Delta glutamate receptor conductance drives excitation of mouse dorsal raphe neurons
Abstract
The dorsal raphe nucleus is the predominant source of central serotonin, where neuronal activity regulates complex emotional behaviors. Action potential firing of serotonin dorsal raphe neurons is driven via α1-adrenergic receptors (α1-AR) activation. Despite this crucial role, the ion channels responsible for α1-AR-mediated depolarization are unknown. Here, we show in mouse brain slices that α1-AR-mediated excitatory synaptic transmission is mediated by the ionotropic glutamate receptor homolog cation channel, delta glutamate receptor 1 (GluD1). GluD1R-channels are constitutively active under basal conditions carrying tonic inward current and synaptic activation of α1-ARs augments tonic GluD1R-channel current. Further, loss of dorsal raphe GluD1R-channels produces an anxiogenic phenotype. Thus, GluD1R-channels are responsible for α1-AR-dependent induction of persistent pacemaker-type firing of dorsal raphe neurons and regulate dorsal raphe-related behavior. Given the widespread distribution of these channels, ion channel function of GluD1R as a regulator of neuronal excitability is proposed to be widespread in the nervous system.
eLife digest
Serotonin is a chemical that allows cells to communicate in the nervous system of many animals. It is also particularly important in the treatment of mental health disorders: a large number of antidepressants work by preventing nerve cells from clearing away serotonin, therefore increasing the overall level of the molecule in the brain. Yet, exactly how serotonin is released remains unclear.
When a serotonin-producing cell is activated, a series of biochemical processes lead to the creation of an electric current that, ultimately, is required for the cell to release serotonin. This mechanism starts when the α1-adrenergic receptor, a protein at the surface of the cell, detects noradrenaline molecules. However, on its own, the α1-adrenergic receptor is unable to create an electric current: this requires ion channels, another type of protein which can let charged particles in and out of the cell. Here, Gantz et al. set out to determine the identity of the ion channel that allows noradrenaline signals to generate electrical activity in cells which can release serotonin.
Electrical and chemical manipulation of mouse brain slices revealed that an ion channel called delta-glutamate 1 was active in serotonin-producing cells exposed to noradrenaline. In fact, applying toxins that specifically blocked the activity of this channel also prevented the cells from responding electrically to noradrenaline.
Further experiments used mice whose serotonin-producing cells were genetically modified to turn off delta-glutamate 1. In turn, these animals showed anxiety-like behaviors, which could be consistent with a drop in serotonin levels. This is in line with previous human studies showing that patients with depression and other mental health conditions have mutations in the gene for delta-glutamate 1.
Taken together, these results give an insight into the electrical activity of serotonin-producing cells. Further work is now required to examine how changes in the gene that codes for delta-glutamate 1 ultimately affect the release of serotonin. This could potentially help to understand if certain individuals may not be able to properly produce this chemical. As many antidepressants work by retaining serotonin that is already present in the brain, this knowledge could ultimately help patients who do not currently respond to treatment.
Introduction
Recent reports estimate that 1 in 5 adults worldwide are affected by a mental health disorder, with anxiety and depression being the most common affecting more than 260 million people (GBD 2017 Disease and Injury Incidence and Prevalence Collaborators, 2018). Most current pharmacotherapies to treat these disorders target serotonin receptors or serotonin clearance. The dorsal raphe nucleus is the largest serotonergic nucleus in the brain and the predominant source of central serotonin (5-HT). In vivo, tonic noradrenergic input to the dorsal raphe that activates Gαq/11 protein-coupled α1-adrenergic receptors (α1-ARs) is required for 5-HT neurons to fire action potentials (Baraban and Aghajanian, 1980; Baraban et al., 1978) and release 5-HT (Clement et al., 1992). In dorsal raphe brain slices, synaptic activation of α1-ARs produces a slow membrane depolarization lasting tens of seconds (Yoshimura et al., 1985). Despite having a crucial role in regulating 5-HT neuron excitability, the ion channels responsible for the depolarization remain unknown.
Throughout the central and peripheral nervous system, activation of Gαq/11 protein-coupled receptors (GqPCRs), namely metabotropic glutamate mGluRs, muscarinic acetylcholine M1 (mAChRs), or α1-ARs produces slow, noisy inward currents. Multiple mechanisms have been reported to underlie the inward current including: inhibition of K+ current (including leak, Ca2+-activated, and Kv7/M-current) (Benson et al., 1988; Halliwell and Adams, 1982; Madison et al., 1987; Shen and North, 1992), modulation of TTX-sensitive persistent Na+ current (Yamada-Hanff and Bean, 2013), and activation of transient potential receptor canonical (TRPC) (Hartmann et al., 2008; Kim et al., 2003), Na+-leak (NALCN) (Lu et al., 2009), or delta glutamate receptor-channels (Ady et al., 2014; Benamer et al., 2018).
The delta glutamate receptors, GluD1R and GluD2R, are mysterious members of the ionotropic glutamate receptor family in that they are not gated by glutamate (Araki et al., 1993; Lomeli et al., 1993). One theory is that they are strictly scaffolding proteins or synaptic organizers, rather than ion conducting channels. But wild-type channels have been reported to conduct in response to activation of mGluRs (Ady et al., 2014; Benamer et al., 2018). GluD1R (Grid1) mRNA is expressed widely throughout the brain, with notably high levels in the dorsal raphe (Hepp et al., 2015; Konno et al., 2014). Here, we used a combination of in vitro patch-clamp electrophysiology and pharmacology with a CRISPR/Cas9 viral genetic strategy to determine that activation of α1-ARs in the dorsal raphe depolarizes neurons via GluD1R-channel conductance. We utilize the α1-AR-GluD1R-EPSC to explore conduction and biophysical properties of GluD1R-channels, to ultimately glean a greater understanding of GluD1R-channel gating. Lastly, we demonstrate that functional deletion of GluD1R-channels in the dorsal raphe produces an anxiogenic behavioral phenotype.
Results
Synaptic activation of α1-adrenergic receptors produces an EPSC
Electrophysiological recordings were made from dorsal raphe neurons in acute brain slices from wild-type mice at 35° C in the presence of NMDAR, AMPAR, KainateR, GABA-AR, and 5-HT1AR antagonists. With cell-attached recordings, a train of 5 electrical stimuli (60 Hz), delivered to the brain slice via a monopolar stimulating electrode, produced firing in previously quiescent neurons, which was blocked by application of the α1-AR antagonist, prazosin (100 nM, Figure 1A). The excitation produced 20±5 action potentials that lasted 9.0±3.0 s, with a latency of 650.6±0.1 ms from onset of stimulation to the first action potential (Figure 1B-E). In whole-cell recording using a potassium-based internal solution, the same train of electrical stimuli produced prolonged action potential firing (Figure 1F). In voltage-clamp mode (Vhold -65 mV), the same stimulation produced a slow and long-lasting (27.4±2.3 s, n=10) excitatory postsynaptic current (EPSC, Figure 1F) that was eliminated by the application of prazosin (Figure 1G). Prazosin had no effect on basal whole-cell current (-3.8±3.4 pA, p=0.232, n=10, data not shown) indicating a lack of persistent inward current due to noradrenaline tone. On average, the duration of the α1-AR-EPSC was orders of magnitude longer than fast AMPAR channel-mediated EPSCs (~103.5×) and ~18× longer than ‘slow’ 5-HT1A receptor-G protein-coupled inwardly rectifying potassium channel (GIRK)-dependent IPSCs (Gantz et al., 2015a; Figure 1H). To test whether α1-AR-EPSCs were dependent on G protein-signaling, recordings were made with an internal solution containing GDPβS-Li3 (1.8 mM) in place of GTP. Disruption of G protein signaling with intracellular dialysis of GDPβS-Li3 eliminated the α1-AR-EPSC within 5-20 mins post-break-in (p=0.004, n=9), whereas dialysis with LiCl alone had no effect on the amplitude of the α1-AR-EPSC (p=0.625, n=4, Figure 1I). These findings demonstrate a cell-autonomous requirement of G protein signaling in the generation of the α1-AR-EPSC. Application of tetrodotoxin (1 μM) reversibly abolished the α1-AR-EPSC, demonstrating a dependence on presynaptic action potentials (Figure 1J). Disruption of the vesicular monoamine transporter with reserpine (1 μM) or removal of external Ca2+ also eliminated the α1-AR-EPSC, indicating noradrenaline release is vesicular (Figure 1K and L).
