circZNF827 nucleates a transcription inhibitory complex to balance neuronal differentiation
Abstract
Circular RNAs are important for many cellular processes but their mechanisms of action remain poorly understood. Here, we map circRNA inventories of mouse embryonic stem cells, neuronal progenitor cells and differentiated neurons and identify hundreds of highly expressed circRNAs. By screening several candidate circRNAs for a potential function in neuronal differentiation, we find that circZNF827 represses expression of key neuronal markers, suggesting that this molecule negatively regulates neuronal differentiation. Among 760 tested genes linked to known neuronal pathways, knockdown of circZNF827 deregulates expression of numerous genes including nerve growth factor receptor (NGFR), which becomes transcriptionally upregulated to enhance NGF signaling. We identify a circZNF827-nucleated transcription-repressive complex containing hnRNP-K/L proteins and show that knockdown of these factors strongly augments NGFR regulation. Finally, we show that the ZNF827 protein is part of the mRNP complex, suggesting a functional co-evolution of a circRNA and the protein encoded by its linear pre-mRNA host.
Introduction
The mammalian non-coding transcriptome, which includes long noncoding RNAs (lncRNAs) and circular RNAs (circRNAs), plays pivotal roles in biological decisions during differentiation and normal cell maintenance (reviewed in Chekulaeva and Rajewsky, 2019; Deveson et al., 2017; Kopp and Mendell, 2018). Even though circRNAs were already identified several decades ago (Capel et al., 1993; Kos et al., 1986; Nigro et al., 1991; Sanger et al., 1976), they only recently have emerged as a large class of abundant noncoding RNAs that exhibit cell-type- and tissue-specific expression patterns (Ashwal-Fluss et al., 2014; Hansen et al., 2013; Jeck et al., 2013; Memczak et al., 2013; Rybak-Wolf et al., 2015; Salzman et al., 2013; Salzman et al., 2012) (reviewed in Chekulaeva and Rajewsky, 2019; Ebbesen et al., 2017; Salzman, 2016). CircRNAs are generated by the canonical spliceosome in a non-linear backsplicing fashion (Cocquerelle et al., 1993; Jeck et al., 2013; Memczak et al., 2013; Pasman et al., 1996; Salzman et al., 2012). During circRNA biogenesis, flanking intronic sequences are thought to bring splice sites within critically close proximity, either by direct basepairing between inverted repeats (e.g. Alu-repeats) or facilitated by interactions between flanking intron-bound RNA-binding proteins (RBPs) (Ashwal-Fluss et al., 2014; Conn et al., 2015; Ebbesen et al., 2017). Most circRNAs are primarily localized to the cell cytoplasm (Ashwal-Fluss et al., 2014; Hansen et al., 2013; Jeck et al., 2013; Memczak et al., 2013; Rybak-Wolf et al., 2015; Salzman et al., 2012), and recent evidence suggests that nuclear export of circRNAs in human cells is influenced by the size of the given molecules, where larger circRNAs (>800 nucleotides) are dependent on DExH/D-box helicase UAP56 (DDX39B), whereas smaller species are dependent on URH49 (DDX39A) (Huang et al., 2018).
Several reports have provided evidence that circRNAs play important roles in various fundamental cellular processes. Well-described examples are the CDR1as/ciRS-7 and Sry circRNAs that function to negatively regulate Mir7-1 and Mir138-1 activity, respectively, by sequestration (miRNA sponging), leading to increased mRNA expression of their respective miRNA-targets (Hansen et al., 2013; Memczak et al., 2013). However, it has also been suggested that the majority of circRNAs are likely not bona fide miRNA sponges, simply due to relatively low copy numbers and a low number of miRNA-binding sites per molecule, leaving efficient miRNA regulation ambigous in many cases (Chekulaeva and Rajewsky, 2019; Ebbesen et al., 2017). Examples of circRNAs acting as binding scaffolds for RBPs, or RBP sponges, which in turn affect their canonical function in for example pre-mRNA splicing and protein translation, have been reported (Abdelmohsen et al., 2017; Ashwal-Fluss et al., 2014). Nuclear variants coined exon-intron circular RNAs (EIciRNAs), have, due to their retention of intronic sequences, been shown to promote transcription by recruitment of U1 snRNP to transcription units by a not fully clarified mechanism (Li et al., 2015). Many abundant circRNAs originate from the 5’ end of their precursor transcripts, often giving rise to backsplicing into parts of the 5’UTR of their linear relative (Jeck et al., 2013; Memczak et al., 2013; Rybak-Wolf et al., 2015). The prevalence af these AUG circRNAs suggests that at least a subset of circRNAs could have protein-coding potential via a cap-independent translation mechanism (Stagsted et al., 2019). This is consistent with both early studies of Internal Ribosome Entry Sites (IRES) placed in a circRNA context (Chen and Sarnow, 1995), as well as more recent studies reporting examples of translation-competent circRNAs (AbouHaidar et al., 2014; Legnini et al., 2017; Pamudurti et al., 2017; Yang et al., 2017). However, global analyses of hundreds of ribosome profiling and mass-spec datasets, suggests that these few examples are specialized events, and not a generally applicable function of circRNAs (Stagsted et al., 2019).
RNA-sequencing of RNA isolated from mouse and human tissues along with various cell lines suggests that circRNAs are most abundantly expressed in the brain, compared to other tissues and that circRNAs are particular enriched in neuronal synaptosomes (Rybak-Wolf et al., 2015). In line with this, cells derived from both embryonal carcinoma (P19) and neuroblastoma (SHSY-5Y) subjected to neuronal/glial differentiation show tightly regulated circRNA expression profiles during neuronal development, that include upregulation of numerous common circRNAs (Rybak-Wolf et al., 2015). Piwecka et al., demonstrated that a ciRS-7 knockout mouse displayed downregulated Mir7-1 levels, alterations in sensorimotor gating associated with neuropsychiatric disease and abnormal synaptic transmission, suggesting that ciRS-7 and Mir7-1 are important for normal brain function in the mouse (Piwecka et al., 2017). Adding to the complexity of this regulatory network, a long noncoding RNA (lncRNA), Oip5os1 (also coined Cyrano), promotes the destruction of Mir7-1, which in turn upregulates ciRS-7 by a still unidentified mechanism (Kleaveland et al., 2018). One circRNA, circSlc45a4, which is very abundant in the cortex of the mouse and human brain, has recently been shown to negatively regulate neuronal differentiation, both in cell cultures and in developing mice, where its knockdown dysregulates the balance between specialized cortex neurons by unknown molecular mechanisms (Suenkel et al., 2020).
Despite these intricate molecular interactions between circRNA, miRNA, and lncRNA, many important questions regarding neuronal differentiation and function remain unanswered. For example, it is largely unknown how the tightly controlled expression of circRNAs potentially affects neuronal development. Here, we present the circRNA inventory of mouse embryonic stem cells (mESC), neuronal progenitor cells (NPC) and differentiated glutamatergic neurons, which represents a well-established model for CNS-type neuronal differentiation (Bibel et al., 2007). We report thousands of RNase R-resistant circRNAs of which many are differentially regulated during neuronal development. In a screen for circRNA function using an established human model for neuronal differentiation, we identify circZNF827 as a negative regulator of neuronal differentiation. Although being almost exclusively localized to the cell cytoplasm, the nuclear population of this circRNA impacts several genes of relevance in neuronal differentiation, at the level of transcription, including nerve growth factor receptor (NGFR), which becomes robustly upregulated upon circZNF827 knockdown. Mechanistically, our evidence suggests that circZNF827 is a necessary scaffold for a transcription-repressive complex containing its own host-encoded protein; ZNF827, together with hnRNP K and hnRNP L.
Results
The circRNA profile of mESCs changes markedly upon neuronal differentiation
To determine whether circRNAs can influence neuronal differentiation, we initially mapped the circRNA inventories at different stages of neuronal differentiation and compared these to other available circRNA datasets of neuronal origin from mice and humans (Rybak-Wolf et al., 2015). Identification of circRNAs from RNA-seq experiments has often been based on quantification of relatively few reads across the circRNA backsplicing junction (circBase Glažar et al., 2014), and current circRNA prediction algorithms inevitably lead to the calling of false positives (Hansen et al., 2016; Jeck and Sharpless, 2014). Hence, to immediately validate the circular nature of to-be called circRNAs, we first performed standard rRNA depletion and subsequently either included or excluded RNase R treatment step prior to RNA-sequencing. Specifically, we used an established differentiation model for CNS-type glutamatergic neurons, based on E14 mouse embryonic stem cells (mESCs) that reportedly yields a purity of glutamatergic neurons of >90% (Bibel et al., 2007). RNA was isolated from three stages of differentiation, mESCs, neuronal progenitor cells (mNPCs) or neuronal differentiation day 8 (mN8) and rRNA depleted (+/- RNase R) prior to library preparation and RNA-seq (Figure 1A). Successful differentiation at the mNPC and mN8 stages was confirmed by the appearance of elongated intercellular dendritic extensions (mN8) (Figure 1—figure supplement 1A) and robust upregulation of several classical neuronal markers including, Ntrk2, Map2, and Tubb3 (mNPC and mN8), while stem cell pluripotency marker Nanog became significantly reduced upon differentiation (Figure 1—figure supplement 1B). Using available circRNA prediction tools CIRI2 (Gao et al., 2018), find_circ (Memczak et al., 2013) and CIRCexplorer2 (Zhang et al., 2016) on the non-RNase R-treated RNA, we identified between 792–1167 circRNAs in mESC, 2230–2893 circRNAs in NPC and 1902–2316 circRNAs in differentiated neurons at mN8 stage (Figure 1B). Upon RNase R treatment most circRNAs either remained unchanged or became enriched, but a considerable fraction of the predicted circRNAs in mESC, mNPC, and mN8 preparations, became depleted by the 3’−5’ exonuclease (CIRCexplorer2: 19.5–36.5%; CIRI2: 7.2–16.6%; find_circ: 38.7–52.3% depleted) (Figure 1—figure supplement 1C). All prediction algorithms showed a correlation between expression level and RNase R resistance, suggesting that mostly low-count circRNAs candidates are likely false positives (Figure 1—figure supplement 1D). From a total of 3581 enriched circRNAs after RNase R treatment (all stages), 1449 circRNAs overlapped between all three circRNA prediction algorithms, and this subset represents a high-confidence circRNA inventory (Hansen et al., 2016; Figure 1C and Supplementary file 1). We next assessed the circular-to-linear ratio of identified circRNAs (find_circ), by comparing splice site usage in circular vs. linear splicing events (Memczak et al., 2013; Rybak-Wolf et al., 2015). This analysis revealed vast differences in the steady-state levels of these isoforms and demonstrated that many circRNA species are considerably more abundant than their linear precursors (Figure 1D). Confirming previous results (Rybak-Wolf et al., 2015), introns flanking the circRNAs are generally longer than average introns and circRNAs often tend to cluster at the 5’ end of their respective precursor RNA (Figure 1—figure supplement 1E–F). Our results suggest that in order to obtain high confidence circRNA inventories from RNA-seq data, it is beneficial to use multiple circRNA prediction algorithms and to enrich for bona fide circRNAs, by depletion of linear RNAs using RNase R.
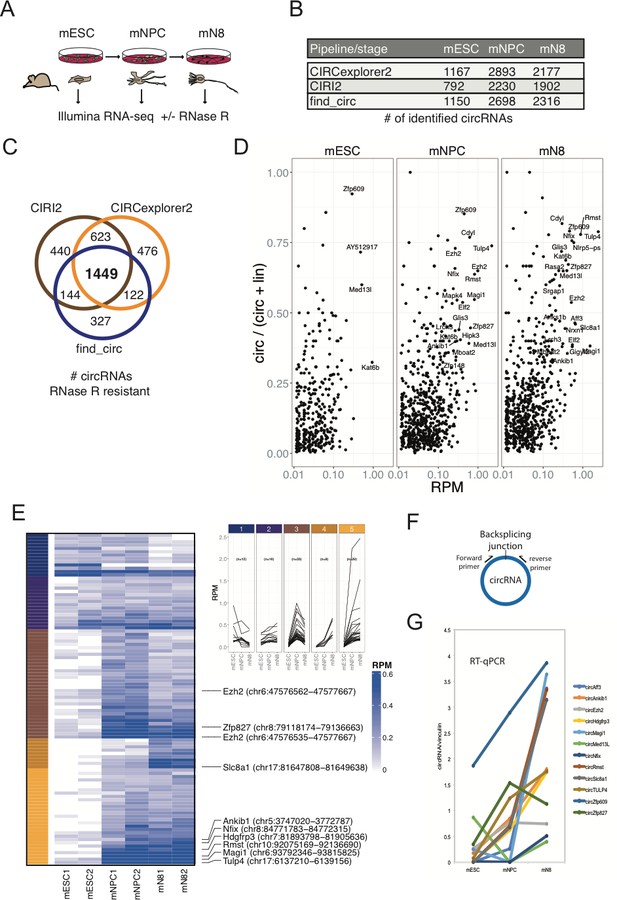
Determining the circRNA inventories of mESC, NPC and differentiated glutamatergic neurons and their differential regulation.
