with the Primer3 (RRID:SCR_003139, http://frodo.wi.mit.edu/) (Supplementary file 1). Data analysis was performed using the ΔΔCT method (Pfaffl, 2001), taking the primer efficiency into account. The expression data was normalized using three reference genes (AT1G13320, AT2G32170, and AT2G28390) according to the GeNorm algorithm (Vandesompele et al., 2002). The statistical analysis (ANOVA, Dunnett’s test) was performed in GraphPad Prism 8.1 (www.graphpad.com, RRID:SCR_002798).
Flow cytometry
Request a detailed protocolThe first leaf pair was harvested from 9 to 27 DAS with a three-day interval and frozen in liquid nitrogen. At least three leaves per time point of each biological repeat (n = 3) were chopped with a razor blade in 200 µL of Cystain UV Precise P Nuclei Extraction buffer (Sysmex), followed by the addition of 800 µL of Cystain UV Precise P staining buffer (Sysmex) and filtering through a 50-mm filter. Nuclei were analyzed with the Cyflow MB flow cytometer (Partec) and the FloMax software (RRID:SCR_014437). The statistical analysis (ANOVA, Dunnett’s test) was performed in GraphPad Prism 8.1 (www.graphpad.com, RRID:SCR_002798).
IP-MS/MS
Request a detailed protocolThe IP-MS/MS was based on the protocol from Wendrich et al. (2017). For each pull-down, we used 3 g of homogenized Arabidopsis seedlings. The powder was dissolved in 4.5 ml BHB+ buffer supplemented with 4.5 µl benzonase and incubated for 30 min at 4°C. Then, the samples were further mixed three times for 30 s at 18,000 rpm using Ultra-TURRAX miniprobes (IKA). Subsequently, the mixtures were incubated for 30 min at 4°C on an end-over-end rotor. After incubation, the cellular debris was pelleted by two centrifugation steps at 14,000 rpm at 4°C in an Eppendorf centrifuge and further withheld by a 0.45-μm filter (Sartorius). The protein content was measured (OD 595) using a Bradford (Biorad) standard curve and equal amounts of proteins were incubated with 50 μl pre-washed anti-GFP-beads (μMacs, Miltenyi Biotec, RRID:AB_2827943) for 1 hr at 4°C in an end-over-end shaker. To isolate the beads, the columns were placed in the magnetic holder and washed four times with 200 μl BHB+ buffer and two times with 500 μl NH4HCO3 buffer. The purified proteins were eluted stepwise by 50 μl 95°C hot NH4HCO3 each time until no more beads pass through the column. Then, 4 μl Tryp/LysC mix (Promega) was added and the proteins were digested on-bead for 4 hr at 37°C with agitation (800 rpm) on an Eppendorf thermomixer. The digested mix was loaded again on the μMacs column attached to the magnetic holder to separate the eluate from beads. The eluate was collected in a new protein low binding Eppendorf tube and additionally 2 μl Tryp/LysC was added for an overnight digestion at 37°C with agitation (800 rpm) in an Eppendorf thermomixer. Finally, the samples were snap-frozen in liquid nitrogen and freeze-dried in a Speedvac (Labconco). Protein identification and data analysis were performed as described before (Van Leene et al., 2015).