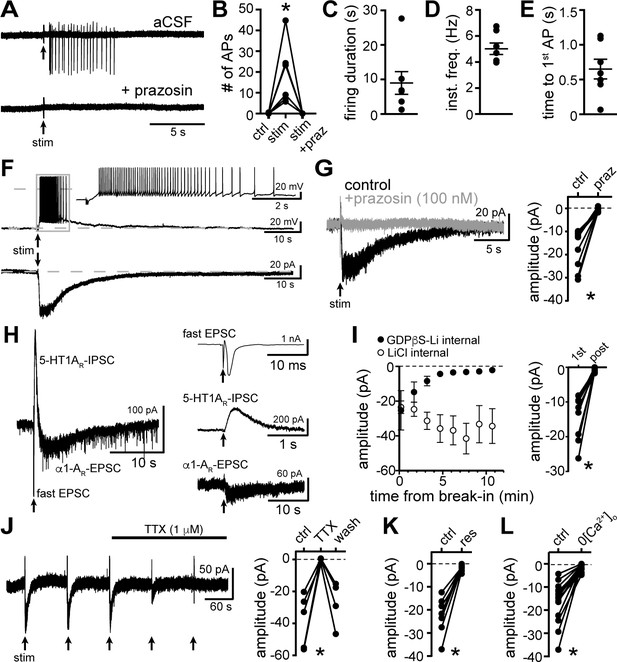
Electrical stimulation evokes long-lasting action potential firing produced by an α1-adrenergic receptor-dependent EPSC.
(A) Representative traces of cell-attached recording where stimulation of the brain slice (5 stims at 60 Hz) produced action potential firing that was abolished by application of the α1-adrenergic receptor antagonist, prazosin (100 nM). (B) Plot of number of action potentials showing the stimulation-induced increase in frequency (p=0.004, n = 6). (C) Plot of duration of action potential firing. (D) Plot of mean instantaneous frequency of action potential firing over the first 10 s of firing. (E) Plot of the latency from stimulation onset to the first action potential. (F) Example whole-cell recordings in the same cell, where electrical stimulation of the slice produced prolonged action potential firing in current-clamp (upper trace) and a slow EPSC in voltage-clamp mode (lower trace). (G) Bath application of prazosin eliminated the slow EPSC shown in a representative trace (left, baseline adjusted) and in grouped data (right, p=0.002, n = 10). (H) Representative traces of a whole-cell recording when the brain slice was stimulated in the absence of antagonists showing the kinetics of the α1-AR-EPSC relative to the fast EPSC (peak has been truncated) and 5HT1AR-IPSC (left). Subsequent addition of AMPAR/KainateR and GABA-AR and 5-HT1AR antagonists revealed the remaining synaptic current produced by α1-AR activation (right). Time of stimulations are marked by arrows. (I) With GDPβS-Li3-containing internal solution, the amplitude of the α1-AR-EPSC ran down within ~5–20 min of break-in to whole-cell mode; shown in a plot compared with control internal solution containing LiCl only (left) and in grouped data (right, p=0.004, n = 9, 1st: first EPSC; post: post-dialysis). (J) Bath application of tetrodotoxin (TTX, 1 μM) reversibly eliminated the α1-AR-EPSC shown in a representative trace (left, α1-AR-EPSC evoked every 90 s (arrows)) and in grouped data (right, p=0.009, n = 4). (K) Plot of the inhibition of α1-AR-EPSC amplitude by application of reserpine (res, 1 μM, p=0.016, n = 7). (L) Plot of the inhibition of α1-AR-EPSC amplitude by removal of external Ca2+ (0[Ca2+]o, p=0.0001, n = 14). Line and error bars represent mean ± SEM, * denotes statistical significance.
-
Figure 1—source data 1
Numerical data that were used to generate graphs in Figure 1.
- https://cdn.elifesciences.org/articles/56054/elife-56054-fig1-data1-v2.xlsx
Biophysical properties of the channel
Under our recording conditions, resistance of the membrane (Rm) significantly decreased during the α1-AR-EPSC, indicative of opening of ion channels (Figure 2A). Membrane noise variance (σ2) increased significantly during the EPSC compared to membrane noise under basal conditions (Figure 2B and C). The α1-AR-EPSC σ2 – amplitude relationship was well fit by linear regression, suggestive of a consistent conductance state, yielding an estimate of a -1.16 pA unitary current (Figure 2D). Voltage ramps from -120 to -10 mV (1 mV/10 ms) before and during the α1-AR-EPSC (Figure 2E) showed that the current reversed polarity at -28.6±2.4 mV (Figure 2E-G). Exogenous application of noradrenaline (30 μM, in the presence of α2-AR antagonist, idazoxan, 1 μM) produced an inward current (INA) with a similar reversal potential (-25.1±2.9 mV, Figure 2G). Replacing extracellular Na+ (126 mM) with N-methyl D-glucamine (NMDG) completely abolished inward INA, suggesting Na+ as the prominent charge carrier (Figure 2H). Increasing extracellular K+ from 2.5 to 6.5 or 10.5 mM, expected to shift Ek from -107 to -81 and -69 mV, respectively, had no effect on the amplitude of the α1-AR-EPSC at Vhold -65 mV (Figure 2I) nor -120 mV (p=0.692, n=11, data not shown), but produced a significant depolarizing shift in Erev of the α1-AR-EPSC (Figure 2J), suggesting the channel is also permeable to K+, and may be 2-3× as permeable to K+ as Na+. Removal of external MgCl2 had no significant effect on Erev (-28.5±5.7 mV), nor on the amplitude of INA (Figure 2K and L). Removal of external CaCl2 also had no effect on Erev (-30.3±3.5 mV) but significantly augmented inward INA, (Figure 2K and L). Taken together, the data suggest that α1-AR-dependent current, whether produced by vesicular release of noradrenaline or exogenous noradrenaline application is carried through a mixed cation channel, with inward current carried predominantly by Na+ entry. Here, measurements of Erev assume voltage-independence of the channel and the signaling mechanism by which α1-AR signal to the channel. To test for voltage-dependence, we employed a two-pulse voltage-step protocol. Current was measured at Vhold -120 mV following a conditioning pre-pulse (-120 to 30 mV, 150 ms) before and after application of noradrenaline (Figure 2—figure supplement 1A and B). INA was isolated by subtracting the current under basal conditions from the current during noradrenaline. Conductance (GNA) was calculated, using an Erev of -25.1 mV. Conditioning depolarizing pre-pulses incrementally reduced GNA and the increase in membrane noise induced by noradrenaline measured at Vhold -120 mV (Figure 2—figure supplement 1C and D), demonstrating voltage-dependence of inward INA, such that depolarization reduced conductance.
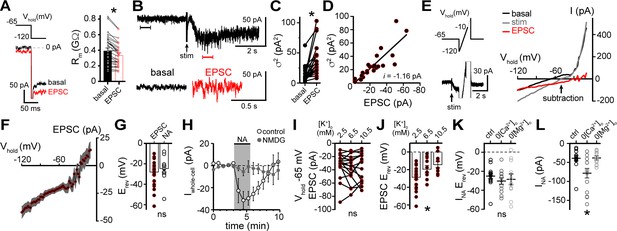
α1-adrenergic receptor-dependent inward current is carried by sodium entry.