(A) Schematic illustration of workflow for differentiation and RNA-seq. (B) Number of circRNAs detected by indicated circRNA prediction algorithm in different stages. (C) Venn-diagram showing 1449 common circRNAs of a total of 3581 circRNAs predicted by the different algorithms (as indicated next to the diagram) that are either constant or enriched upon RNase R treatment. (D) circRNA/circRNA+linear precursor ratios as a function of expression level (RPM) at the three sequenced stages. (E) Left: Heatmap showing differential expression of top-100 expressed circRNAs (RPM scale to the right), with selected examples of circRNAs as indicated along with genomic coordinates (mm10). Top: K-means analysis displaying five different expression profiles during differentiation (same color code given to the left of the heatmap). (F) circRNA RT-qPCR strategy spanning the backsplicing junction. (G) RT-qPCR validaton of selected circRNAs. Data are depicted as mean ± SD (two biological replicates).
We next tested differential circRNA expression during differentiation, which revealed marked changes in circRNA expression over the 16-day timecourse (Figure 1E; left panel). Kmeans clustering of circRNAs by expression (Top 100 highest expressed) pattern showed two main clusters with peak expression at mNPC and mN8 (Figure 1E; right panel). Comparison with previously identified mouse and human homologue circRNAs, isolated from mouse brain regions or cell lines of either murine or human origin (Rybak-Wolf et al., 2015), revealed a substantial overlap between circRNAs at differentiated stages (e.g. 80% of all 1449 circRNAs found in differentiated murine p19 cells and primary neurons, 45% of Top100 found in human SH-SY5Y and 75% overlap with circRNAs found in the human ENCODE data previously analyzed Rybak-Wolf et al., 2015; Stagsted et al., 2019; Figure 1—figure supplement 1G). We confirmed differential expression of a subset of the most abundant and upregulated circRNAs (circTulp4, circMagi1, circRmst, circEzh2, circHdgfrp3, circZfp827, circMed13l, circZfp609, circSlc8a1, circNfix) using RT-qPCR with amplicons across the backsplicing junction (Figure 1F–G). 75% of the top-100 expressed mouse circRNAs was also found in human circRNA datasets (Rybak-Wolf et al., 2015; Figure 1—figure supplement 1G). We conclude that significant changes in circRNA expression patterns are induced upon neuronal differentiation and that the majority of these circRNAs are conserved between various neuronal cell-types originating from humans and the mouse.
Knockdown of circZNF827 stimulates neuronal marker expression
To ascertain whether the highly upregulated circRNAs might contribute to the process of neuronal differentiation, we next depleted a number of candidate circRNAs by RNA interference. We first tested knockdown efficiency of circZfp827 (circZNF827 in humans) by lentivirally delivered dishRNAs (Kaadt et al., 2019) targeting the backsplicing junction in either mESC, p19, SH-SY5Y or L-AN-5 cells, of which the latter three cell lines are well-established models of neuronal differentiation following retinoic acid treatment. Knockdown efficiency in mESC and p19 proved relatively poor (30–60% remaining circRNA) compared to the two human cell lines: SH-SY5Y (10% remaining) and L-AN-5, which displayed superior results (<8% remaining) (Figure 2A and Figure 2—figure supplement 1A). Moreover, when testing SH-SY5Y cells for an increase of neuronal differentiation markers NTRK2, NEFL, MAP2 and TUBB3 upon retinoic acid treatment, only NTRK2 was significantly upregulated upon differentiation (Figure 2—figure supplement 1B), whereas these genes showed a more expected and dynamic expression pattern in L-AN-5 cells (Figure 2B). We therefore transduced L-AN-5 cells with lentiviral dishRNA vectors to perform knockdown of 14 candidate circRNAs (Figure 2A and Figure 2—figure supplement 1C; circTULP4, circSLC8A1, circZNF609, circHDGFRP3, circMAGI, circRMST, circZNF827, circANKIB1, circMED13L, circCDYL, circUNC79, circHIPK3, circNFIX, circCAMSAP1) (Supplementary file 2a) and subsequently subjected these to retinoic acid-induced differentiation followed by neuronal marker quantification in order to probe for changes in differentiation. In general, we observed efficient knockdown (Figure 2A and Figure 2—figure supplement 1C). While the majority of knockdowns did not significantly change neuronal marker expression, knockdown of circZNF827 (and to a lesser extent circANKIB1), produced a significant and reproducible increase in neuronal marker expression upon differentiation (Figure 2B and Figure 2—figure supplement 2A). Importantly, the linear ZNF827 mRNA was not affected by backsplicing junction-specific knockdown (Figure 2—figure supplement 2B). The upregulation of neuronal markers following circZNF827 knockdown was also evident at the protein level for MAP2 and TUBB3 (Figure 2C, and quantified to the right). In addition, proliferation assays demonstrated a smaller S-phase population (32% to 24%) upon circZNF827 knockdown, suggesting lowered replication kinetics (Figure 2D and Figure 2—figure supplement 3A–B). This phenomenon was accompanied by a minor stall in G2/M phase, while G0/G1 phase was not significantly affected between control and circZNF827 knockdown. Taken together, our results suggest that circZNF827 knockdown exerts a repressive effect on proliferation, while it enhances neuronal marker expression and hence differentiation.
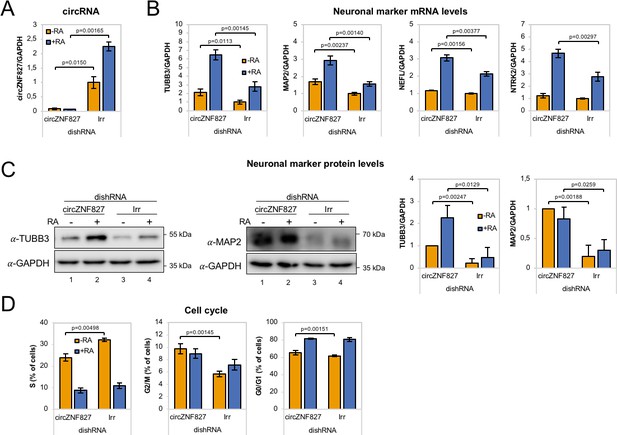
circZNF827 regulates neuronal marker expression levels.
(A) RT-qPCR analysis evaluating knockdown of circZNF827 with dicer-independent short hairpin RNAs (dishRNAs) in the neuroblastoma cell line L-AN-5. (B) Relative mRNA levels of the neuronal markers TUBB3, MAP2, NEFL, and NTRK2 evaluated by RT-qPCR upon knockdown of circZNF827. The mRNA expression levels were evaluated by RT-qPCR after 4 days of RA-mediated neuronal differentiation. (C) Western blotting (left panels) of TUBB3 and MAP2 upon circZNF827 knockdown. GAPDH was used as loading control. The results of quantification of band intensities from western blots are shown the right panels. One representative western blot and the quantification of three is shown. (D) Cell cycle assay based on flow cytometric measurements of EdU incorporation into newly synthesized DNA in L-AN-5 cells upon circZNF827 knockdown. +RA: differentiated L-AN-5 cells. -RA: undifferentiated L-AN-5 cells. Irr: Irrelevant dishRNA. In all panels, data are depicted as mean ± SD (three biological replicates). p-Values were determined by a two-tailed Student’s t test.
circZNF827 controls retinoic acid receptor homeostasis
We next asked whether the retinoic acid receptors (RARs), which represent central nodes in relaying anti-proliferative differentiation cues during neuronal development (Gudas and Wagner, 2011), and are key targets of retinoic acid (RA), also become upregulated upon knockdown of circZNF827. Indeed, knockdown of circZNF827 leads to a moderate but significant increased expression (1.5–2.5 fold) of RARB and RARG, while RARB remained constant (Figure 3A). Since most circRNAs have been reported to predominantly localize in the cell cytoplasm, we addressed the localization of circZNF827, circANKIB1 and circTULP4 by cellular fractionation. These circRNAs are mainly cytoplasmically localized in L-AN-5 cells (~90% cytoplasmic signal) (Figure 3B). We therefore hypothesized that circZNF827 could potentially affect RAR-mRNA stability post-transcriptionally in the cell cytoplasm. However, BrU pulse-chase mRNA decay assays demonstrated no significant change in RAR-mRNA decay rates upon knockdown of circZNF827 (Figure 3C). Next, we investigated transcription rates, by treating cells with a short pulse of BrU, followed by BrU immunoprecipitation to quantify de novo labeled RNA, serving as a proxy for transcription rates during control- or knockdown of circZNF827. As expected from the constant mRNA decay rates, BrU incorporation was moderately upregulated, although significantly only for RARA, upon circZNF827 knockdown (Figure 3—figure supplement 1). Although this moderate increase in transcription measured at a single late time-point, cannot readily explain the larger effect on the RARA mRNA level (2.5-fold) measured at steady-state, our results suggest that circZNF827 contributes to controlling the RARA receptor levels in order to keep neuronal differentiation in check.
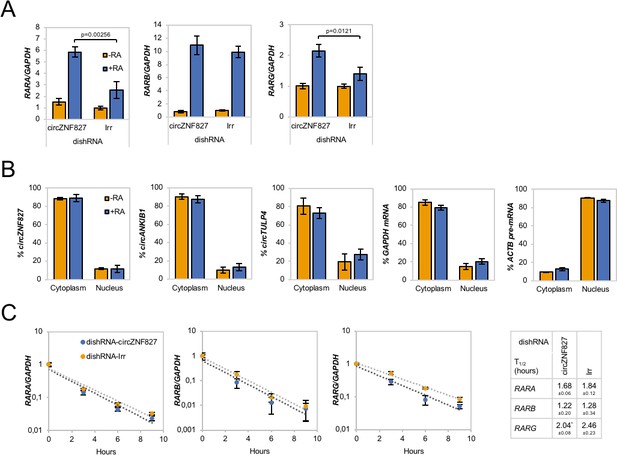
Increased RAR expression upon circZNF827 knockdown.
