Hepatic MIR20B promotes nonalcoholic fatty liver disease by suppressing PPARA
Abstract
Background:
Non-alcoholic fatty liver disease (NAFLD) is characterized by excessive lipid accumulation and imbalances in lipid metabolism in the liver. Although nuclear receptors (NRs) play a crucial role in hepatic lipid metabolism, the underlying mechanisms of NR regulation in NAFLD remain largely unclear.
Methods:
Using network analysis and RNA-seq to determine the correlation between NRs and microRNA in human NAFLD patients, we revealed that MIR20B specifically targets PPARA. MIR20B mimic and anti-MIR20B were administered to human HepG2 and Huh-7 cells and mouse primary hepatocytes as well as high-fat diet (HFD)- or methionine-deficient diet (MCD)-fed mice to verify the specific function of MIR20B in NAFLD. We tested the inhibition of the therapeutic effect of a PPARα agonist, fenofibrate, by Mir20b and the synergic effect of combination of fenofibrate with anti-Mir20b in NAFLD mouse model.
Results:
We revealed that MIR20B specifically targets PPARA through miRNA regulatory network analysis of nuclear receptor genes in NAFLD. The expression of MIR20B was upregulated in free fatty acid (FA)-treated hepatocytes and the livers of both obesity-induced mice and NAFLD patients. Overexpression of MIR20B significantly increased hepatic lipid accumulation and triglyceride levels. Furthermore, MIR20B significantly reduced FA oxidation and mitochondrial biogenesis by targeting PPARA. In Mir20b-introduced mice, the effect of fenofibrate to ameliorate hepatic steatosis was significantly suppressed. Finally, inhibition of Mir20b significantly increased FA oxidation and uptake, resulting in improved insulin sensitivity and a decrease in NAFLD progression. Moreover, combination of fenofibrate and anti-Mir20b exhibited the synergic effect on improvement of NAFLD in MCD-fed mice.
Conclusions:
Taken together, our results demonstrate that the novel MIR20B targets PPARA, plays a significant role in hepatic lipid metabolism, and present an opportunity for the development of novel therapeutics for NAFLD.
Funding:
This research was funded by Korea Mouse Phenotyping Project (2016M3A9D5A01952411), the National Research Foundation of Korea (NRF) grant funded by the Korea government (2020R1F1A1061267, 2018R1A5A1024340, NRF-2021R1I1A2041463, 2020R1I1A1A01074940, 2016M3C9A394589324), and the Future-leading Project Research Fund (1.210034.01) of UNIST.
Editor's evaluation
The manuscript by Lee et al. provides mechanistic insight into the regulatory role of micro RNAs in modulating nuclear receptor expression and function. This is likely to have a high impact on the field as nuclear receptor regulation of metabolic disease is well established, however, the molecular mechanisms governing this process still remains unknown largely. Lee et al.'s manuscript provides a molecular target (miR-20b) that holds therapeutic potential in improving hepatic steatosis.
https://doi.org/10.7554/eLife.70472.sa0Introduction
Obesity has emerged as a host of metabolic disorders, such as non-alcoholic fatty liver disease (NAFLD). Many reports have demonstrated that 90% of obese patients in the United States have NAFLD ranging from hepatic steatosis to much more severe forms of non-alcoholic steatohepatitis (NASH), which can induce fibrosis, cirrhosis, and hepatocellular carcinoma (HCC) (Corey and Kaplan, 2014). NAFLD is associated with hepatic metabolic reprogramming that leads to excessive lipid accumulation and imbalances in lipid metabolism in the liver (de Alwis and Day, 2008). Hepatic lipid homeostasis is appropriately described as a complex machinery involving signaling and transcriptional pathways, as well as targeted genes associated with fatty acid (FA) oxidation and lipogenesis (Fabbrini et al., 2010). Although the pathogenesis of NAFLD has been widely studied for years, the molecular mechanisms underlying its complicated disorder are still being investigated.
Nuclear receptors (NRs) are a superfamily of ligand-activated transcription factors that regulate biological homeostasis (McKenna et al., 2009). Recent evidence suggests that NRs are key regulators in the progression of diverse hepatic diseases associated with glucose and lipid metabolism, inflammation, bile acid homeostasis, fibrosis, and cancer development in the liver (López-Velázquez et al., 2012). Among them, growing evidence suggests a link between PPARα and obesity-induced NAFLD. Hepatic PPARα plays an important role in energy homeostasis by regulating the expression of genes required for FA uptake, FA oxidation, and triglyceride (TG) hydrolysis in the liver (Chakravarthy et al., 2005). The decreased expression of PPARα is significantly associated with severity in NAFLD patients (Francque et al., 2015). Therefore, understanding the molecular mechanism underlying PPARα regulation is critical for understanding the pathogenesis of NAFLD.