(A) Membrane resistance (Rm, ΔV −65 to −120 mV) decreased during the α1-AR-EPSC indicating an opening of ion channels, as shown in an example trace (left) and in grouped data (right, p<0.0001, n = 31). (B) Representative trace of membrane noise during the α1-AR-EPSC, brackets denote segments shown below on an expanded scale. (C) Membrane noise (variance, σ2) increased during the α1-AR-EPSC (p<0.0001, n = 22). (D) Plot of α1-AR-EPSC variance versus mean amplitude, linear fit represents mean unitary current (i, r2 = 0.713, p<0.0001). (E) Slow voltage ramps (1 mV/10 ms, analyzed from −120 to −10 mV) were used to determine the current-voltage relationship of the α1-AR-EPSC (subtraction), determined by subtracting current at the peak of the α1-AR-EPSC (stim) from current measured in control conditions just prior to stimulation (basal). Current generated during ramps were truncated for clarity. (F) Current-voltage relationship of the α1-AR-EPSC from grouped data. Shaded area represents mean ± SEM. (G) Plot of reversal potentials (Erev) of the α1-AR-EPSC and INA (p>0.999, n = 26 and 14). (H) Replacing 126 mM NaCl with NMDG eliminated inward INA, shown in a time-course plot (Vhold−65 mV, p<0.0001, n = 14 and 13). (I) Plot of α1-AR-EPSC amplitudes measured at Vhold−65 mV, in 2.5, 6.5, and 10.5 mM [K+]o (p=0.162, n = 17). (J) Plot of α1-AR-EPSC reversal potential (Erev) with varying concentration of external K+ ([K+]o), demonstrating a depolarizing shift in Erev as external K+ was increased (p=0.010, n = 26, 10, and 11). (K) Plot of reversal potentials (Erev) of INA, demonstrating no significant difference between control conditions (ctrl), and after removal of external Ca2+ (0[Ca2+]o, p=0.49, n = 14 and 12) or Mg2+ (0[Mg2+]o, p=0.73, n = 14 and 11). (L) Plot of the amplitude of INA (Vhold−65 mV) demonstrating an augmented INA amplitude in 0[Ca2+]o (p=0.017, n = 14), but not in 0[Mg2+]o, (p>0.9999, n = 11) as compared with control conditions (n = 14). Line and error bars represent mean ± SEM, * denotes statistical significance, ns denotes not significant.
-
Figure 2—source data 1
Numerical data that were used to generate graphs in Figure 2.
- https://cdn.elifesciences.org/articles/56054/elife-56054-fig2-data1-v2.xlsx
α1-adrenergic receptors modulate tonic GluD1R-channel current
To assess involvement of GluD1R-channels in carrying the α1-AR-EPSC, we applied 1-Naphthyl acetyl spermine (NASPM), a synthetic analogue of Joro spider toxin that is an open-channel blocker of some other Ca2+-permeable ionotropic glutamate receptors (Blaschke et al., 1993; Guzmán et al., 2017; Koike et al., 1997) and of GluDR-channels (Benamer et al., 2018; Kohda et al., 2000). Application of NASPM (100 μM, 6 min) blocked the α1-AR-EPSC (96.0 ± 12.5% reduction), which recovered to baseline after a wash of >30 mins (Figures 3A, B, E and I). NASPM also produced an apparent outward current (INSP) of 20.5 ± 3.7 pA with an Erev of −31.4 ± 4.8 mV (Figures 3A, C, E and G) and a reduction in membrane noise (Figure 3A and D). After washout, INSP reversed with a similar time course of recovery of the α1-AR-EPSC (Figure 3E). INSP was associated with an increase in Rm (Figure 3F) indicating a closure of channels. Replacing extracellular Na+ (126 mM) with NMDG eliminated INSP (Figure 3G). Thus, INSP was due to block of tonic Na+-dependent inward current. INSP was not dependent on prior electrical stimulation of the brain slice, as the magnitude of INSP was similar between stimulated and unstimulated brain slices (Figure 3H). Given that NASPM is an open-channel blocker (Koike et al., 1997), we tested whether electrically evoking an α1-AR-EPSC during the application of NASPM was required for block. After obtaining a steady α1-AR-EPSC baseline, NASPM was applied for 6 min without stimulating the brain slice. The α1-AR-EPSC was blocked when stimulation was reapplied (Figure 3I), indicating that the channels underlying the α1-AR-EPSC were already blocked. Thus, the α1-AR-EPSC is mediated by channels that are at least transiently open at rest and may be the same channels underlie the apparent outward current induced by NASPM.
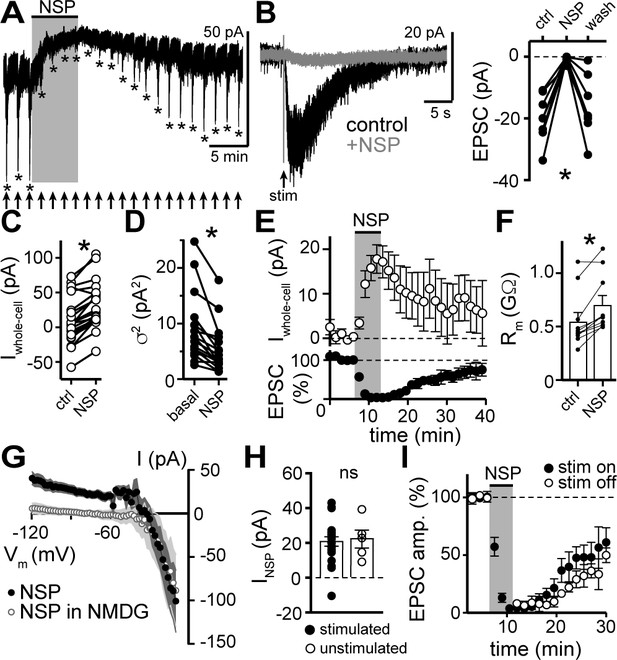
NASPM blocks the α1-AR-EPSC and a tonic sodium inward current.
(A) Example whole-cell voltage-clamp recording of the basal whole-cell current and the α1-AR-EPSC evoked every 90 s prior to, during, and after bath application of NASPM (NSP, 100 μM). Time of stimulations are marked by arrows and peak of the α1-AR-EPSC are marked by asterisks. (B) NASPM completely eliminated the α1-AR-EPSC shown in representative traces (left, baseline adjusted) and in grouped data (right, p=0.001, n = 8). (C) Bath application of NASPM produced an apparent outward current (p<0.0001, n = 21). (D) Membrane noise (variance, σ2) decreased following NASPM (NSP, p<0.0001, n = 19).(E) Time course of the inhibition of the α1-AR-EPSC amplitude (bottom) and apparent outward current (top) by application of NASPM. (F) Membrane resistance (Rm, ΔV −65 to −75 mV) increased during bath application of NASPM, indicating the apparent outward current was due to ion channels closing (p=0.004, n = 10). (G) Current-voltage relationship of apparent outward current produced by NASPM (n = 8). Replacing 126 mM NaCl eliminated the apparent outward current (n = 11), suggesting a block of a tonic inward Na+ current. Shaded area represents mean ± SEM. (H) Plot of amplitude of NASPM-induced apparent outward current in stimulated and unstimulated brain slices demonstrating no effect of prior electrical stimulation (p=0.850, n = 21 and 5). (I) Time course of the inhibition of the α1-AR-EPSC amplitude by application of NASPM, demonstrating identical block of the α1-AR-EPSC whether or not α1-AR-EPSCs were evoked during NASPM application. Line and error bars represent mean ± SEM, ns indicates not significant, * denotes statistical significance.
-
Figure 3—source data 1
Numerical data that were used to generate graphs in Figure 3.
- https://cdn.elifesciences.org/articles/56054/elife-56054-fig3-data1-v2.xlsx
GluDRs bind the amino acids D-serine and glycine, both of which partially reduce constitutively open mutant and wild-type GluDR channel current (Ady et al., 2014; Benamer et al., 2018; Naur et al., 2007; Yadav et al., 2011), likely by inducing a conformational change in the channel that resembles a desensitized state (Hansen et al., 2009). Application of D-serine (10 mM, 13.5 min) reduced the amplitude of the α1-AR-EPSC by 49.7 ± 9.6% (Figure 4A and E), without affecting unitary channel current (Figure 4B). Application of glycine (10 mM, 4.5 mins, in the presence of the glycine receptor antagonist, strychnine (10 μM), also reduced the amplitude of the α1-AR-EPSC by 70.9 ± 11.0% (Figure 4C and E), without affecting unitary channel current (Figure 4D). Lastly, we found that application of the glutamate receptor antagonist kynurenic acid (1 mM, 10.5 min) reduced the α1-AR-EPSC amplitude by 65.6 ± 8.3% (Figure 4E).