(A) mRNA expression levels of the RAR receptors RARA, RARB, and RARG in L-AN-5 cells upon circZNF827 knockdown evaluated by RT-qPCR. (B) Subcellular localization of the circRNAs circZNF827, circANKIB1, and circTULP4 examined by RT-qPCR after fractionation of differentiated L-AN-5 cells into cytoplasmic and nuclear fractions. GAPDH mRNA and ACTB pre-mRNA levels was used for validation of the purity of the cytoplasmic and nuclear fractions. (C) BrU pulse-chase mRNA decay assay evaluating decay rates of RAR mRNAs upon circZNF827 knockdown. The RAR mRNA expression levels were evaluated by RT-qPCR. In right panel, half-lives of the RARs obtained in the experiment are indicated. +RA: differentiated L-AN-5 cells. -RA: undifferentiated L-AN-5 cells. Irr: Irrelevant dishRNA. In all panels data are depicted as mean ± SD (three biological replicates). p-Values were determined by a two-tailed Student’s t test.
circZNF827 knockdown affect multiple genes in neuronal signaling
Our results indicate that L-AN-5 cells are lowering their proliferation rates and promote RAR-signaling by transcriptional upregulation of one of these transcription factors when circZNF827 levels are low. To test how circZNF827 knockdown affects other key factors of the neuronal transcriptome, we next performed Nanostring analyses using a neuro-differentiation/pathology panel of 760 genes with RNA purified from differentiated or non-differentiated L-AN-5 cells. 135 genes become differentially expressed (9 upregulated and 126 downregulated, fold change > +/- 2, p<0.05) due to circZNF827 knockdown after differentiation (Figure 4A, Supplementary file 2b). In line with a potential negative regulatory function of circZNF827 on neuronal differentiation, GO-term analyses show enrichment of terms including axon/dendrite structure, neural cytoskeleton, transmitter synthesis, neural connectivity, growth factor signaling and trophic factors among differentially expressed genes (Figure 4B). The most significantly upregulated gene is nerve growth factor receptor (NGFR), which plays a central role in regulating neuronal differentiation, death, survival, and neurite outgrowth (Yamashita et al., 1999; Zhu et al., 2012). Conversely, Phosphatase and tensin homolog (PTEN), STAT3 and NAD(P)H quinone dehydrogenase 1 (NQO1) were all significantly downregulated upon circZNF827 knockdown (2–4 fold), which reportedly also contributes positively to neuronal differentiation (Lyu et al., 2015; Ma et al., 2017), and in case of the latter, also renders cells more susceptible to energetic and proteotoxic stress (Hyun et al., 2012). Since NGFR is a key regulator of neuronal differentiation and the highest upregulated gene upon circZNF827 knockdown, we next focused on the mechanism of its upregulation. Using both RT-qPCR and western blotting, demonstrated a strong upregulation at both the protein and mRNA level (Figure 4C and Figure 4—figure supplement 1). This upregulation was not due to changes in mRNA decay rates, since BrU pulse-chase mRNA decay assays yielded nearly identical mRNA half-lives upon circZNF827 knockdown (Figure 4D). To address whether the observed changes in gene expression are elicited at the transcriptional level, we subjected cells to a short BrU-pulse prior to BrU immunoprecipitation and Nanostring hybridization. Interestingly, NGFR and also ATP8A2 proved to be highly upregulated (~4–6 fold) at the level of transcription (Figure 4E–F), while only NQO1 and not PTEN and STAT3 exhibited significantly reduced transcription activity (ranging from ~1.3- to ~4-fold) (Figure 4E). Also, the MAP2 gene did not change its de novo RNA output, suggesting that the tuning of the steady-state levels of PTEN, STAT3 and MAP2 mRNAs, as initially observed (Figures 2B and 4A), are mainly facilitated by posttranscriptional changes to mRNA stability. If circZNF827 is involved in a direct transcription-associated complex that regulates NGFR output, the transcriptional effects elicited by circZNF827 knockdown would require nuclear knockdown of the circRNA. Indeed, the use of dicer-independent shRNA (dishRNA) vectors proved very efficient in depleting nuclear circRNA (Figure 4G).
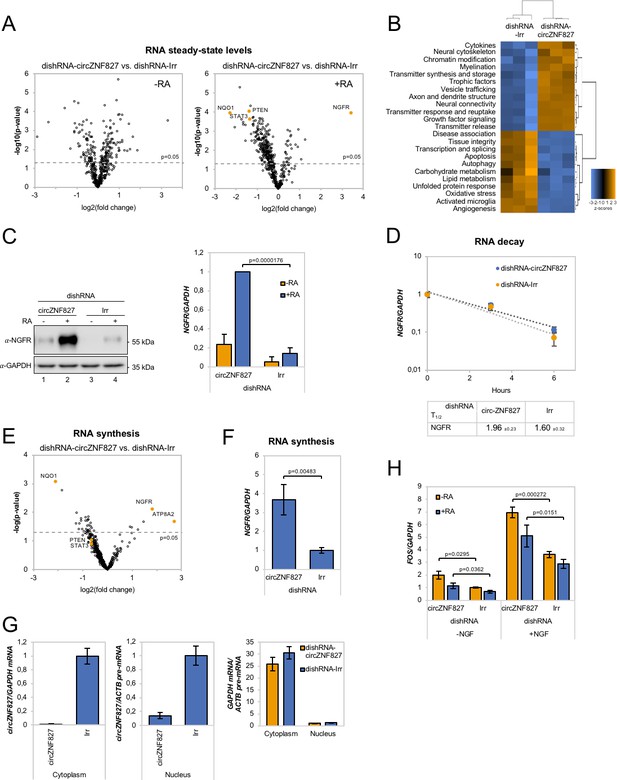
circZNF827 regulates NGFR expression.
(A) Volcano plot based on a Nanostring analysis of the expression of ~800 neuropathology-related genes upon circZNF827 knockdown in L-AN-5 cells vs control without RA treatment (left panel) or with RA treatment (right panel). (B) GO-term analysis based on genes found differentially expressed by the Nanostring analysis upon circZNF827 knockdown in differentiated L-AN-5 cells. (C) Western blotting (left panel) of NGFR upon circZNF827 knockdown in L-AN-5 cells. GAPDH was used as loading control. The result of quantification of band intensities from the western blots is shown in the right panel. One representative western blot and the quantification of three is shown. (D) BrU pulse-chase mRNA decay assay evaluating decay rates of NGFR mRNAs upon circZNF827 knockdown. In the bottom panel, the half-lives of NGFR obtained in the experiment are indicated. (E) Volcano plot showing mRNAs with changed synthesis rates estimated after BrU-labeling of newly synthesized RNA by Nanostring analysis using the neuropathology panel. (F) RT-qPCR-based validation of the Nanostring analysis shown in (E). (G) Evaluation of circZNF827 knockdown in L-AN-5 cells after subcellular fractionation into nuclear and cytoplasmic RNA fractions by RT-qPCR. GAPDH mRNA and ACTB pre-mRNA levels was used for validation of the purity of the cytoplasmic and nuclear fractions. (H) FOS mRNA levels evaluated by RT-qPCR after circZNF827 knockdown and NGF stimulation of L-AN-5 cells. +RA: differentiated L-AN-5 cells. -RA: undifferentiated L-AN-5 cells. Irr: Irrelevant dishRNA. Data are depicted as mean ± SD (three biological replicates). (D–F) One representative western blot is shown. p-Values were determined by a two-tailed Student’s t test.
Next, we assayed the cellular impact of NGFR upregulation upon circZNF827 knockdown. To this end, we NGF-treated L-AN-5 cells subjected to either control or circZNF827 knockdown, and quantified downstream signaling output by quantification of FOS, which is a well-known downstream ‘immediate early’ target of NGFR signaling. FOS levels increased significantly, strongly indicating that the higher levels of NGFR protein indeed leads to functional increase in NGFR signaling (Figure 4H and Figure 4—figure supplement 1), which can at least in part explain the upregulation of neuronal markers. Taken together, we conclude that circZNF827 serves to keep neuronal differentiation ‘in check’ by limiting expression of, and signaling by, RARA and NGFR.
circZNF827 interacts with transcriptional regulators hnRNP K and -L
To address the mechanism by which these transcriptional and post-transcriptional events are controlled by circZNF827, we next sought to identify its protein interactome. To this end, we synthesized biotin-labeled circZNF827 (linear version) and control RNAs (circTULP4, circZNF609, circHDGFRP3 and circSLC8A1) in vitro and subjected these to pull-down experiments using L-AN-5 cell lysates and streptavidin-coupled magnetic beads as previously described (Seitz et al., 2017). Silver-stained SDS-PAGE gels of retained proteins revealed unique profiles, suggesting that specific proteins exhibited increased affinity toward circZNF827, although prominent RNA-binding proteins common to both control RNAs and circZNF827 could also be observed (Figure 5—figure supplement 1A). By subjecting pulled-down fractions to LC-MS/MS, we identified several circZNF827-specific proteins, including hnRNP K and -L, while others (e.g. DHX9 and DDX3X) bound strongly to any of the bait RNAs (Figure 5A). To validate these interactions, we performed RNA-immunoprecipitaiton (RIP) using monoclonal anti-hnRNP K or -L antibodies followed by qRT-PCR across the backsplicing junction, and observed a significant enrichment of circZNF827 compared to IgG controls (>100 fold), suggesting that these interactions can be recapitulated in L-AN-5 cells (Figure 5B). As expected for these highly expressed RNA-binding proteins, both proteins associate with GAPDH mRNA, but in the case of hnRNP L, the IP/input ratios were ~18 fold higher for circZNF827, whereas hnRNP K displayed a similar enrichment of GAPDH mRNA as of circZNF827 (Figure 5B). Scrutinizing the circZNF827 sequence for putative-binding sites for hnRNP K and -L using eCLIP datasets (ENCODE consortium), proved unfeasible due to low expression levels of the ZNF827 gene in the K562 and HepG2 cells used by ENCODE. Using RBPmap (Paz et al., 2014), which is based on established RBP consensus binding sequences, revealed a potential high-affinity cluster for primarily hnRNP L binding and one site for hnRNP K in the most 3’ part of the circle-encoding sequence (Figure 5—figure supplement 1B–C). According to circZNF827 secondary RNA structures predicted by Mfold, these binding sites are located into mostly single-stranded regions within the circRNA, consistent with the binding preferences of most hnRNP proteins toward single-stranded RNA (Figure 5—figure supplement 1D). To further characterize these interactions, we prepared a stable HEK293 Flp-In T-rex cell-line expressing circZNF827 (Figure 5—figure supplement 1E–F) under the control of a tetracycline-inducible promoter (tet-on), based on the laccase vector system (Kramer et al., 2015). We then performed RIP by immunoprecipitation of endogenous hnRNP K or -L and observed a remarkable enrichment of exogenous circZNF827, compared to control IgG or GAPDH mRNA (Figure 5C and E). hnRNP L gave a particularly high IP/Input ratio (>200 fold enrichment over IgG), consistent with the results from: (1) the L-AN-5 RIP, (2) the pull-down LC-MS/MS experiment and (3) the prediction of several hnRNP L binding site clusters in circZNF827. To test whether the predicted hnRNP K/- L binding sites in the 3′ part of circZNF827 are indeed sites of interaction, we constructed a deletion mutant that removes the putative binding sites (Figure 5—figure supplement 1C, dark grey letters and Figure 5—figure supplement 1D, dotted dark grey line) and prepared HEK293 stable cell lines (Figure 5—figure supplement 1E–F). Although this mutant was expressed at somewhat lower levels than WT circZNF827, it remained virtually unbound by hnRNP K/- L similar to IgG pull-down efficiency (Figure 5C).
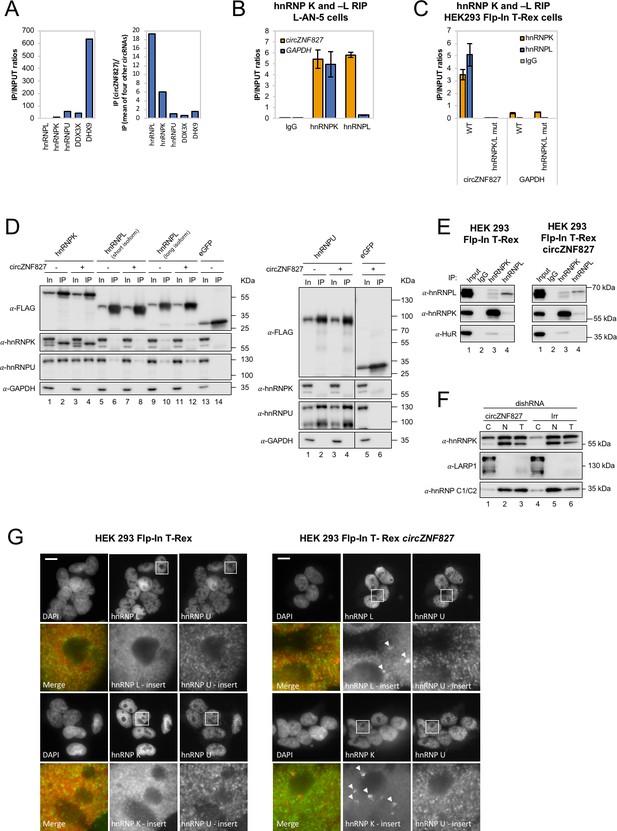
circZNF827 interacts with and regulates the subcellular localization of hnRNP K and -L.