In vitro and in vivo pull-own
Request a detailed protocolThe in vitro pull-down experiments were performed as described before (Dong et al., 2017). As recombinant bait proteins, we used equal quantities of recombinant GST (Prospec, ENZ-393) and full-length GST-UBP12 or GST-UBP13. For the in vivo pull-down, full expression constructs of 35S::RFP-DA1, 35S::RFP-DAR1, 35S::RFP-DAR2, 35S::GFP-UBP12 and 35S::GFP were amplified by PCR and cloned into Golden Gate modules (pGGA000, pGGB000) using the Gibson assembly method (NEB, E5510S). Existing bsaI sites in the Gateway p35S and DAR1 coding sequence were mutated by site-directed mutagenesis using DpnI. Subsequently, these constructs were cloned as interaction pairs on a Golden Gate vector to ensure the co-expression of the constructs in each cell. Arabidopsis cell suspension cultures were transformed as described before (Van Leene et al., 2011). Proteins were extracted and purified as described above and the purified fraction was subjected to Western blot. After blocking, GFP-tagged proteins were detected with anti-GFP (Abcam, ab290, RRID:AB_303395) and RFP-tagged proteins with anti-RFP antibody (Chromotek, RFP antibody [6G6], RRID:AB_2631395) and subsequently with a secondary Rabbit IgG HRP-linked antibody (Sigma-Aldrich, NA934v, RRID:AB_2722659) or secondary Mouse IgG HRP-linked antibody (Sigma-Aldrich, NA931v, RRID:AB_2827944), respectively.
Cell free deubiquitination assay
Request a detailed protocolProteins were freshly extracted from 8-day-old seedlings of Col-0, ubp12-2, 35S::UBP12 an 35S::UBP13 using a 0.5 M sucrose, 1 mM MgCl2, 10 mM EDTA (pH 8.0), 5 mM DTT, 50 mM Tris-MES (pH 8.0) extraction buffer without protease inhibitors (one µl of buffer was added per mg plant material). Subsequently, 400 µg of cell-free extract was added per 200 ng ubiquitinated HIS-MBP-DA1 proteins. For each time point, equal amounts of reaction mix were taken and the reaction was stopped by the addition of SDS sample buffer. The samples were blotted and detected as described above. To quantify the deubiquitination, a rectangle was drawn in ImageJ (RRID:SCR_003070, NIH; http://rsb.info.nih.gov/ij/) spanning the size of fully ubiquitinated HIS-MBP-DA1. Then, the lane intensities were plotted and the pixels within the resulting curves were measured.
Transient expression and confocal microscopy
Request a detailed protocolFor the co-localization experiments, the coding sequences of DA1, DAR1 and DAR2 were cloned in the gateway destination vector pK7WGR2 and those of UBP12 and UBP13 in pK7WGF2 to generate N-terminal RFP- and GFP fusion constructs, respectively. A 3-ml culture of competent LBA 4404 cells containing these constructs or P19 was grown overnight (28°C) in YEB medium with the appropriate antibiotics. The next day, one ml of this culture was inoculated in a 9-ml YEB, 10-mM MES, 20-µM acetosyringone solution with the appropriate antibiotics and incubated overnight at 28°C. Then, the bacteria were washed twice in a 100-mM MGCl2, 10-mM MES, 20-µM acetosyringone buffer and the OD600 was adjusted to one for all cultures and further incubated for two hours at 28°C. Then, equal mixtures of the constructs were infiltrated in 4-week-old Nicotiana benthamiana leaves. After three nights, leaf discs were imaged with a Zeiss LSM 710 confocal inverted microscope (RRID:SCR_018063, Zeiss) to image the RFP- (lasers: 561 nm: 3.0%, beam splitters: MBS: MBS 488/561, pinhole: 100 µm,, digital gain: 1.00, Master gain: 925) and GFP fusion proteins (lasers: 488 nm: 2.0%, beam splitters: MBS_InVis: Plate, pinhole: 100 µm, digital gain: 0.75, Master gain: 956).
Data availability
All generated data is included in the data source files.
References
-
Ubiquitin extension proteins of Arabidopsis thaliana. Structure, localization, and expression of their promoters in transgenic tobaccoThe Journal of Biological Chemistry 265:12486–12493.
-
Structure and evolution of genes encoding polyubiquitin and ubiquitin-like proteins in Arabidopsis thaliana ecotype columbiaGenetics 139:921–939.