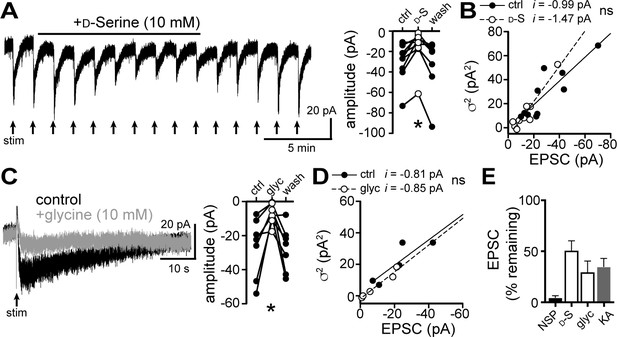
D-serine and glycine reduce the α1-AR-EPSC.
(A) Bath application of D-serine (10 mM) reversibly reduced the α1-AR-EPSC, shown in a representative trace (left) and in grouped data (right, p=0.001, n = 7). (B) Plot of α1-AR-EPSC variance versus mean amplitude prior to (ctrl) and after reduction by D-serine (D–S), linear fit represents mean unitary current (i), demonstrating no change in i with D-serine (p=0.165, n = 10 and 10). (C) Bath application of glycine (10 mM) reversibly reduced the α1-AR-EPSC, shown in representative traces (left, baseline adjusted) and in grouped data (right, p=0.015, n = 7). (D) Plot of α1-AR-EPSC variance versus mean amplitude prior to (ctrl) and after reduction by glycine (glyc), linear fit represents mean unitary current (i), demonstrating no change in i with glycine (p=0.895, n = 5 and 5). (E) Summarized data of percent remaining in α1-AR-EPSC after NASPM (NSP, 100 μM), D-serine (D-S, 10 mM), glycine (glyc, 10 mM), or kynurenic acid (KA, 1 mM). Line and error bars represent mean ± SEM, ns indicates not significant, * denotes statistical significance.
-
Figure 4—source data 1
Numerical data that were used to generate graphs in Figure 4.
- https://cdn.elifesciences.org/articles/56054/elife-56054-fig4-data1-v2.xlsx
Next, a viral genetic strategy was used to functionally delete GluD1R-channels by targeting the encoding gene, Grid1, via CRISPR/Cas9 (Figure 5—figure supplement 1A–C). In brief, one of two cocktails of AAV1 viruses were microinjected into the dorsal raphe of wild-type mice. The Grid1 cocktail that targeted GluD1R-channels included AAV1 viruses encoding Cas9, and mouse Grid1 guide RNA with a nuclear envelope-embedded enhanced green fluorescent protein (eGFP) reporter. A separate cohort received a control cocktail of AAV1 viruses encoding Cas9 and eGFP reporter (control). Brain slices were prepared after >4 weeks and the dorsal raphe was microdissected and frozen on dry ice to assess the mutation of Grid1. Restriction enzyme site-digested PCR confirmed in vivo mutation of Grid1 at the expected site (Figure 5—figure supplement 1D). In separate Grid1 and control cohorts, brain slices were prepared and whole-cell voltage-clamp recordings were made from transduced and non-transduced neurons visualized in brain slices by expression of eGFP. In eGFP+ neurons from control mice, electrical stimulation produced a decrease in Rm and an α1-AR-EPSC, and bath application of noradrenaline caused inward INA (Figure 5). However, in eGFP+ neurons from Grid1 mice, electrical stimulation did not change Rm (Figure 5A) and no α1-AR-EPSC was detected above baseline noise (Figure 5B and C). In addition, inward INA was substantially smaller in eGFP+ neurons from Grid1 mice, as compared to eGFP+ neurons from control mice (Figure 5D). In the same slices from Grid1 mice, eGFP- neurons still had an α1-AR-EPSC and inward INA (Figure 5B and D). Lastly, bath application of NASPM produced an apparent outward current in eGFP+ neurons from control mice, but not from Grid1 mice (Figure 5E). Taken together, these results demonstrate that conduction through GluD1R-channels is necessary for the α1-AR-EPSC and the NASPM-sensitive tonic inward current.
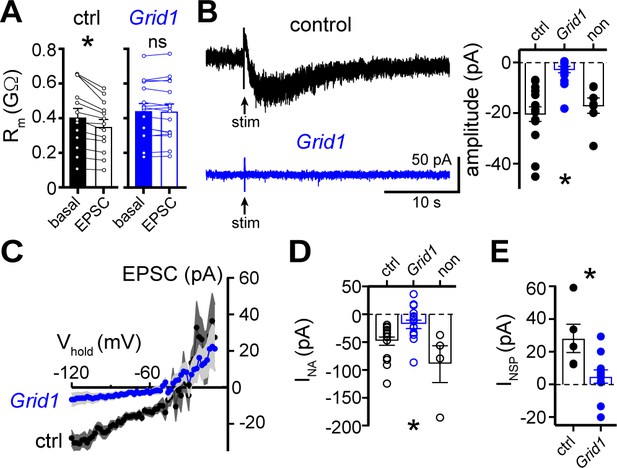
The α1-AR-EPSC is eliminated by targeting of GluD1R-channels via CRISPR/Cas9.
(A) Membrane resistance (Rm, ΔV −65 to −120 mV) decreased after stimulation in transduced neurons from mice injected with AAV-Cas9 and AAV-empty (ctrl, p=0.0002, n = 13), but not in transduced neurons from mice injected with AAV-Cas9 and AAV-Grid1 (Grid1, p=0.562, n = 16). (B) Representative traces (left) and grouped data (right, p<0.0001, n = 15 and 16 and 7) demonstrating the presence of an α1-AR-EPSC in transduced neurons from control mice, but not from Grid1 mice. Neighboring non-transduced neurons from mice injected with AAV-Cas9 and AAV-Grid1 (non) had an α1-AR-EPSC that was indistinguishable from transduced neurons from control mice (p>0.999). (C) Current-voltage relationship of the α1-AR-EPSC from control (n = 13) and Grid1 (n = 16) grouped data. Shaded area represents mean ± SEM. (D) Targeting GluD1R reduced the inward current to noradrenaline (NA, INA,30 μM) as compared to transduced neurons from control mice and neighboring non-transduced neurons (p=0.004, n = 16 and 16 and 4). Inward INA in non-transduced neurons from mice injected with AAV-Cas9 and AAV-Grid1 was similar to transduced neurons from control mice (p=0.631). (E) Targeting GluD1R reduced the tonic inward current revealed by bath application of NASPM (100 μM, INSP) as compared to transduced neurons from control mice (p=0.009, n = 5 and 11). Line and error bars represent mean ± SEM, * denotes statistical significance, ns denotes not significant.
-
Figure 5—source data 1
Numerical data that were used to generate graphs in Figure 5.
- https://cdn.elifesciences.org/articles/56054/elife-56054-fig5-data1-v2.xlsx
Functional deletion of GluD1R-channels in the dorsal raphe produces a behavioral phenotype
To assay a functional role of GluD1R-channels in dorsal raphe-related behavior, wild-type mice received a microinjection into the dorsal raphe of either Grid1 or control virus cocktails. Behavioral assays were conducted >4 weeks post-injection, then the accuracy of the dorsal raphe injection and limited-spread of transduction was verified post-hoc by immunohistochemistry (Figure 6A). Basal locomotion was assayed in a dark arena. There was no difference between the two groups in the total distance traveled (Figure 6B) nor in the velocity of movements between control and Grid1 mice (p=0.772, n = 18 and 16, data not shown). Next, mice were tested on an elevated plus maze in a well-lit room, an experimental assay of rodent anxiety behavior (Walf and Frye, 2007) known to involve both serotonergic and non-serotonergic neurons in the dorsal raphe (Lawther et al., 2015). Grid1 mice spent less time in the open arms when compared to control mice (Figure 6C–E). Control and Grid1 mice made a similar total number of entries to either open or enclosed arms (control: 39.4 ± 2.0; Grid1: 36.0 ± 2.3, p=0.697), but Grid1 mice made proportionally fewer entries to the open arms (Figure 6D). Time spent grooming or in stretched-attend postures were similar between control and Grid1 mice (Figure 6F and G). Since movement in the elevated plus maze reflects conflict between innate drive to explore of a novel environment and natural avoidance of open spaces (Walf and Frye, 2007), we also examined exploratory behaviors. Grid1 mice spent less time lowering their head over the edge of the open arms than control mice (head-dipping, Figure 6H), suggestive of decreased exploratory behavior. However, Grid1 mice spent a similar amount of time rearing in the enclosed arms compared to control mice (Figure 6I) suggesting innate exploratory drive in the enclosed arms was intact. Taken together, these results are indicative of heightened anxiety after functional deletion of GluD1R-channels in the dorsal raphe.