(A) circRNA-RBP complex isolation from differentiated L-AN-5 cells followed by protein identification using mass spectrometry (LC-MS/MS). IP/Input ratios (based on IBAQ values) for selected RBPs (hnRNP L, hnRNP L, hnRNP U, DDX3X and DHX9) pulled down by circZNF827 are shown in left panel. In the right panel IP ratios of selected RBPs pulled down by circZNF827 relative to IP ratios for four other circRNAs (circTULP4, circHDGFRP3, circSLC8A1, and circZNF609) are shown. (B) RIP experiment evaluating interaction between circZNF827 and hnRNP K and -L in differentiated L-AN-5 cells. (C) RIP experiment evaluating interaction between both wildtype circZNF827 (WT) and circZNF827 with a deletion of predicted hnRNPK/L binding sites (hnRNPK/L mut) and hnRNP K and -L in the HEK293 Flp-In T-rex circZNF827 cell lines. Co-immunoprecipitation (co-IP) of both exogenously FLAG-tagged (D) and endogenously (E) expressed hnRNP K, -L and -U in HEK293 Flp-In T-rex cells with and without circZNF827 expression. GAPDH and HuR were used as loading controls in (D) and (E) respectively. (F) Western blot evaluating subcellular localization of hnRNP K in differentiated L-AN-5 cells upon circZNF827 knockdown. LARP1 and hnRNP C1/C2 were used for validation of the purity of the cytoplasmic and nuclear fractions. (G) Co-immunofluorescence (co-IF) of hnRNP K, -L and -U in HEK293 Flp-In T-rex cells upon circZNF827 overexpression. Arrows are pointing to hnRNP K- and hnRNP L-containing nuclear foci. Nuclei were visualized by DAPI staining. The scale bar indicates 10 μm. Irr: Irrelevant dishRNA. C: cytoplasmic fraction, N: nuclear fraction, T: total cell lysate. Data are depicted as mean ± SD (three biological replicates). (B), (E), and (H) One representative western blot is shown. Data shown in (A) and (C) are based on two and one replicates, respectively.
We conclude that both hnRNP K and -L can be found in complex with endogenous or exogenous circZNF827 in both L-AN-5 and HEK293 Flp-in T-Rex cells, likely via high-affinity binding sites in the 3′ part of the circular RNA.
Increasing expression of circZNF827 induces distinct hnRNP K and -L nuclear foci
hnRNP K is a well-documented transcriptional regulator (Moumen et al., 2005; Thompson et al., 2015) that is reported to interact directly with hnRNP L and -U (Havugimana et al., 2012; Kim et al., 2000; Wan et al., 2015) and bind both DNA and RNA (Tomonaga and Levens, 1995). To assess interactions between hnRNP K, -L and -U, and their potential dependence on circZNF827, we performed co-immunoprecipitation experiments using FLAG-tagged hnRNP K, -L, and -U, and subsequently probed for their interaction with endogenous proteins (Figure 5D) in HEK293 Flp-in cells either overexpressing circZNF827 or not. hnRNP K co-immunoprecipitates both hnRNP U and hnRNP L (long isoform), but these interactions remain unaffected by increased expression of circZNF827 (Figure 5D). In accordance with these findings, immunoprecipitation of endogenous hnRNP K and -L proteins in HEK293 Flp-in cells, confirmed that a hnRNP L/hnRNP K complex can be detected although only a small fraction of the total hnRNP K/- L populations co-immunoprecipitate the other (Figure 5E, left). This complex was not affected by overexpression of circZNF827 (Figure 5E, right). Hence, exogenous circZNF827 likely does not regulate bulk hnRNP K/L-complex assembly/disassembly in HEK293 cells per se.
To test whether circZNF827 potentially regulates the normal subcellular distribution of hnRNP K, L-AN-5 cells were fractionated during control or circZNF827 knockdown and lysates subjected to western blotting. We observe a small but significant and reproducible increase in the cytoplasmic population of hnRNP K upon circZNF827 knockdown, suggesting that circZNF827 retains, albeit a very small fraction of the hnRNP K population, in the nucleus (Figure 5F). To address this, we overexpressed circZNF827 and monitored hnRNP K and L localization by immunofluorescence in HEK293 cells. Induction of circZNF827 led to accumulation of hnRNP K and to a lesser extent hnRNP L in multiple distinct nuclear foci that were not detected in control cells (Figure 5G, white arrows). Taken together, our results suggest that while bulk hnRNP K and L complex formation is not affected by circZNF827 levels, overexpression of the circRNA induces specific nuclear localization of hnRNP K and L.
hnRNP K or -L knockdown increases NGFR levels
Could a circZNF827-dependent hnRNP K/L-containing nuclear complex regulate the output from the NGFR gene? If such a complex is instrumental in repressing NGFR, we predict that knockdown of any of these factors would enhance NGFR expression. In support of a role in hnRNP K-mediated regulation of NGFR, it was recently reported that hnRNP K knockdown strongly induces NGFR expression in mouse ES cells (Thompson et al., 2015). To test this in our context, we designed dishRNAs for hnRNP K and -L, transduced L-AN-5 cells and assayed for NGFR expression by qRT-PCR or Western blotting. Both knockdowns increased NGFR expression at both the mRNA and protein levels, similar to the effect of depleting circZNF827 alone (Figure 6A–B and Figure 6—figure supplement 1A–B). However, co-depletion of circZNF827 with any of these factors strongly augmented NGFR expression (four- to fivefold higher than individual knockdowns) (Figure 6A–B), suggesting that their effects are synergistic.
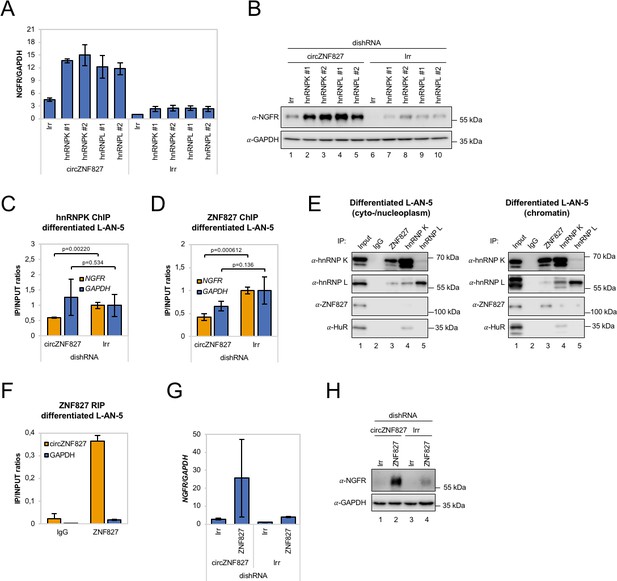
circZNF827 regulates hnRNP K and ZNF827 activity in L-AN-5 cells.
RT-qPCR (A) and western blotting (B) evaluating NGFR expression upon co-knockdown of circZNF827 and either hnRNP K or -L in differentiated L-AN-5 cells. GAPDH was used as loading control for the western blots. #1 and #2: two different dishRNAs targeting the same RBP. ChIP experiment assessing association between the NGFR gene and hnRNP K (C) and ZNF827 (D) upon circZNF827 knockdown in differentiated L-AN-5 cells. (E) Co-immunoprecipitation (co-IP) of ZNF827, hnRNP K, -L and ZNF827 in cyto-/nucleoplasm (left) or chromatin fractions (right; sonicated pellets from cleared lysates) of differentiated L-AN-5 cells. IgG was used as IP control. HuR was used as negative control. (F) RNA-immunoprecipitation of circZNF827 by ZNF827 in differentiated L-AN-5 cells. RT-qPCR (G) and western blotting (H) evaluating NGFR expression upon co-knockdown of circZNF827 and ZNF827 in differentiated L-AN-5 cells. GAPDH was used as loading control for the western blots. Irr: Irrelevant dishRNA. Data are depicted as mean ± SD (three biological replicates). p-Values were determined by a two-tailed Student’s t test.
Given these results, a feasible possibility is that a hnRNP K/L-circZNF827 complex could facilitate transcriptional repression of NGFR by interacting with gene-regulatory regions, consistent with NGFR upregulation upon circZNF827 knockdown. To this end, publicly available ChIP-seq data (ENCODE consortium) in K562 and HepG2 cells demonstrate that hnRNP K indeed interacts with transcription regulatory regions (promoter proximal) of the NGFR gene (Figure 6—figure supplement 1C). To determine the circZNF827-dependence of a hnRNP K-containing complex that docks at the promoter region of NGFR gene in L-AN-5 cells, we next performed hnRNP K ChIP in the presence or absence of circZNF827 and assayed for the NGFR promoter region by qPCR. Our results show that hnRNP K engagement at the NGFR promoter is decreased upon circZNF827 knockdown compared to the GAPDH gene (Figure 6C), which displayed constant transcription rates in our previous BrU pulse labeling assay. We next wondered how this circRNP complex can interact with chromatin. It was recently demonstrated that hnRNP K partakes in a complex with chromatin-bound KRAB-domain zinc finger proteins (KRAB-ZNFs), and that hnRNP K is necessary for recruitment of a transcription inhibitory SETDB1/KAP1 complex, which catalyzes H3K27 trimethylation and heterochromatin formation (Thompson et al., 2015). We therefore hypothesized that ZNF827 protein, which does not harbor a discernible KRAB domain, could interact with either hnRNP K and/or its encoded circRNA and perhaps link this transcription repressive complex to the NGFR promoter. To test this hypothesis, we first conducted a ZNF827 ChIP experiment and probed for an interaction with the NGFR promoter region in the presence or absence of circZNF827. Indeed, our results suggest that ZNF827 interacts with the NGFR promoter and that the signal was significantly diminished upon circZNF827 knockdown (Figure 6D). Interestingly, we observed a similar reduction in signal at the RARA promoter region, which we had previously found to be moderately, yet significantly, upregulated (Figure 6—figure supplement 1D). To address whether ZNF827 and hnRNP K interact, we next performed ZNF827 immunoprecipitation and found it to strongly associate with hnRNP K and to a lesser extent with hnRNP L in nucleoplasmic extracts (Figure 6E, left). Upon sonication of the remainder from the Triton X-100 extracted cleared lysates (chromatin enriched), we observed an even stronger association of hnRNP K with ZNF827, further suggesting that the complex is chromatin bound (Figure 6E, right). When assessing the ability of ZNF827 to interact with circZNF827, we observed a strong enrichment over IgG (~18 fold), and ZNF827 protein co-immunoprecipitated circZNF827 more efficiently than GAPDH mRNA (~19 fold more enriched) (Figure 6F). Finally, we performed ZNF827 knockdown to test whether this would also augment expression of NGFR as observed upon hnRNP K/- L knockdown. Indeed, we observed a strong upregulation of NGFR upon ZNF827 knockdown, an effect that was further augmented in a circZNF827 knockdown background (Figure 6G–H and Figure 6—figure supplement 1E).
Taken together, our results are consistent with a model where circZNF827 represses NGFR transcription (and likely many other genes, including RARA) by bridging a hnRNP K/L-contaning inhibitory complex with their genomic loci, facilitated by the ZNF827 protein.
circZNF827 is part of the chromatin-bound hnRNP-ZNF827 complex
Mechanistically, an important outstanding question is whether circZNF827 nucleates the hnRNP-ZNF827 complex to potentially prepare/activate it for engagement with chromatin, or whether circZNF827 itself, is part of the chromatin-bound complex. To address this question, we first cloned a circZNF827 expression construct, which includes a sequence encoding 2 X MS2 hairpins (Figure 7A) and established stable inducible HEK293 cells (HEK293-circZNF827-MS2). Next, we transfected these cells, HEK293 control cells (Empty) or HEK293-circZNF827-WT (lacking MS2 hairpins) with a plasmid encoding FLAG-tagged MS2 coat protein (FLAG-MS2cp) and subsequently performed anti-FLAG ChIP analysis, to test whether the FLAG-tagged MS2cp becomes crosslinked to the NGFR promoter via circZNF287-2XMS2. The NGFR promoter signal was significantly higher in HEK293 cells harboring the circZNF827-2XMS2 expression cassette, when compared to both control cell lines and background signal on the GAPDH promoter remained constant in all three cell types (Figure 7B). Importantly, RIP experiments demonstrated that both hnRNP K and -L interact with circZNF827-2XMS2 with similar affinities as circZNF827-WT (Figure 7—figure supplement 1). These results demonstrate that circZNF827 engages the NGFR promoter, suggesting that the circular RNA nucleates the hnRNP-ZNF827-containing complex on chromatin to limit NGFR expression (Figure 7C), which in turn contributes to an important balance between neuronal differentiation and self-renewal/proliferation.