-
The ubiquitination machinery of the ubiquitin systemThe Arabidopsis Book 12:e0174.https://doi.org/10.1199/tab.0174
-
Breaking the chains: deubiquitylating enzyme specificity begets functionNature Reviews Molecular Cell Biology 20:338–352.https://doi.org/10.1038/s41580-019-0099-1
-
Cell cycling and cell enlargement in developing leaves of ArabidopsisDevelopmental Biology 215:407–419.https://doi.org/10.1006/dbio.1999.9443
-
Regulation of deubiquitinase proteolytic activityCurrent Opinion in Structural Biology 23:806–811.https://doi.org/10.1016/j.sbi.2013.07.012
-
Deubiquitylating enzymes and their emerging role in plant biologyFrontiers in Plant Science 5:56.https://doi.org/10.3389/fpls.2014.00056
-
Breaking the chains: structure and function of the deubiquitinasesNature Reviews Molecular Cell Biology 10:550–563.https://doi.org/10.1038/nrm2731
-
Control of final seed and organ size by the DA1 gene family in Arabidopsis thalianaGenes & Development 22:1331–1336.https://doi.org/10.1101/gad.463608
-
Perception and signaling of strigolactonesFrontiers in Plant Science 7:1260.https://doi.org/10.3389/fpls.2016.01260
-
A new mathematical model for relative quantification in real-time RT-PCRNucleic Acids Research 29:45e.https://doi.org/10.1093/nar/29.9.e45
-
The ubiquitin isopeptidase UBPY regulates endosomal ubiquitin dynamics and is essential for receptor down-regulationJournal of Biological Chemistry 281:12618–12624.https://doi.org/10.1074/jbc.M512615200
-
The ubiquitin-proteasome system regulates plant hormone signalingThe Plant Journal 61:1029–1040.https://doi.org/10.1111/j.1365-313X.2010.04112.x
-
E3 ubiquitin ligases: ubiquitous actors in plant development and abiotic stress responsesPlant and Cell Physiology 58:1461–1476.https://doi.org/10.1093/pcp/pcx071
-
The leaf index: heteroblasty, natural variation, and the genetic control of polar processes of leaf expansionPlant and Cell Physiology 43:372–378.https://doi.org/10.1093/pcp/pcf051
-
The ubiquitin-26S proteasome system at the nexus of plant biologyNature Reviews Molecular Cell Biology 10:385–397.https://doi.org/10.1038/nrm2688
-
In Vivo Identification of Plant Protein Complexes Using IP-MS/MSMethods in Molecular Biology 1497:147–158.https://doi.org/10.1007/978-1-4939-6469-7_14
-
UBIQUITIN-SPECIFIC PROTEASES function in plant development and stress responsesPlant Molecular Biology 94:565–576.https://doi.org/10.1007/s11103-017-0633-5
Article and author information
Author details
Funding
Bijzonder Onderzoeksfonds (BOF08/01M00408)
- Mattias Vermeersch
Bijzonder Onderzoeksfonds (01SC3117)
- Ying Chen
China Scholarship Council (201604910566)
- Ying Chen
Research Foundation Flanders (12V0218N)
- Hannes Vanhaeren
The funders had no role in study design, data collection and interpretation, or the decision to submit the work for publication.
Acknowledgements
We like to express our gratitude to our colleagues of the Systems Biology of Yield and the Proteomics group, in particular Marieke Dubois and Ting Li, for all constructive discussions and scientific advice. We also like to thank Nancy Helderwert for preparing a tremendous amount of LB+ medium and Annick Bleys for her help in preparing the manuscript. The IP-MS/MS samples were processed by the VIB Proteomics Core (Ghent University, 9000 Ghent, Belgium).
Copyright
© 2020, Vanhaeren et al.
This article is distributed under the terms of the Creative Commons Attribution License, which permits unrestricted use and redistribution provided that the original author and source are credited.
Metrics
-
- 3,208
- views
-
- 770
- downloads
-
- 34
- citations
Views, downloads and citations are aggregated across all versions of this paper published by eLife.
Citations by DOI
-
- 34
- citations for umbrella DOI https://doi.org/10.7554/eLife.52276