Loss of functional GluD1 receptors in the dorsal raphe produces an anxiogenic behavioral phenotype in mice.
(A) Example maximum intensity projection confocal image of spread of viral transduction following dorsal raphe microinjection of AAV-Cas9 and AAV-Grid1 using an eGFP reporter; scale bars, 0.5 mm. Image was registered and aligned with plate 69 (Franklin and Paxinos mouse brain atlas) with dorsal raphe outlined in solid white. (B) Plot of distance traveled in 30 mins in a dark arena, demonstrating no difference in horizontal locomotion between mice with dorsal raphe microinjections with AAV-Cas9 and AAV-empty (ctrl) versus with AAV-Cas9 and AAV-Grid1 (p=0.762, n = 18 and 15). (C) In an elevated plus maze, Grid1 mice spent less time in the open arms as compared with control-transduced mice (p=0.007, n = 10 and 10). (D) Grid1 mice made proportionally fewer entries to the open arms compared to control-transduced mice (p=0.033, n = 10 and 10). (E) Plot of cumulative time spent in the open arms of an elevated plus maze (EPM). (F) Plot of time spent grooming, demonstrating no different between control-transduced and Grid1 mice (p=0.481, n = 10 and 10). (G) Plot of time spent in stretched-attend posture, demonstrating no difference between control-transduced and Grid1 mice (p=0.968, n = 10 and 9). (H) Grid1 mice spent less time looking over the edge of the open arms (head-dip) than control-transduced mice (p=0.018, n = 10 and 10). (I) Plot of time spent rearing in the enclosed arms, demonstrating no difference between control-transduced and Grid1 mice (p=0.143, n = 10 and 10). Line and error bars represent mean ± SEM, n = number of mice, * denotes statistical significance, ns denotes not significant.
-
Figure 6—source data 1
Numerical data that were used to generate graphs in Figure 6.
- https://cdn.elifesciences.org/articles/56054/elife-56054-fig6-data1-v2.xlsx
Discussion
Physiological relevance of GluD1R-channels to dorsal raphe function
In vivo, 5-HT neurons in the dorsal raphe require noradrenaline release and subsequent activation of α1-ARs to maintain persistent action potential firing (Baraban and Aghajanian, 1980). The activation of α1-ARs in the dorsal raphe by exogenous agonist was thought to depolarize neurons through net reduction of K+ conductance, transiently activating calcium-activated K+ current while persistently decreasing another K+ current, and by activation of an unidentified non-K+ conductance (Pan et al., 1994). In a more recent study in the dorsal raphe, Brown et al. (2002) reported that activation of α1-ARs, induces Na+-dependent inward current with an Erev of −23 mV, similar to our findings. Our study identifies GluD1 receptor-channels as the ion channel that carries this mixed cation current, indicating that modulation of GluD1R-channels is a key constituent in driving persistent action potential firing of the 5-HT neurons. In principle, inward GluD1R-channel current may bring the membrane potential to threshold, but recruitment of other voltage-gated ion channels is expected to underlie the persistent pacemaker-like activity. Intriguingly, Brown et al. (2002) demonstrated that activation of Gq protein-coupled histamine H1 and orexin OX2 receptors also produced an inward current that was occluded by the α1-AR-dependent current. Whether these receptors, and other GqPCRs, augment GluD1R-channel current remains to be determined.
Dysregulation of the 5-HT signaling neuropsychiatric disorders is well-established. Pharmacotherapies to boost serotonin signaling are common and often efficacious in some of these conditions. Genetic association studies have identified GRID1 as a susceptibility gene for psychiatric conditions, including schizophrenia, major depressive disorder, bipolar disorder, autism spectrum disorder, and alcohol dependence (Edwards et al., 2012; Fallin et al., 2005; Griswold et al., 2012). Global Grid1 knock-out mice display abnormal social behaviors, including heightened aggression and decreased social interaction, as well as altered emotional behaviors (Yadav et al., 2012) that are analogous to features of neuropsychiatric conditions in humans. Our study found that functional deletion of GluD1R-channels, specifically in the dorsal raphe, produces a heightened anxiety-like response in the elevated plus maze without changing basal locomotion and exploratory behaviors in non-threatening environments. Previous studies have demonstrated that both 5-HT and non-5-HT/GABAergic dorsal raphe neurons are activated by aversive, anxiety or fear-producing stimuli (Seo et al., 2019; Silveira et al., 1993), with regional subpopulation specificity (Grahn et al., 1999; Grahn et al., 2019; Lawther et al., 2015). Our viral strategy functionally deleted GluD1R-channels in a non-specific manner, targeting all dorsal raphe neurons, including 5-HT and GABAergic neurons. Given the rich diversity of dorsal raphe neuron subtypes and subdivisions within the 5-HT neurons (Huang et al., 2019; Luo et al., 2015; Ren et al., 2018), future work will be needed to parse the behavioral role of GluD1R-channels with subnuclei/subpopulation specificity.
Metabotropic-ionotropic receptor crosstalk modulates ion channel function of GluD1R
GluDR have been characterized as scaffold proteins or synaptic organizers, regulating LTD, endocytosis and trafficking of AMPAR, formation of excitatory and inhibitory synapses, and spine density, independent of ion conduction through the pore (Fossati et al., 2019; Hirai et al., 2003; Schmid and Hollmann, 2008; Tao et al., 2018). Similarly, NMDAR are known to signal through non-ionotropic or ‘metabotropic’ mechanisms where ion conduction is not required, to regulate LTD, AMPAR endocytosis, and spine morphology (Dore et al., 2016). The ability of GluDR-channels to carry ionic current does not conflict with its known role as a synaptic organizer, but rather expands the similarities between NMDAR and GluDR.
The largest obstacle in advancing the understanding of the ionotropic nature of GluDR is the lack of known agonist and inability to gate the intact channel. The majority of studies have been performed on constitutively open mutant or chimeric channels. In domain-swapped chimeric channels, agonist binding to the ligand-binding domain (LBD) of AMPAR or KainateR opens the GluDR-channel pore and generates a substantial current, but the LBD of GluDR on the pore region of AMPAR or KainateR-channels fails to generate current (Orth et al., 2013; Schmid et al., 2009). Two prior studies have demonstrated that in heterologous systems and brain slices, activation of metabotropic glutamate receptors (mGluR) produces an inward current carried by GluD1R- (Benamer et al., 2018) or GluD2R-channels (Ady et al., 2014), concluding that mGluR activation triggers gating of GluDR channels. The congruous explanation of our results is that, in dorsal raphe neurons, GluD1R-channels are functional and open under basal conditions, carrying subthreshold, tonic Na+ current. Activation of α1-ARs, by exogenous agonist or synaptic release of noradrenaline modulates gating of GluD1R-channels and excites dorsal raphe neurons by increasing tonic GluD1R-channel inward current.
In general, the kinetics of iGluR synaptic currents are controlled by the lifetime of the receptor-agonist complex and the rate of desensitization and deactivation. The presence of ambient levels of glutamate and glycine along with slow desensitization activate NMDAR to produce a tonic inward current (Sah et al., 1989). Our results demonstrate that GluD1R are functional ion channels, but whether they function as ligand-gated receptor-channels that open in response to a chemical signal, is not yet determined. What remains to be understood are the conditions that permit GluD1R-channel opening and why their activation has been largely elusive in heterologous expression systems. Reminiscent of times before the discovery of glycine as a necessary co-agonist at NMDAR (Johnson and Ascher, 1987; Kleckner and Dingledine, 1988), it may be that an endogenous agonist needed for gating is present in brain slices. Alternatively, it is possible GluD1R-channels are gated by an intracellular factor or require expression of accessory or interacting protein (Tomita, 2010). Tonic activation of α1-ARs cannot explain the tonic inward current as α1-AR antagonism did not change basal whole-cell current.