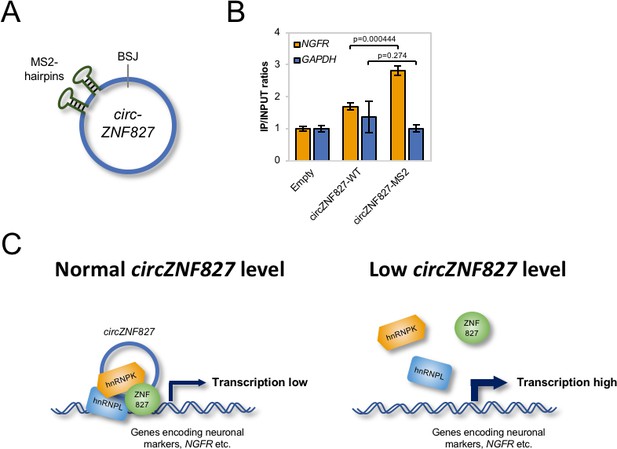
circZNF827 is associated with the NGFR promoter region.
(A) Schematic representation of circZNF827 tagged with two MS2 hairpins. (B) ChIP experiment assessing association between the NGFR gene and circZNF827 in HEK293 Flp-In T-rex cell lines expressing etiher wildtype circZNF827 (circZNF827-WT) or MS2-tagged circZNF827 (circZNF827-MS2). (C) Model illustrating how circZNF827, hnRNP K/- L and ZNF827 regulates target gene expression. Target genes, e.g. NGFR is bound by a transcription repressive complex consisting of circZNF827, hnRNP K, hnRNP L and ZNF827. High levels of circZNF827, induced by neuronal differentiation keeps further differentiation markers in check (left panel), while knockdown of circZNF827 (or hnRNP K/L or ZNF827) allows for higher transcription rates of target neuronal marker genes including NGFR. Data in (C) are depicted as mean ± SD (three biological replicates).
Discussion
Circular RNAs are by now considered as an important class of abundant and conserved RNAs but their functional potential has not been fully elucidated yet. Here, we identified high-confidence circRNA inventories of E14 mESCs, mNPCs and differentiated glutamatergic neurons, and show a generally high degree of conservation among circRNAs previously identified using cell lines and tissues of neuronal origin (Rybak-Wolf et al., 2015). Three different circRNA prediction pipelines, CIRI2 (Gao et al., 2018), find_circ (Memczak et al., 2013) and CIRCexplorer2 (Zhang et al., 2016), displayed marked differences in their predictions, which is in line with our earlier observations (Hansen et al., 2016). This could indicate that many reported circRNAs are false positives, especially when expressed at low levels. A surprisingly large fraction of initially called circRNAs by the three pipelines becomes depleted upon RNase R treatment (between 7.2% and 52.3%), with CIRI2 clearly being the most robustly performing predictor in terms of RNase R resistance. Among 3581 RNase R-resistant circRNAs, only 1449 were called by all three algorithms, suggesting that caution should be taken when predicting circRNAs from RNA-seq data and that including multiple prediction algorithms and/or an RNase R step prior to RNA-seq is beneficial.
Analyzing circRNA expression over the three neuronal developmental stages, we identified 116 differentially expressed circRNAs (>2 fold change). Of 14 tested circRNA candidates, knockdown of circZNF827 in human L-AN-5 cells had a significant and positive impact on the expression of several classical neuronal markers, suggesting that the circRNA normally exerts a negative role in neuronal differentiation. Among 760 genes important to neuronal differentiation and disease, we found that NGFR was most strongly induced, also at the protein level, upon circZNF827 knockdown. NGFR is a member of the TNF superfamily of receptors and relays, along with three paralogous receptor tyrosine kinases (NTRK1, NTRK2 and NTRK3), signals from the four mammalian neurotrophins (Nerve Growth Factor (NGF)), brain-derived neurotrophic factor (BDNF), neurotrophin-3 (NTF3) and neurotrophin 4 (NTF4) [Bothwell, 2016]. The regulation and functional output from the neurotrophins and their receptors, which are interdependent proteins, is very complex and involves a multitude of effector proteins and interaction partners (Bothwell, 2016). NGFR can, depending on expression levels of the other neurotrophin receptors and their ligands, either induce death- or survival signaling to promote neuronal differentiation and control axonal growth or apoptosis (Bothwell, 2016). Whether NGFR upregulation is instrumental and causal for the enhanced expression of NTRK2, NEFL, TUBB3, and MAP2 that we observe in the L-AN-5 neuroblastoma system, remains to be investigated. However, we did observe strongly augmented FOS expression (immediate early gene) upon treatment of L-AN-5 cells with NGF, when circZNF827 was downregulated, which suggests that NTRK1-mediated NGF response becomes enhanced by increased NGFR expression. It is possible that NGFR is induced to increase death-signaling, as a result of skewed and sub-optimal stoichiometry between key neuronal markers/effectors (e.g. NTRK2, NEFL, TUBB3, and MAP2). Such a scenario might be part of a normal surveillance system that monitors a strict and sequential appearance of differentiation factors; however, this awaits further disclosure in more physiologically relevant cell- and animal models.
Mechanistically, several lines of evidence support a model in which circZNF827 plays a direct role in the transcriptional repression of the NGFR and RARA loci, potentially as a scaffolding-RNA for a hnRNP K/-L-ZNF827 containing complex (Figure 7C). NGFR mRNA decay rates remain unchanged upon circZNF827 knockdown, while stready-state levels increase three- to fourfold. Knockdown of circZNF287 resulted in significantly higher BrU incorporation rates in NGFR pre-mRNA and an increased association of PolII at the NGFR promoter. Importantly, hnRNP K and ZNF287 association with the NGFR promoter was decreased upon circZNF287 knockdown. In addition, depletion of either hnRNP K, -L, or ZNF827, which all interact robustly with circZNF827, strongly augmented the transcriptional induction by circZNF827 knockdown. We observed strong focal nuclear condensates containing endogenous hnRNP K and -L proteins in HEK293 Flp-in T-Rex cells stably expressing circZNF827. Although such condensates may be non-physiological entities (phase-separated hnRNPs), induced by high local concentrations of circZNF827, these results suggest that the circRNA could function as a scaffold that nucleates hnRNP K and -L, although not readily visible in the microscope when circZNF827 levels are significantly lower.
It is well established that hnRNP K participates in transcriptional repression. hnRNP K can bridge classical DNA-binding KRAB-ZNF proteins and a KAP1/SETDB1-containing complex, which in turn facilitates heterochromatin formation – also in the NGFR gene of ES cells (Thompson et al., 2015). A similar mechanism was described by Huarte and collegues, where a p53-induced lincRNA-p21 interacts with hnRNPK, which facilitates silencing of several downstream targets (Huarte et al., 2010). Interestingly, transcriptional stimulation, rather than repression, has been reported for intron-containing circRNAs (ElciRNAs), via recruitment of U1 snRNP to the transcriptional complex on their parental genes, which by definition requires exon-intron boundaries (Li et al., 2015). CircZNF827 is a regular exonic circRNA, without intronic sequences (data not shown), perhaps explaining why it represses transcription as opposed to ElciRNAs. Another circRNA, circSlc45a4, was recently also shown to negatively regulate neuronal differentiation, both in cell cultures and in developing mice, where its knockdown dysregulates the balance between specialized cortex neurons (Suenkel et al., 2020). Taken together, our ChIP, RIP and co-IP results all suggest that the protein-product originating from the circZNF827-encoding pre-mRNA host, ZNF827, links hnRNP K/- L-circZNF827 to chromatin, via its DNA-binding capacity, similar to known roles of KRAB-ZNF proteins. If so, circZNF827 co-regulates target genes along with its precursor-encoded protein, which argues for co-evolutionary selection pressure to preserve both circRNA-generating and protein-coding sequences. circMBNL1 has also been shown to regulate the activity of its cognate protein product (Ashwal-Fluss et al., 2014), suggesting that this phenomenon could be a common theme that awaits further investigation.
Materials and methods
Sequences of all primers and probes used in the study are specified in Supplementary file 2c-e. Antibodies are described in Supplementary file 2f.
Vector construction
Request a detailed protocolTo create plasmids for expression of dishRNAs, sense and antisense oligonucleotides were annealed and cloned into BglII/XhoI-digested pFRT/U6, resulting in vectors designated pFRT/U6-dishRNA (Kaadt et al., 2019). Subsequently, the U6-dishRNA expression cassettes were PCR-amplified from pFRT/U6-dishRNA vectors and inserted into ClaI/BsiWI-digested pCCL/PGK-eGFP-MCS (Kaadt et al., 2019). The resulting lentiviral transfer vectors were designated pCCL/U6-dishRNA-PGK-eGFP-MCS. As a negative control, an irrelevant dishRNA based on a previously described irrelevant shRNA (Jakobsen et al., 2009), which does not match any known sequence in the human genome was used throughout the study.
To generate plasmids for in vitro transcription of circRNAs, the exons encoding circTULP4, circZNF827, circHDGFRP3, circZNF609, and circSLC8A1 were PCR-amplified from cDNA prepared from RNA isolated from L-AN-5 cells. PCR-amplicons encoding circTULP4, circZNF827, circHDGFRP3, and circSLC8A1 were inserted in BamHI/NotI-digested pcDNA3/PL whereas PCR-amplicons encoding circZNF609 were inserted in HindIII/NotI-digested pcDNA3/PL. The resulting plasmids were designated pcDNA3/circRNA. The plasmid for expression of the CTC lincRNA was constructed as previously described in Seitz et al., 2017.
For exogenous expression of circZNF827, the exons encoding circZNF827 and circZNF827-hnRNPK/L mut were PCR-amplified from cDNA prepared from RNA isolated from L-AN-5 cells and inserted into PacI/SacII-digested pcDNA3.1(+)-Laccase2-MCS-exon-vector (Kramer et al., 2015). Subsequently, the Laccase-circZNF827 and Laccase2-circZNF827-hnRNPK/L mut expression cassettes was inserted into HindIII/NotI- or BamHI/XhoI-digested pcDNA5_FRT/TO resulting in vectors designated pcDNA5_FRT/TO-Laccase2-circZNF827 and -circZNF827-hnRNPK/L mut. pcDNA5-Laccase-circZNF827-2XMS2 was created by subcloning a 2xMS2 hairpin sequence (annealed phosphorylated DNA oligos) into pcDNA3.1(+)-Laccase2-circZNF827 using the unique BspE1 site, Supplementary file 2c. Positive clones were verified by sequencing and a HindIII-NotI fragment from this vector was used to insert into the HindIII-NotI sites of pcDNA5-FRT-TO. This construct was used to create stable inducible HEK293-Laccase2-circZNF827-2XMS2 cells.
pcDNA5-FLAG-MS2-coat-Twin-Streptag was created by inserting the FLAG-MS2 coat protein open-reading frame (HindIII fragment) from pNMS2-FLAG (Clement et al., 2011) into pcDNA5-Twin-Streptag. pcDNA5-Twin-Streptag was made by inserting a PCR product (containing a Twin-Streptag followed by a TEV protease site) using a pDSG-IBA-Twin-Strep-Tag vector as template (IBA-lifesciences) into the HindIII-ApaI site of pcDNA5-FRT-TO (Invitrogen).
To create plasmids for expression of FLAG-tagged RNA binding proteins (RBPs), the coding sequences of RBPs hnRNPK, hnRNPL, hnRNPU, DDX3X, and DHX9 were PCR-amplified from cDNA prepared from RNA isolated from L-AN-5 cells whereas eGFP was sub-cloned from pNEGFP (HindIII/HindIII) and inserted into either KpnI/NotI-, BamHI/NotI- or HindIII-digested pcDNA5_FRT/TO-FLAG. The resulting plasmids were designated pcDNA5_FRT/TO-FLAG-RBP. All plasmids were verified by Sanger sequencing.
Cell culturing
Request a detailed protocolAll used cell lines have been periodically tested for mycoplasma contamination (PCR-based test) with negative results. L-AN-5 cells (obtained from Childrens Oncology Group - COG Cell Line and Xenograft Repository (www.cogcell.org)) were maintained in RPMI whereas SH-SY5Y (obtained from ATCC; ATCC CRL-2266) and HEK293S-Flp-In T-Rex (purchased from Invitrogen) cells were maintained in DMEM medium (Gibco, Dublin, Ireland, 32430100). For all three cell lines, the cell culture medium was supplemented with 10% fetal bovine serum (Gibco, 10082139) and 1% penicillin/streptomycin (Gibco, 15140122). P19 cells (obtained from ATCC; ATCC CRL-1825) were maintained in MEMα supplemented with 7.5% newborn calf serum (Gibco, 26010074), 2.5% fetal bovine serum (Gibco, 10082139), and 1% penicillin/streptomycin (Gibco, 15140122). HEK293 Flp-In T-Rex cells were maintained in DMEM supplemented with 10% tetracyclin-free fetal bovine serum (Gibco, 10082139) and 1% penicillin/streptomycin (Gibco, 15140122). All cells were cultured at 37°C in 5% (v/v) CO2.