The mechanism by which α1-ARs increase GluD1R-channel current also remains to be described and may be distinct from the tonic activation. It is well-established that GqPCRs, especially mGluR and mAChR, bidirectionally change NMDAR and AMPAR ionic currents, producing the two major forms of synaptic plasticity, long-term potentiation (LTP) and long-term depression (LTD), in part through a variety of distinct postsynaptic mechanisms (Hunt and Castillo, 2012). To our knowledge, the duration of the α1-AR-EPSC (~27 s) is exceptional for any known synaptic current and more closely resembles the duration of short-term synaptic plasticity; for instance, endocannabinoid-mediated short-term depression (Lu and Mackie, 2016). Canonically, GqPCRs activate phospholipase C which hydrolyzes the integral membrane lipid phosphatidylinositol 4,5-bisphosphate (PIP2) into inositol triphosphate (IP3) and diacylglycerol. PIP2 stabilizes Kv7 channels such that PIP2 hydrolysis following mAChR activation accounts for inhibition of M-current (Suh and Hille, 2002). In contrast, PIP2 inhibits TRPV4 channels, such that GqPCR-dependent PIP2 depletion allows for TRPV4 channels to open (Harraz et al., 2018). By the same signaling cascade, GqPCRs stimulate the production of the endocannabinoid, 2-AG, that can act directly on ion channels in the membrane (Gantz and Bean, 2017). Thus, one possibility is that α1-ARs modulate GluD1R-channels through membrane lipid signaling, involving PIP2, diacylglycerol, or 2-AG, as it can take tens-of-seconds to minutes for ion channels to recover from modulation by membrane lipids (Gantz and Bean, 2017; Suh and Hille, 2002). Alternatively, there may be direct modulation of GluD1R-channels by G protein subunits or activation of protein kinase signaling cascades. The inclusion of the calcium-chelator BAPTA in the internal recording solution makes it unlikely that α1-ARs modulate GluD1R-channels via IP3 and calcium release from intracellular stores (Hoesch et al., 2004). Largely, it remains to be seen whether these intracellular signaling cascades, many of which are known to affect NMDAR- and AMPAR-channels, modify GluDR-channels.
In heterologous systems and constitutively open mutant GluDR-channels, the current reverses polarity around 0 mV (Zuo et al., 1997), akin to AMPAR- and NMDAR-channels, while our results show Erev of ~ −30 mV. While slow voltage-ramps were employed to minimize space-clamp error, we cannot rule out that some of the difference may be attributed to space-clamp error in brain slices, especially since the magnitude of subtracted current is small relative to total membrane current at depolarized potentials. However, there are many reports of inward currents produced by activation of many different GqPCRs with reversal potentials between −40 and −23 mV (Awad et al., 2000; Brown et al., 2002; Yamada-Hanff and Bean, 2013) under different recording conditions; a commonality that is unlikely to be accounted for by space-clamp error alone. Tail current analysis revealed voltage-dependence of INA, such that depolarization reduced conductance. These data may reflect block of GluD1R-channels by endogenous intracellular polyamines, as established for calcium-permeable AMPAR- and KainateR-channels (Bowie and Mayer, 1995). Another important consideration is that our measurements may be subject to voltage-dependence of the signaling pathway between α1-ARs and GluD1R-channels. Taken together, measurements here should be considered an estimate of GluD1R-channels, and more precisely as the current-voltage relationship of the α1-ARs-GluD1R-channel signaling complex.
Summary
In summary, the α1-AR-mediated depolarization of dorsal raphe neurons that drives action potential firing in vivo is carried by the mixed cation channel, GluD1R. Thus in addition to their role as a scaffold protein, GluD1R are functional ion channels that critically regulate neuronal excitability. Many of the biophysical properties of the GluD1R-channel are like other members of the ionotropic glutamate receptor family. Given the widespread distribution of these receptors throughout the brain (Hepp et al., 2015), ion channel function of GluD1R may be prevalent and relevant to neuronal excitability and circuit function in different parts of the throughout the nervous system. This study lays the foundation to investigate the ion channel function of GluD1R in excitatory GqPCR-dependent synaptic transmission and regulation of neuronal excitability, expanding upon the wealth of knowledge of pharmacology and regulatory elements established for NMDAR and AMPAR signaling.
Materials and methods
Reagent type (species) or resource | Designation | Source or reference | Identifiers | Additional information |
---|---|---|---|---|
Strain, strain background (Mus musculus) | C57BL/6J | The Jackson Laboratory | Stock# 000664 RRID:IMSR_JAX:000664 | males and females |
Strain, strain background (E. coli) | NEB Stable | New England Biolabs | Cat# C3040H | - |
Genetic reagent (adeno-associated virus) | AAV-Cas9 | PMIDs:25326897 30792150 | NIDA IRP Core Facility, AAV1, pX551, RRID:Addgene_60957 | Lot# AAV692 |
Genetic reagent (adeno-associated virus) | AAV-Grid1 | This paper | NIDA IRP Core Facility, AAV1, pOTTC1706, Addgene 131683 | Lot# AAV732 |
Genetic reagent (adeno-associated virus) | AAV-empty | This paper | NIDA IRP Core Facility, AAV1, pOTTC1553, Addgene 131682 | Lot# AAV746 |
Chemical compound, drug | Bgl I restriction enzyme | New England Biolabs | Cat# R0143S | - |
Chemical compound, drug | D-serine | Millipore Sigma | Cat# S4250 | 10 mM |
Chemical compound, drug | GDPβS-Li3 | Millipore Sigma | Cat# G7637 | 1.8 mM |
Chemical compound, drug | Glycine | Millipore Sigma | Cat# G7126 | 10 mM |
Chemical compound, drug | Idazoxan | Millipore Sigma | Cat# I6138 | 1 μM |
Chemical compound, drug | Kynurenic acid | Millipore Sigma | Cat# K3375 | 1 mM |
Chemical compound, drug | MK-801 | Tocris Bioscience | Cat #0924 | 5 μM |
Chemical compound, drug | NASPM | Tocris Bioscience | Cat #2766 | 100 μM |
Chemical compound, drug | NBQX | Tocris Bioscience | Cat #1044 | 3 μM |
Chemical compound, drug | NMDG | Millipore Sigma | Cat# 66930 | 126 mM |
Chemical compound, drug | Noradrenaline | Tocris Bioscience | Cat #5169 | 30 μM |
Chemical compound, drug | Picrotoxin | Tocris Bioscience | Cat #1128 | 100 μM |
Chemical compound, drug | Prazosin | Millipore Sigma | Cat# P7791 | 100 nM |
Chemical compound, drug | Reserpine | Millipore Sigma | Cat# R0875 | 1 μM |
Chemical compound, drug | Strychnine | Millipore Sigma | Cat# S8753 | 10 μM |
Chemical compound, drug | Tetrodotoxin | Tocris Bioscience | Cat# 1069 | 1 μM |
Chemical compound, drug | WAY-100635 | Tocris Bioscience | Cat# 4380 | 300 nM |
Sequence-based reagent | Forward amplification primer | IDTDNA | tgattacgccaagctt GGTGGAGCTGTGTGGATGAAGC | - |
Sequence-based reagent | Forward sequence primer | IDTDNA | CCAGCCTGTGACCTCATGACC | - |
Sequence-based reagent | Reverse amplification primer | IDTDNA | gacggccagtgaattc CTTCAGCTGTCATGATAAGGTGATGTTG | - |
Commercial assay, kit | In-Fusion HD Cloning | Takara Bio Clontech | Cat# 639647 | - |
Software, algorithm | Clampfit 10.7 | Axon Instruments | RRID:SCR_011323 | https://www.moleculardevices.com/products/axon-patch-clamp-system |
Software, algorithm | EthoVision XT | Noldus Information Technology | RRID:SCR_000441 | https://www.noldus.com/ethovision-xt |
Software, algorithm | Fiji | PMID:22743772 | RRID:SCR_002285 | http://fiji.sc |
Software, algorithm | Prism 8 | GraphPad | RRID:SCR_002798 | http://www.graphpad.com |
Software, algorithm | VersaMax Analyzer | Omnitech-electronics, Inc | http://www.omnitech-electronics.com/product/VersaMax-Legacy-Open-Field---Locomotor-Activity/1930 | - |
Animals
All studies were conducted in accordance with the National Institutes of Health Guide for the Care and Use of Laboratory animals with the approval of the National Institute on Drug Abuse Animal Care and Use Committee. Wild-type C57BL/6J (>3 months old) mice of either sex were used. Mice were group-housed on a 12:12 hr reverse light cycle.