For neuronal differentiation of the neuroblastoma cell lines L-AN-5, SH-SY5Y, and P19 10 μM retinoic acid (RA) (Sigma-Aldrich, St. Louis, Missouri, United States) was added to the cell culture medium for 4 days.
The cell line with stable expression of circZNF827 was generated as previously described (Hollensen et al., 2018). Briefly, HEK293 Flp-In T-Rex cells were co-transfected with pcDNA5-FRT/TO-laccase2-circZNF827, -circZNF827-MS2, or -circZNF827-hnRNPK/L mut and a plasmid for expression of the Flp recombinase (pOG44). Cell culture medium supplemented with 100 ng/ml Hygromycin (Thermo Scientific, Waltham, Massachusetts, United States) and 10 ng/ml Basticidin S (Thermo Scientific) was used for selection of positive clones. The resulting cell line was designated HEK293 Flp-In T-Rex-circZNF827, circZNF827-MS2, and -circZNF827-hnRNPK/L mut. Tetracycline (Tet) concentrations used for titration of circRNA induction (Northern blot) were 5 ng/ml, 25 ng/ml, 100 ng/ml, and 250 ng/ml, respectively. 25 ng/ml Tet was used for RIP experiments and 250 ng/ml Tet for hnRNP K/L immunofluorescence assays.
mESC culture and differentiation
Request a detailed protocolE14 mESCs (ES-E14TGs2a; obtained from ATCC; ATCC CRL-1821) were grown on 0.1% gelatin-coated plates in 2i medium (Ying et al., 2008) containing: DMEM/F12 (Gibco, 31331) and Neurobasal (Gibco, 12348) 1:1, N2 supplement (Gibco, 17502048), B27 supplement (Gibco, 17504044), 1X glutaMax (Gibco, 35050061), 1X penicilin/streptomycin (Gibco, 15140122), 1 mM sodium pyruvate (Gibco, 11360070), 50 nM 2-mercaptoethanol (Gibco, 31350010), nonessential amino acids (Gibco, 11140076), LIF, 3 μM GSK3 inhibitor (CHIR-99021) and 1 μM MEK inhibitor (PD0325901). They were differentiated into neurons as previously described (Bibel et al., 2007) with some modifications. 4 million cells were differentiated into embryoid bodies in suspension in petri dishes for bacterial culture in 15 ml medium containing the same as before, but with 10% FBS and without LIF or GSK3 and MEK inhibitors. Every second day, the medium was changed and the embryoid bodies transferred to fresh petri dishes. On days 4 and 6, 5 μM ATRA (Sigma-Aldrich, R2625) was added to the medium. On day 8 of differentiation, the embryoid bodies were disgregated with 5% trypsin (Gibco, 15400054) and the cells plated in poly-DL-ornithine (Sigma-Aldrich, P8638) and laminin (Sigma-Aldrich, L2020) coated plates in N2 medium, containing DMEM/F12 and neurobasal 1:1, N2 supplement, sodium pyruvate, glutaMax, 15 nM 2-mercaptoethanol, and 50 μg/ml BSA. The medium was changed after 2 hr and after 24 hr. 48 hr after plating the neuronal precursors, the medium was changed to complete medium, containing B27 supplement, in addition to the N2 medium. Neurons were harvested 2 and 8 days after plating. Our E14 cell line has periodically been tested for mycoplasma contamination (PCR-based test) with negative results.
RNA sequencing and circRNA prediction
Request a detailed protocol20 µg RNA from each sample was depleted of rRNA using a Ribo-Zero rRNA Magnetic Kit (Epicentre, St Louis, Missouri, United States) including the optional RiboGuard RNase inhibitor according to the manufacturer’s protocol. The concentration was normalized so that each sample contained the same amount of RNA. To 1/3 of the sample 1/10 of the recommended amount of spike-in (ERCC RNA spike-in mix, Ambion) was added, ethanol precipitated, and resuspended in ‘Elute, fragment, finish mix’ (Illumina, San Diego, California, United States). The remaining 2/3 of the sample was ethanol precipitated and resuspended in 15 µl nuclease free water. The sample was heated to 70 °C for 1 min and incubated on ice for 2 min. 5 µl RNase R mixture (Epicentre) was added to the sample before incubation at 37°C for 30 min. RNase R was removed by phenol/chloroform extraction. The RNA was resuspended in ‘Elute, fragment, finish mix’ (Illumina). Sequencing libraries were prepared using Truseq stranded RNA LT kit (Illumina) from both Ribo-Zero and Ribo-Zero/RNase R samples, by fragmentation, 1st and 2nd strand cDNA synthesis, 3′-end adenylation, ligation of adaptors, and enrichment of DNA fragments using the manufacturer’s protocol. The quality of the library was validated using an Agilent Bioanalyzer 1000 (Agilent Technologies, Santa Clara, California, United States). The samples were sequenced using the Illumina HiSeq 2500 platform with 100 bp paired-end reads (AROS Applied Biotechnology, Aarhus, Denmark).
Reads were mapped onto the mm10 genome, and circRNAs were detected and quantified using find_circ (Memczak et al., 2013), CIRCexplorer2 (Zhang et al., 2016) (v2.3.3), and ciri2 (Gao et al., 2018) (v2.0.6) using default settings except for find_circ, where a stringent mapq threshold of 40 was used for both adaptor sequences as proposed previously (Hansen, 2018). The prediction-output from all pipelines was merged and intersected, and only circRNAs detected by all three pipelines and with three-fold enrichment of backsplice-spanning reads in the RNAseR-treated samples were defined as bona fide. Expression, based on untreated samples quantified ciri2, was RPM normalized and the top100 expressed bona fide circRNAs across all samples were subjected to kmean clustering using five centers based on within-clusters sum of squared. Annotated genes (UCSC annotation) with at least one splice site in common with circRNAs were denoted as host genes, and based on host-gene annotation, exon numbers and flanking intron lengths were extracted.
The circ-to-linear ratios were based on the backsplice junction spanning reads and the mean of upstream and downstream linear spliced reads as quantified by find_circ.
To compare with human expression profiles, the top100 expressed circRNAs were converted from mm10 to hg19 coordinates using liftOver (UCSC), and only fully matched loci were considered homologous. Data is available at the NCBI Gene Expression Omnibus: GSE157788.
RNAseq from Rybak-Wolf et al (GSE65926 Rybak-Wolf et al., 2015) was solely analyzed with find_circ using stringent settings as described above.
Lentiviral production
Request a detailed protocolThird-generation lentiviral vectors were produced in HEK293T cells as previously described (Hollensen et al., 2017). One day before transfection, cells were seeded in 10 cm dishes at a density of 4 × 106 cells/dish. Transfections were carried out with 3.75 μg pMD.2G, 3 μg pRSV-Rev, 13 μg pMDLg/pRRE and 13 μg lentiviral transfer vector using a standard calcium phosphate or polyethylenimine transfection protocol. Medium was changed to RPMI medium one day after transfection. Two days after transfection, viral supernatants were harvested and filtered through 0.45 μm filters (Sartorius, Göttingen, Germany). All lentiviral preparations were made in at least triplicates and pooled before determination of viral titers.
To determine viral titers of lentiviral preparations, flow cytometric measurements of eGFP expression were used as previously described (Hollensen et al., 2017). One day prior to transduction, L-AN-5 cells were seeded at a density of 5 × 105 cells/well in 12-well plates. For all lentiviral preparations, transductions with 102- and 103-fold dilutions of virus-containing supernatants were carried out. Both viral supernatants and growth medium were supplemented with 4 μg/ml polybrene. One day after transduction, medium was changed. Five days after transduction, cells were harvested and fixated in 4% paraformaldehyde (Sigma-Aldrich). eGFP expression levels were analyzed on a CytoFLEX flow cytometer (Beckman Coulter, Brea, California, United States). Lentiviral titers were calculated based on samples with between 1% and 20% eGFP-positive cells using the formula: titer (TU/ml)=F·Cn·DF/V, where F represents the frequency of eGFP-positive cells, Cn the total number of target cells counted the day the transductions were carried out, DF the dilution factor of the virus and V the volume of transducing inoculum.
circRNA knockdown and differentiation of L-AN-5 cells
Request a detailed protocolOne day prior to transduction with lentiviral vectors encoding circRNA-specific dishRNAs, L-AN-5 cells were seeded at a density of 6.6 × 106 cells/dish in 10 cm dishes, 2.2 × 106 cells/dish in 6 cm dishes, or 0.8 × 106 cells/well in 6-well plates. Transductions were carried out using equal MOIs calculated based on titers determined by flow cytometry. Both viral supernatants and growth medium were supplemented with 4 μg/ml polybrene. One day after transduction, medium was changed. Two days after transduction, differentiation was initiated by addition of 10 μM RA (Sigma-Aldrich) to the cell culture medium. The L-AN-5 cells were differentiated for 4 days.
NGF stimulation
Request a detailed protocolLentiviral transduction and RA-mediated differentiation of L-AN-5 cells were carried out as described in the section ‘circRNA knockdown and differentiation of L-AN-5 cells’. After 4 days of differentiation, the L-AN-5 cells were stimulated with NGF (200 ng/ml) (Thermo Scientific) for 30 min and subsequently harvested for RNA purification.
mRNA decay assay
Request a detailed protocolLentiviral transduction and RA-mediated differentiation of L-AN-5 cells were carried out as described in the section ‘circRNA knockdown and differentiation of L-AN-5 cells’. The L-AN-5 cells were cultured in 6 cm dishes containing 6 ml cell culture medium supplemented with 10 μM RA. 4 ml cell culture medium was aspirated from each 6 cm dish and pooled from cells transduced with the same dishRNA. For one dish per dishRNA, the residual medium was aspirated and 3.5 ml of the collected medium was added. For the remaining dishes, the residual medium was aspirated and 3.5 ml of the collected medium supplemented with 2 mM BrU (ThermoFisher) was added. 1 hr after addition of BrU to the cell culture medium, the cells were washed three times in cell culture medium. 50 min after removal of the BrU-containing cell culture medium the first samples including the samples not treated with BrU were harvested. Subsequently, samples were harvested after 3, 6, and 9 hr. Total RNA was purified using 1 ml TRI Reagent (Sigma-Aldrich) according to manufacturer’s protocol. circRNA knockdown and differentiation of L-AN-5 cells were verified by RT-qPCR using total RNA as described in the section ‘Quantitative PCR’. BrU-labeled RNA was immunoprecipitated as described elsewhere (Meola et al., 2016). Briefly, BrU antibodies were conjugated to magnetic beads. 15 μl Dynabeads M-280 Sheep Anti-Mouse IgG (Invitrogen, Carlsbad, California, United States) per sample were washed twice in 1x BrU-IP buffer (20 mM Tris-HCl (pH 7.5)), 250 mM NaCl, 0.5 μg/μl BSA, 20 U/ml RiboLock (Fermentas, Waltham, Massachusetts, United States) and resuspended in 1 ml 1x BrU-IP buffer with heparin (20 mM Tris-HCl (pH 7.5), 250 mM NaCl, 1 mg/ml heparin). After 30 min of incubation at room temperature on a rotator, the beads were washed in 1x BrU-IP buffer. Subsequently, the beads were resuspended in 1 ml 1x BrU-IP buffer supplemented with 0.9 μl mouse BrdU antibody (BD Biosciences, San Jose, California, United States, clone 3D4) per sample and incubated for 1 hr at room temperature on a rotator. The beads were washed three times in 1x BrU-IP buffer and resuspended in 50 μl 1x BrU-IP buffer supplemented with 1 mM 5-BrU per sample. After 30 min of incubation at room temperature on a rotator the beads were washed three times in 1x BrU-IP buffer and resuspended in 50 μl 1x BrU-IP buffer per sample. 25 μg of total RNA was diluted to 200 μl and incubated at 80°C for 2 min. 200 μl 2x BrU-IP buffer with BSA and RiboLock (20 mM Tris-HCl (pH 7.5)), 250 mM NaCl, 1 μg/μl BSA, 80 U/ml RiboLock (Thermo Scientific) and 50 μl beads conjugated with BrdU antibodies were added to the RNA samples. After 1 hr of incubation at room temperature on a rotator, the beads were washed four times in 1x BrU-IP buffer. For elution of immunoprecipitated RNA, the beads were resuspended in 200 μl 0.1% SDS. RNA was purified by phenol/chloroform extraction, ethanol precipitation and the RNA pellets were resuspended in 10 μl nuclease free water. 2 μl of immunoprecipitated RNA was used for quantification of mRNA expression levels by RT-qPCR as described in the section ‘Quantitative PCR’ except that DNase treatment was omitted and 1 μg yeast RNA (Roche, Basel, Switzerland) was added in the cDNA reaction.