Brain slice preparation and electrophysiological recordings
Request a detailed protocolThe methods for brain slice preparation and electrophysiological recordings were almost identical to previous reports in the dorsal raphe (Gantz et al., 2015a) and ventral midbrain (Gantz et al., 2015b). In brief, mice were deeply anesthetized with isoflurane and killed by decapitation. Brains were removed quickly and placed in warmed artificial cerebral spinal fluid (modified Krebs’ buffer) containing (in mM): 126 NaCl, 2.5 KCl, 1.2 MgCl2, 1.2 CaCl2, 1.2 NaH2PO4, 21.5 NaHCO3, and 11 D-glucose with 5 μM MK-801 to reduce excitotoxicity and increase viability, bubbled with 95/5% O2/CO2. In the same solution, coronal dorsal raphe slices (220 μm) were obtained using a vibrating microtome (Leica 1220S) and incubated at 32°C > 30 min prior to recording.
Slices were then mounted in a recording chamber and perfused ~3 mL/min with ~35°C modified Krebs’ buffer. Electrophysiological recordings were made with a Multiclamp 700B amplifier (Molecular Devices), Digidata 1440A A/D converter (Molecular Devices), and Clampex 10.4 software (Molecular Devices) with borosilicate glass electrodes (King Precision Glass) wrapped with Parafilm to reduce pipette capacitance (Gantz and Bean, 2017). Pipette resistances were 1.8–2.8 MΩ when filled with an internal solution containing, (in mM) 104.56 K-methylsulfate, 5.30 NaCl, 4.06 MgCl2, 4.06 CaCl2, 7.07 HEPES (K), 3.25 BAPTA(K4), 0.26 GTP (sodium salt), 4.87 ATP (sodium salt), 4.59 creatine phosphate (sodium salt), pH 7.32 with KOH, mOsm ~285, for whole-cell patch-clamp recordings. Series resistance was monitored throughout the experiment. Transmitter release was evoked by trains of electrical stimuli delivered via a Krebs’ buffer-filled monopolar stimulating electrode positioned in the dorsal raphe, within 200 μm of the recorded neuron (Gantz et al., 2015a). Cell-attached recordings were made from quiescent neurons in slice, using pipettes filled with modified Krebs’ buffer. For experiments involving viral microinjections, transduced neurons were identified in the slice by visualization of eGFP. Reported voltages are corrected for a liquid junction potential of −8 mV between the internal solution and external solution. All drugs were applied by bath application. All experiments were conducted following incubation in an NMDAR channel blocker (MK-801, 5 μM,>1 hr), and then with AMPAR and KainateR (NBQX, 3 μM), GABA-AR (picrotoxin, 100 μM), and 5-HT1AR (WAY-100635, 300 nM) antagonists in the external solution. In addition, a α2-adrenergic receptor antagonist (idazoxan, 1 μM) was added for experiments where noradrenaline was applied and a glycine receptor antagonist (strychnine, 10 μM) was added when glycine was applied. Unitary current was calculated from fluctuation analysis, as previously described (Bean et al., 1990), assuming the macroscopic current arises from independent, identical channels with a low probability of opening, according probability theory; i = σ2/[I(1 p)] where i is unitary current, σ2 is the variance, I is mean current amplitude, and p is probability of opening.
Vector construction gRNA identification
Request a detailed protocolCRISPR SpCas9 gRNA target sites were identified in the mouse Grid1 gene (NC_000080.6) using CRISPOR (Haeussler et al., 2016). The seed sequence (GAACCCTAGCCCTGACGGCG) was chosen based on its relatively high specificity scores and the observation that it contains a Bgl I restriction enzyme site (GCCNNNN^NGGC) that overlaps with the Cas9 cleavage site.
Mouse Grid1 genotyping
Request a detailed protocolC57BL/6J mouse genomic DNA was isolated from tail biopsies or brain pieces containing microdissected dorsal raphe by digestion in DNA lysis buffer (50 mM KCl, 50 mM Tris-HCl (pH 8.0), 2.5 mM EDTA, 0.45% NP-40, 0.45% Tween-20, 0.5 ug/mL proteinase K) for 3 hr at 55°C, and 1 hr at 65°C. Lysates were then used as templates to amplify a 654 basepair fragment including the 390F gRNA target site using Q5 HotStart Master mix (New England Biolabs). A portion of the finished PCR reaction was treated with Bgl I restriction enzyme (New England Biolabs) for 60 min and processed on an AATI fragment analyzer.
Construction and packaging of AAV vectors
Request a detailed protocolThe AAV vector plasmid encoding SpCas9 (Swiech et al., 2015) (pX551) expressed from the Mecp2 promoter was a gift from Feng Zhang (Addgene plasmid # 60957, AAV-Cas9). The AAV packaging plasmid encoding a nuclear envelope-embedded eGFP reporter (Addgene 131682) was constructed by amplifying the KASH domain from (Addgene 60231, a gift of Feng Zhang) and fusing it (in-frame) to the end of coding region for eGFP in (Addgene 60058, pOTTC407) using ligation-independent cloning (AAV-empty, Figure 5—figure supplement 1A). gRNA was cloned into a mU6 expression cassette and then moved into an AAV backbone expressing a nuclear envelope-embedded (KASH-tagged) eGFP reporter (Addgene 131683) by PCR amplification and ligation-independent cloning (AAV-Grid1). Insert-containing clones were verified by sequencing and restriction fragment analysis prior to virus production. All AAV vectors were produced using triple transfection method as previously described (Howard and Harvey, 2017). All vectors were produced using serotype 1 capsid proteins and titered by droplet digital PCR.
Stereotaxic intracranial microinjections
Request a detailed protocolMice were anesthetized with a cocktail of ketamine/xylazine, immobilized in a stereotaxic frame (David Kopf Instruments), and received one midline injection of a 1:1 (v/v) cocktail of viruses AAV-Cas9 and AAV-empty or AAV-Grid1 for total volume 400 nL delivered over 4 min. The coordinates for injection were AP −4.4; ML 1.19, 20° angle; DV −3.62 mm, with respect to bregma. Prior to surgery, mice were injected subcutaneously with warm saline (0.5 mL) to replace fluid lost during surgery and given carprofen (5 mg/kg) post-surgery for pain relief. Mice recovered for >4 weeks to allow expression.
Behavioral assays
Request a detailed protocolBehavioral assays were conducted during the dark cycle, using 3 separate cohorts of AAV-Cas9 and AAV-empty or AAV-Grid1-injected mice as biological replicates, 30–55 d post-injection. To measure basal locomotion, mice were placed in locomotor boxes (VersaMax System, Omnitech Electronics, Inc) in a dark room for 1 hr, following prior habituation to the locomotor boxes for >2 d (1 hr/d). VersaMax Analyzer software was used to determine the total distance traveled, time spent moving, and velocity of movement in the last 30 min of the session. The boxes were cleaned with 70% ethanol and allowed to dry between trials. The elevated-plus maze was used to assay anxiety-related behaviors (Walf and Frye, 2007). The apparatus (Med associates, Inc) was placed 30 cm above the floor and consisted of two plastic light gray open arms (30 × 5 cm) and two black enclosed arms (30 × 5 cm) extending from a central platform (5 × 5 × 5 cm) at 90 degrees. Following habituation to the brightly lit room, mice were placed individually in the center of the maze, facing an open arm. Video tracking EthoVision XT software (Noldus Information Technology) was used to track mouse location, total distance traveled, velocity of movement, body elongation, and entries and time spent into the open and enclosed arms for each 5 min trial. Duration of head-dips, grooming, and enclosed-arm rearing were scored manually from videos played a 0.5x speed. Rearing in the enclosed arm was often associated with pressing one or both forepaws to the wall. Stretched-attend postures was defined by body elongation (70% threshold) and movement velocity <1 cm/s. No mice fell or jumped from the maze and open-arm rearing was not observed. The maze was cleaned with 70% ethanol after every trial and allowed to dry before the next trial. Mice were excluded from analysis if there was limited or no expression in the dorsal raphe, or if expression spread rostrally to the ventral tegmental area or caudally to locus coeruleus.
Immunohistochemistry and confocal microscopy
Request a detailed protocolFollowing behavioral assays, mice were euthanized with Euthasol and transcardially perfused with PBS followed by ice-cold 4% paraformaldehyde in PBS (pH 7.4). Brains were fixed overnight at 4 C and then sliced coronally in 50 μm sections. Alternatively, mice were anesthetized with isoflurane and euthanized by decapitation. Brains were removed and slices were prepared as for brain slice electrophysiology (220 μm), then fixed in room temperature 4% paraformaldehyde in PBS for 1 hr. Slices were mounted with Fluoromount-G with DAPI (Invitrogen) aqueous mounting medium. Confocal images were collected on an Olympus microscope (4x, 0.16 NA) and processed using Fiji.
Data analysis and visualization
Request a detailed protocolData were analyzed using Clampfit 10.7. Data are presented as representative traces, or in scatter plots where each point is an individual cell, and bar graphs with means ± SEM. In traces with electrical stimulation, stimulation artifacts have been blanked for clarity. Unless otherwise noted, n = number of distinct cells or mice as biological replicates. No sample was tested in the same experiment more than once (technical replication). Erevs were determined by linear regression for each cell. Recordings in which current did not cross 0 pA were omitted from analysis. To minimize space-clamp errors, analysis of current during voltage ramps was limited to −10 mV where the currents were typically less than 500 pA. Ramp currents were averaged in 2 mV bins (20 ms). Data sets with n > 30 were tested for normality with a Shapiro-Wilk test. When possible (within-group comparisons), significant differences were determined for two group comparisons by paired t-tests, Wilcoxon matched-pairs signed rank test, and in more than two group comparisons by nonparametric repeated-measures ANOVA (Friedman test). Significant mean differences in between-group comparisons were determined for two group comparisons by Mann Whitney tests, and in more than two group comparisons by Kruskal-Wallis tests. ANOVAs were followed, when p<0.05 by Dunn’s multiple comparisons post hoc test. Linear trends were analyzed using a mixed model ANOVA. A difference of p<0.05 was considered significant. For behavioral assays, Grubbs test was used to identify outliers. Basal locomotion and time spent in stretched-attend posture from one Grid1 mouse each were found to be outliers and were excluded from group comparisons. Exact values are reported unless p<0.0001 or>0.999. Statistical analysis was performed using GraphPad Prism 8 (GraphPad Software, Inc).
Data availability
All data analysed for this study are included in the manuscript.
References
-
Selective Expression of the Glutamate Receptor Channel δ2 Subunit in Cerebellar Purkinje CellsBiochemical and Biophysical Research Communications 197:1267–1276.https://doi.org/10.1006/bbrc.1993.2614
-
Reserpine suppression of dorsal raphe neuronal firing: mediation by adrenergic systemEuropean Journal of Pharmacology 52:27–36.https://doi.org/10.1016/0014-2999(78)90018-3
-
GluD1, linked to schizophrenia, controls the burst firing of dopamine neuronsMolecular Psychiatry 23:691–700.https://doi.org/10.1038/mp.2017.137
-
The emergence of NMDA receptor metabotropic function: insights from imagingFrontiers in Synaptic Neuroscience 8:20.https://doi.org/10.3389/fnsyn.2016.00020
-
Bipolar I disorder and schizophrenia: a 440-single-nucleotide polymorphism screen of 64 candidate genes among ashkenazi jewish case-parent triosThe American Journal of Human Genetics 77:918–936.https://doi.org/10.1086/497703
-
Evaluation of copy number variations reveals novel candidate genes in autism spectrum disorder-associated pathwaysHuman Molecular Genetics 21:3513–3523.https://doi.org/10.1093/hmg/dds164
-
Glutamate receptors of the Delta family are widely expressed in the adult brainBrain Structure and Function 220:2797–2815.https://doi.org/10.1007/s00429-014-0827-4
-
Localized IP3-evoked Ca2+ release activates a K+ current in primary vagal sensory neuronsJournal of Neurophysiology 91:2344–2352.https://doi.org/10.1152/jn.01008.2003
-
Assaying the stability and inactivation of AAV serotype 1 vectorsHuman Gene Therapy Methods 28:39–48.https://doi.org/10.1089/hgtb.2016.180
-
Synaptic plasticity of NMDA receptors: mechanisms and functional implicationsCurrent Opinion in Neurobiology 22:496–508.https://doi.org/10.1016/j.conb.2012.01.007
-
An introduction to the endogenous cannabinoid systemBiological Psychiatry 79:516–525.https://doi.org/10.1016/j.biopsych.2015.07.028
-
Reward processing by the dorsal raphe nucleus: 5-ht and beyondLearning & Memory 22:452–460.https://doi.org/10.1101/lm.037317.114
-
Voltage clamp analysis of cholinergic action in the HippocampusThe Journal of Neuroscience 7:733–741.https://doi.org/10.1523/JNEUROSCI.07-03-00733.1987
-
The Delta subfamily of glutamate receptors: characterization of receptor chimeras and mutantsEuropean Journal of Neuroscience 37:1620–1630.https://doi.org/10.1111/ejn.12193
-
Alpha 1-adrenoceptors in rat dorsal raphe neurons: regulation of two potassium conductancesThe Journal of Physiology 478 Pt 3:437–447.https://doi.org/10.1113/jphysiol.1994.sp020263
-
Induction of Fos immunoreactivity in the brain by exposure to the elevated plus-mazeBehavioural Brain Research 56:115–118.https://doi.org/10.1016/0166-4328(93)90028-O
-
In vivo interrogation of gene function in the mammalian brain using CRISPR-Cas9Nature Biotechnology 33:102–106.https://doi.org/10.1038/nbt.3055
Article and author information
Author details
Funding
NIH Center on Compulsive Behaviors (Center on Compulsive Behaviors Fellowship)
- Stephanie C Gantz
National Institute on Drug Abuse (1ZIADA000612)
- Stephanie C Gantz
- Khaled Moussawi
- Holly S Hake
The funders had no role in study design, data collection and interpretation, or the decision to submit the work for publication.
Acknowledgements
This work was supported by the Intramural Research Program at the National Institute on Drug Abuse 1ZIADA000612 (SCG, HSH, KM) and the Center on Compulsive Behaviors, National Institutes of Health via NIH Director’s Challenge Award to SCG. Construction and packaging of AAV viral vectors, and guide RNA selection, validation, and PCR-Bgl I analysis was performed by the Genetic Engineering and Viral Vector Core of the National Institute on Drug Abuse. We thank Drs. John T Williams and Bruce P Bean for comments on this manuscript, and Ms. Maria M Ortiz for assistance with the behavioral assays. The opinions expressed in this article are the authors’ own and do not reflect the views of the NIH/DHHS.
Ethics
Animal experimentation: This study was conducted in accordance with the National Institutes of Health Guide for the Care and Use of Laboratory animals. The protocol was approved by the National Institute on Drug Abuse Animal Care and Use Committee.
Copyright
This is an open-access article, free of all copyright, and may be freely reproduced, distributed, transmitted, modified, built upon, or otherwise used by anyone for any lawful purpose. The work is made available under the Creative Commons CC0 public domain dedication.
Metrics
-
- 3,082
- views
-
- 389
- downloads
-
- 40
- citations
Views, downloads and citations are aggregated across all versions of this paper published by eLife.
Citations by DOI
-
- 40
- citations for umbrella DOI https://doi.org/10.7554/eLife.56054