BrU-labeling and immunoprecipitation of newly synthesized RNA
Request a detailed protocolThe BrU-labeling and immunoprecipitation of newly labeled RNA were carried out as for the mRNA decay assay except that the cells were harvested 45 min after addition of BrU to the cell culture medium. Furthermore, after binding of the RNA to the beads, the beads were washed once in 1x BrU-IP buffer, twice in 1x BrU-IP buffer supplemented with 0.01% Triton X-100 and twice in 1x BrU-IP buffer.
Subcellular fractionation of nuclear and cytoplasmic RNA
Request a detailed protocolSubcellular fractionation of nuclear and cytoplasmic RNA was carried out as previously described (Hollensen et al., 2018). Briefly, cells were washed in PBS, then 800 μl PBS was added and the cells were scraped off. 100 μl of the cell solution was centrifuged at 12,000 rpm for 10 s at 4°C. Cell pellets were used for purification of total RNA using 1 ml of TRI Reagent (Sigma-Aldrich) according to manufacturer’s protocol. The remaining 700 μl of the cell solution was used for subcellular fractionation of nuclear and cytoplasmic RNA. After centrifugation at 12,000 rpm for 10 s at 4°C 300 μl lysis buffer (20 mM Tris-HCl (pH 7.5), 140 mM NaCl, 1 mM EDTA, 0.5% Igepal-630 (Nonidet P-40)) were added to the cell pellets, which were then incubated on ice for 2 min and centrifugated at 1000 g for 4 min at 4°C. Cytoplasmic RNA was purified from the supernatants using 1 ml TRI Reagent (Sigma-Aldrich) according to the manufacturer’s protocol. Pellets were washed twice in 500 μl lysis buffer, subjected to a single 5 s pulse of sonication at the lowest settings (Branson Sonifier 250) and nuclear RNA was purified using 1 ml TRI Reagent (Sigma-Aldrich) according to the manufacturer’s protocol.
Subcellular fractionation of nuclear and cytoplasmic protein
Request a detailed protocolCells were washed in PBS, then 800 μl PBS were added and the cells were scraped off. 80 μl of the cell solution was centrifuged at 500 g for 5 min and 200 μl lysis buffer (1x TBS, 0.5% Igepal-630 (Nonidet P-40)) were added to the cell pellets for isolation of total protein. The remaining 720 μl of the cell solution was used for subcellular fractionation of nuclear and cytoplasmic protein. After centrifugation at 12,000 rpm for 10 s at 4°C cell 300 μl lysis buffer were added and the cell pellets, which were incubated on ice for 2 min and centrifugated at 1000 g for 4 min at 4°C. The supernatants (cytoplasmic fractions) were transferred to new tubes. Pellets (nuclear fractions) were washed twice in 500 μl lysis buffer and once in 500 μl 1x TBS and resuspended in 200 μl lysis buffer. All samples were subjected to two 5 s pulses of sonication at the lowest settings (Branson Sonifier 250) followed by centrifugation at 4000 g for 25 min at 4°C. Supernatants were transferred to new tubes containing 87% glycerol (final concentration of 10%) and concentrations were adjusted using Bio-Rad protein assay (Bio-Rad, Hercules, California, United States).
Quantitative PCR
Request a detailed protocolRNA was purified using TRI reagent (Thermo Scientific) according the to manufacturer’s protocol. RNA samples were treated with DNase I (Thermo Scientific) according to the manufacturer’s protocol. First-strand cDNA synthesis was carried out using the Maxima First Strand cDNA synthesis Kit for qPCR (Thermo Scientific) according to the manufacturer’s protocol. qPCR reactions were prepared using gene-specific primers (Supplementary file 2d) and Platinum SYBR Green qPCR Supermix-UDG (Thermo Scientific) according to the manufacturer’s protocol. An AriaMx Real-time PCR System (Agilent Technologies) was used for quantification of RNA levels and the X0 method was used for calculations of relative RNA levels (Thomsen et al., 2010) normalized to either GAPDH or beta-actin (ACTB) mRNA as indicated.
NanoString
Request a detailed protocolGene expression analysis of 770 neuropathology-related genes were analyzed using the nCounter Human Neuropathology Panel (NanoString Technologies, Seattle, Washington, United States) and the nCounter SPRINT Profiler (NanoString Technologies) according to manufacturer’s protocol. Data analysis was carried out in the nSolver 4.0 software (NanoString Technologies) using the default settings in the nCounter Advanced Analysis Software (NanoString Technologies).
Cell cycle assay
Request a detailed protocolLentiviral transduction and RA-mediated differentiation of L-AN-5 cells were carried out as described in the section ‘circRNA knockdown and differentiation of L-AN-5 cells’. Labeling of newly synthesized DNA was carried out using Click-iT Plus EdU Alexa Flour 647 Flow Cytometry Assay Kit (Thermo Scientific) according to manufacturer’s protocol. Notably, the cell culture medium of L-AN-5 cells cultured in six-well plates was supplemented with 10 μM EdU for 1.5 hr. To stain total DNA, cells with already detected EdU were resuspended in 400 μl 1x Click-iT saponin-based permeabilization and wash reagent from the Click-iT Plus EdU Alexa Flour 647 Flow Cytometry Assay Kit (Thermo Scientific). Subsequently, RNase A was added to a final concentration of 0.2 mg/ml. After 5 min of incubation at room temperature, propidium iodide was added to a final concentration of 5 μg/ml and the cells were incubated for 30 min at room temperature. Incorporated EdU and total DNA levels were analyzed on a BD LSRFortessa flow cytometer (BD Biosciences). Data analysis was carried out in the FLOWJO software (BD Biosciences). The gating strategy is shown in Figure 2—figure supplement 3A.
Western blotting
Request a detailed protocolCells were scraped off, pelleted and lysed for 15 min on ice in RSB100 (10 mM Tris-HCl (pH 7.4), 100 mM NaCl, 2.5 mM MgCl2) supplemented with 0.5% Triton X-100 and 1 pill Complete protease inhibitor cocktail (Roche). The cell lysates were subjected to two 5 s pulses of sonication at the lowest settings (Branson Sonifier 250) followed by centrifugation at 4000 g for 15 min at 4°C. Glycerol was added to the supernatants (final concentration: 10%) and protein concentrations were adjusted using Bio-Rad protein assay (Bio-Rad). The protein samples were diluted in 6x loading buffer (9.8% glycerol, 12% SDS, 375 mM Tris-HCl (pH 6.8), 0.03% bromophenol blue, 10% β-mercaptoethanol), heated at 95°C for 3 min and seprated on a Novex WedgeWell 4–12% Tris-Glycine Gel (Invitrogen). Proteins were transferred to an PVDF Transfer Membrane (Thermo Scientific) using standard procedures. The membranes were blocked in 5% skimmed milk powder in PBS for 1 hr at room temperature. The membranes were incubated at 4°C overnight with primary antibodies diluted as indicated in Supplementary file 2f in 5% skimmed milk powder in PBS. After three times wash, the membranes were incubated with goat polyclonal HRP-conjungated secondary antibodies (Dako, Glostrup, Denmark) diluted 1:20,000 in 5% skimmed milk powder in PBS. After 1 hr of incubation at room temperature, the membranes were washed three times and the bound antibodies were detected using the SuperSignal West Femto maximum sensitivity substrate (Thermo Scientific) according to the manufacturer’s protocol and using the LI-COR Odyssey system (LI-COR Biosciences, Lincoln, Nebraska, United States).
In vitro transcription
Request a detailed protocolAs DNA templates for in vitro transcription, pcDNA3/circRNA vectors encoding the full-length exonic sequences of five human circRNAs were used. Biotinylated RNAs were produced from 0.5 µg linearized, and phenol/chloroform extracted template using the MEGAscript T7 Transcription Kit (Ambion, Austin, Texas, United States), according to the manufacturer’s protocol with addition of 0.75 mM Biotin-14-CTP (Invitrogen) to the transcription reaction. In controls, nuclease free water was added instead of Biotin-14-CTP. The transcribed RNA was purified by phenol/chloroform extraction and dissolved in nuclease free water.
Streptavidin-biotin pull-down
Request a detailed protocolFor each pull-down, 125 µL (bead volume) Pierce Streptavidin magnetic beads (Thermo Scientific) pre-washed in NET-2 buffer (50 mM Tris-HCl pH 7.5, 150 mM NaCl, 0.1% Triton X-100) were incubated with 30 µg in vitro synthesized circRNAs or 30 µg control RNA in 500 µL NET-2 buffer for 1 hr. 4°C mixing end-over-end. The conjugated beads were washed once in NET-2 buffer and incubated with 1.5 mL cell lysate prepared as follows: For each pull down, one 90% confluent 150 mm plate of differentiated L-AN-5 cells was washed in 10 mL ice cold PBS, and subjected to cell lysis in 1.5 mL hypotonic gentle lysis buffer (10 mM Tris-HCl pH 7.5, 10 mM NaCl, 2 mM EDTA pH 8.0, 0.1% Triton X-100) supplemented with Complete protease inhibitor cocktail (Roche, 1 pastel per 10 mL lysis buffer) for 5 min. on ice. Cells were collected by scraping and re-suspension, then supplemented with NaCl to 150 mM final concentration and incubated on ice for 5 min. Cleared cell lysate was obtained by centrifugation at 14,000 rpm, 4°C, 10 min. and supplemented with 10 µL Ribolock RNase Inhibitor (40 U/µL, Thermo Scientific) per 10 mL lysis before incubation with circRNA-coupled streptavidin beads for 1.5 hr, 4°C mixing end-over-end. From the cleared lysate 1% was mixed 1:1 with 2xSDS-load buffer (20% glycerol, 4% SDS, 100 mM Tris-HCL pH 6.8, 0.05% Bromophenol blue/Xylene cyanol and 10% β-mercaptoethanol) and kept as input sample. Following capture of proteins, beads were washed four times in NET-2 buffer and bound proteins were eluted in 40 µL preheated 2x SDS-load buffer by boiling at 90°C for 5 min. Eluates were subjected to SDS-PAGE electrophoresis and run either completely through and stained with SilverQuest Silver staining kit (Life Technologies, Carlsbad, California, United States) according to the manufacturer’s protocol, or only 1.5 cm into the gel for subsequent staining with GelCode Blue Stain Reagent (Thermo Scientific) according to the manufacturer’s protocol and excision of the bands for mass spectrometry application (see below).
Protein analysis by nano-LC-MS/MS
Request a detailed protocolInteracting proteins were identified and quantified according to previously described methods (Britze et al., 2014). Briefly, each gel lane was cut into 1 × 1 mm pieces and cysteine residues were blocked by reduction and alkylation using tris(2-carboxyethyl)phosphine and iodoacetamide, respectively. In-gel digestion was performed using trypsin and resulting peptides were extracted from gel pieces using acetonitrile and trifluoroacetic acid and finally purified on PepClean C-18 Spin columns (Thermo Scientific). Liquid chromatography tandem mass spectrometry (LC-MS/MS) was performed on an EASY nanoLC coupled to a Q Exactive Plus Hybrid Quadrupole-Orbitrap Mass Spectrometer (Thermo Scientific). Peptide samples were separated on a C-18 reverse phase column (EASY-Spray PepMap from Thermo Scientific with 25 cm length, 75 μm inner diameter, and 2 μm particle size) and eluted by a 90 min linear gradient of acetonitrile (4–40%) containing 0.1% formic acid. The MS was operated in data dependent mode, automatically switching between MS and MS2 acquisition, with mass resolution of 70,000 and 17,500, respectively. Up to 10 most intense ions were fragmented per every full MS scan, by higher energy collisional dissociation. Dynamic exclusion of 10 s was applied and ions with single charge or unassigned charge states were excluded from fragmentation.
MaxQuant software version 1.5.2.8 was applied for protein identification and label-free quantification by means of peptide peak areas (Cox and Mann, 2008). MS raw files were searched against a database consisting of 20,197 Homo sapiens sequences downloaded from Uniprot.org, August 2015. Carbamidomethylation of cysteines was set as a fixed modification, whereas methionine oxidation and protein N-terminal acetylation were set as dynamic modifications. The false discovery rate (FDR) was assessed by searching against a reverse decoy database, and FDR thresholds of protein and peptide identification were both set to 0.01.
Immunofluorescence
Request a detailed protocolFor indirect immunofluorescence experiments, 1 × 105 HEK293 Flp-In T-Rex or circZNF827_HEK293 Flp-In T-Rex cells were grown directly on poly-L-lysine coated coverslips in 12-well plates. Transcription of circRNA transgene was induced by addition of 10–250 ng/ml tetracycline and the induction profile was tested by Northern blotting in a parallel experiment. 24 hr later cells were fixed in 4% paraformaldehyde for 15 min, and permeabilized and blocked with PBS/1% goat serum (or horse serum)/0.5% Triton X-100 for 20 min. Cells were then incubated for 1–16 hr with mouse anti-hnRNPK (Abcam, Cambridge, United Kingdom), Rabbit anti-hnRNPU (Santa Cruz Biotechnologies, Dallas, Texas, United States) or mouse anti-hnRNPL (Abcam). Antibodies were used at 1:1000 dilutions. Following removal of the primary antibody, cells were incubated for 1 hr with 4 μg/mL secondary anti-IgG antibodies labeled with Alexa-594 and Alexa-488 (Molecular Probes, Eugene, Oregon, United States).
RNA immunoprecipitation and co-immunoprecipitation of proteins
Request a detailed protocolFor RNA immunoprecipitation (IP) and co-immunoprecipitation (co-IP) of proteins L-AN-5 cells were seeded at a density of 6.6 × 106 cells/dish in 10 cm dishes and differentiated as described in the section ‘Cell culturing’. HEK293 Flp-In T-Rex cells and HEK293 Flp-In T-Rex circZNF827 cells were seeded a density of 6.6 × 106 cells/dish in 10 cm dishes. HEK293 Flp-In T-Rex and HEK293 Flp-In T-Rex circZNF827 cells were transfected with 5 μg pcDNA5/FRT-TO-FLAG-RBP and 25 μl polyethylenimine (PEI) (1 μg/μl) according to a standard PEI transfection protocol. 6 hr after transfection, RBP and circRNA expression were induced by addition of 100 ng/ml tetracycline to the cell culture medium. For IP of endogenously expressed proteins, antibodies were conjugated to Protein G dynabeads (Thermo Scientific) prior to harvest of the cells. 25 μl beads per sample were washed three times in 1 ml NET-2 buffer (50 mM Tris-HCl pH 7.5, 100 mM NaCl, 0.1% Triton-X100). Subsequently, the beads were resuspended in 800 μl NET-2 buffer per sample and added 10 μl hnRNP K, hnRNP L, or IgG antibody per sample. After conjugation for 120 min at 4°C on a rotator, the beads were washed twice in NET-2 buffer and resuspended in 50 μl NET-2 buffer per sample. For IP of FLAG-tagged proteins, 50 μl anti-FLAG-M2 agarose slurry was washed twice in 1.5 ml NET-2 buffer and resuspended in 50 μl NET-2 buffer per sample. The cells were lysed after a single wash in PBS by addition of 1 ml ice-cold hypotonic gentle lysis buffer (10 mM Tris-HCl pH 7.5, 10 mM NaCl, 1 mM EDTA, 0.25% Triton-X100, and one pill Complete protease inhibitor cocktail (Roche) per 10 ml), scraped off, and transferred to an Eppendorf tube. After incubation for 5 min on ice, 35 μl 4 M NaCl (final 150 mM) was added and the samples were incubated for 2 min on ice. The lysates were subjected to a single 5 s pulse of sonication at the lowest settings (Branson Sonifier 250) and centrifuged at 13,000 rpm for 15 min at 4°C. For input protein and RNA controls, 50 μl and 100 μl of the lysate were resuspended in 50 μl 2xSDS-load buffer (20% glycerol, 4% SDS, 100 mM Tris-HCL pH 6.8, 0.05% Bromophenol blue/Xylene cyanol and 10% β-mercaptoethanol) and 1 ml TRI Reagent (Sigma-Aldrich), respectively. The input protein control samples were incubated for 3 min at 80–90°C before storage at −20°C. The remainder of the supernatants was transferred to tubes containing 50 μl bead slurry and nutated at 4°C for 2 hr. Subsequently, the beads were washed seven times in 1.5 ml ice-cold NET-2 (5 min per wash) and protein was eluted form one third of the beads by addition of 100 μl 2xSDS-load buffer followed by incubation for 3 min at 80–90°C, whereas RNA was eluted from two thirds of the beads by addition of 1 ml TRI Reagent (Sigma-Aldrich).
Northern blotting
Request a detailed protocolNorthern blots were carried out as previously described in Damgaard and Lykke-Andersen, 2011. Briefly, 10 μg RNA was separated in a 1.2% formaldehyde-agarose gel. Subsequently, the RNA was transferred to a Hybond membrane (GE Healthcare, Chicago, Illinoise, United States). The membrane was hybridized with circZNF827- or ACTB-specific [32P]-end-labeled oligonucleotides (sequences are specified in Supplementary file 2e) overnight and subsequently exposed on phosphorimager screens and visualized on a Typhoon FLA 9500 (GE Healthcare).
ChIP
Request a detailed protocolLentiviral transduction and RA-mediated differentiation of L-AN-5 cells were carried out as described in the section ‘circRNA knockdown and differentiation of L-AN-5 cells’. HEK293 Flp-In T-Rex, HEK293 Flp-In T-Rex circZNF827, and HEK293 Flp-In T-Rex circZNF827-MS2 cells were seeded at a density of 5 × 106 cells/dish in 10 cm dishes. Transfections were carried out with 5 μg pcDNA5-FLAG-MS2-coat-Twin-Streptag encoding the MS2 coat protein and 25 μl PEI (1 μg/μl) according to a standard PEI transfection protocol. 5 hr after transfection, MS2 coat protein and circRNA expression were induced by addition of 100 ng/ml tetracyclin to the cell culture medium. The ChIP assay including crosslinking and harvest of cells were carried out using the Pierce Magnetic ChIP Kit (Thermo Scientific) according to the manufacturer’s protocol except that sonication was carried out on a Covaris S2 ultrasonicator (settings: burst: 15%, cycles: 200, intensity: 6, cycle time: 20 min, frequency sweeping: on, de-gas: on) and 10 μl Anti-FLAG M2 beads (Sigma-Aldrich) blocked with 10 ng/ml FLAG-peptid (Sigma-Aldrich) were used for IP of the FLAG-tagged MS2 coat protein. The antibodies used for the ChIP assay are listed in Supplementary file 2f. DNA fragments were quantified as described in the section ‘Quantitative PCR’ using the gene-specific primers listed in Supplementary file 2d.
Statistical analysis
Request a detailed protocolIn biochemical assays (conducted in at least biological triplicates), the significance of difference between samples were calculated by a two-tailed Student’s t test to test the null hypothesis of no difference between the two compared groups. The assumption of equal variances was tested by an F test. p<0.05 was considered statistically significant. Data are presented as mean ± SD.
Data availability
RNA sequencing data related to Figure 1 and Figure 1 supplement figure 1 has been deposited in GEO under the accesion code: GSE157788.
-
NCBI Gene Expression OmnibusID GSE157788. circZNF827 nucleates a transcription inhibitory complex to balance neuronal differentiation.
-
NCBI Gene Expression OmnibusID GSE65926. Circular RNAs in the mammalian brain are highly abundant, conserved, and dynamically expressed.
References
-
Roles of long noncoding RNAs and circular RNAs in translationCold Spring Harbor Perspectives in Biology 11:a032680.https://doi.org/10.1101/cshperspect.a032680
-
Phosphorylation of tristetraprolin by MK2 impairs AU-rich element mRNA decay by preventing deadenylase recruitmentMolecular and Cellular Biology 31:256–266.https://doi.org/10.1128/MCB.00717-10
-
Mis-splicing yields circular RNA moleculesThe FASEB Journal 7:155–160.https://doi.org/10.1096/fasebj.7.1.7678559
-
Translational coregulation of 5'TOP mRNAs by TIA-1 and TIARGenes & Development 25:2057–2068.https://doi.org/10.1101/gad.17355911
-
Insights into circular RNA biologyRNA Biology 14:1035–1045.https://doi.org/10.1080/15476286.2016.1271524
-
Circular RNA identification based on multiple seed matchingBriefings in Bioinformatics 19:803–810.https://doi.org/10.1093/bib/bbx014
-
Retinoids regulate stem cell differentiationJournal of Cellular Physiology 226:322–330.https://doi.org/10.1002/jcp.22417
-
Comparison of circular RNA prediction toolsNucleic Acids Research 44:e58.https://doi.org/10.1093/nar/gkv1458
-
Improved circRNA identification by combining prediction algorithmsFrontiers in Cell and Developmental Biology 6:20.https://doi.org/10.3389/fcell.2018.00020
-
Detecting and characterizing circular RNAsNature Biotechnology 32:453–461.https://doi.org/10.1038/nbt.2890
-
Efficient knockdown and lack of passenger strand activity by Dicer-Independent shRNAs expressed from pol II-Driven MicroRNA scaffoldsMolecular Therapy - Nucleic Acids 14:318–328.https://doi.org/10.1016/j.omtn.2018.11.013
-
Protein-protein interaction among hnRNPs shuttling between nucleus and cytoplasmJournal of Molecular Biology 298:395–405.https://doi.org/10.1006/jmbi.2000.3687
-
Exon-intron circular RNAs regulate transcription in the nucleusNature Structural & Molecular Biology 22:256–264.https://doi.org/10.1038/nsmb.2959
-
Stat3 controls maturation and terminal differentiation in mouse hippocampal neuronsJournal of Molecular Neuroscience 61:88–95.https://doi.org/10.1007/s12031-016-0820-x
-
RBPmap: a web server for mapping binding sites of RNA-binding proteinsNucleic Acids Research 42:W361–W367.https://doi.org/10.1093/nar/gku406
-
Circular RNA expression: its potential regulation and functionTrends in Genetics 32:309–316.https://doi.org/10.1016/j.tig.2016.03.002
-
Noncoding AUG circRNAs constitute an abundant and conserved subclass of circlesLife Science Alliance 2:e201900398.https://doi.org/10.26508/lsa.201900398
-
Analysis of qpcr data by converting exponentially related ct values into linearly related X0 valuesJournal of Bioinformatics and Computational Biology 08:885–900.https://doi.org/10.1142/s0219720010004963
-
Heterogeneous nuclear ribonucleoprotein K is a DNA-binding transactivatorJournal of Biological Chemistry 270:4875–4881.https://doi.org/10.1074/jbc.270.9.4875
Article and author information
Author details
Funding
Novo Nordisk (NNF17OC0028804)
- Anne Kruse Hollensen
Novo Nordisk (NNF15OC0017598)
- Anne Kruse Hollensen
Lundbeckfonden (R140-2013-13425)
- Anne Kruse Hollensen
Lundbeckfonden (R289-2018-1504)
- Christian Kroun Damgaard
Danish Cancer Society (R167-A11105)
- Andreas Bjerregaard Kamstrup
Aarhus Universitets Forskningsfond (NOVA grant)
- Henriette Sylvain Thomsen
The funders had no role in study design, data collection and interpretation, or the decision to submit the work for publication.
Acknowledgements
Proliferation assays using flow cytometry was performed at the FACS Core Facility, Aarhus University, Denmark. Karina Hjorth and Maria Vad Jakobsen are thanked for excellent technical assistance. Thanks to Serafin Pinol-Roma for sharing anti-hnRNP C1/C2 antibody.
Copyright
© 2020, Hollensen et al.
This article is distributed under the terms of the Creative Commons Attribution License, which permits unrestricted use and redistribution provided that the original author and source are credited.
Metrics
-
- 2,300
- views
-
- 293
- downloads
-
- 46
- citations
Views, downloads and citations are aggregated across all versions of this paper published by eLife.
Citations by DOI
-
- 46
- citations for umbrella DOI https://doi.org/10.7554/eLife.58478