MicroRNAs (miRNAs) are short, non-coding RNA molecules with a length of 18–25 nucleotides that play an important role in regulating the expression of target genes in a post-transcriptional manner by targeting base-pairing with the 3’UTR of specific target mRNAs, inhibiting translation, or mRNA degradation (Bartel, 2004). These miRNAs contribute to the regulation of a wide variety of cellular functions and metabolic homeostasis, including fatty acid metabolism. Recent studies have suggested that miRNAs significantly regulate the pathogenesis of NAFLD by targeting the nuclear receptors (López-Sánchez et al., 2021). Previous report has demonstrated that MIR20B, a member of the MIR17 family, presents in the circulating plasma of NAFLD patients and has been highlighted as a novel biomarker of NAFLD and type 2 diabetes mellitus (T2DM) for the diagnosis and risk estimation of NAFLD (Ye et al., 2018). However, the mechanisms underlying the involvement of MIR20B in the occurrence and progression of NAFLD remain unknown. In this study, we analyzed the regulatory networks of miRNAs for NR genes and RNA-seq data in NAFLD patients, which prioritized MIR20B as a key regulator in NAFLD.
Materials and methods
Reagent type (species) or resource | Designation | Source or reference | Identifiers | Additional information |
---|---|---|---|---|
Genetic reagent(Mus. Musculus) | C57BL/6JbomTac | DBL | RRID:IMSR_TAC:b6jbom | |
Cell line (Homo sapiens) | HepG2 | ATCC | HB-8065, RRID:CVCL_0027 | |
Cell line (Homo sapiens) | Huh-7 | Dr. Yoshiharu Matsuura; originally from Japanese Collection of Research Bioresources Cell Bank | JCRB0403, RRID:CVCL_0336 | |
Antibody | Anti-PPARα(Rabbit Polyclonal) | abcam | Cat# ab24509, RRID:AB_448110 | WB(1:1000) |
Antibody | Anti-HSP90(Rabbit Polyclonal) | Cell Signaling Technology | Cat# 4,877 S, RRID:AB_2233307 | WB(1:1000) |
Sequence-based reagent | MIR20B/Mir20b(MIR20B/Mir20b mimic) | GenePharma | N/A | Sequence: CAAAGUGCUCAUAGUGCAGGUAG |
Sequence-based reagent | anti-MIR20B/Mir20b (MIR20B/Mir20b inhibitor) | GenePharma | N/A | Sequence: CUACCUGCACUAUGAGCACUUUG |
Sequence-based reagent | PPARA siRNA | GenePharma | N/A | Sequence: CGGCGAGGATAGTTCTGGAAGCTTT |
Sequence-based reagent | PPARA shRNA | Sigma-Aldrich | N/A | Sequence: GAACAGAAACAAATGCCAGTASequence:GTAGCGTATGGAAATGGGTTT |
Sequence-based reagent | Primers for qPCR | This paper | N/A | |
Recombinant DNA reagent | psiCHECK-2- PPARA-WT (plasmid) | This paper | N/A | |
Recombinant DNA reagent | psiCHECK-2- PPARA-Mut (plasmid) | This paper | N/A | |
Recombinant DNA reagent | pOTTC385-pAAV CMV-IE IRES EGFP-Mir20b | This paper | N/A | |
Recombinant DNA reagent | pOTTC385-pAAV CMV-IE IRES EGFP-anti-Mir20b | This paper | N/A | |
Commercial assay or kit | Dual-Luciferase kit | Promega | Cat# E1910 | |
Commercial assay or kit | RNeasy mini kit | Qiazen | Cat# 74,004 | |
Commercial assay or kit | QuickChange II Site-Directed Mutagenesis Kit | Agilent | Cat# 200,521 | |
Commercial assay or kit | AAVpro Purification Kit | Takara Bio. | Cat# 6,675 | |
Commercial assay or kit | Triglyceride Colorimetric Assay Kit | Cayman Chemical | Cat# 10010303 | |
Commercial assay or kit | Alanine Transaminase Colorimetric Activity Assay Kit | Cayman Chemical | Cat# 700,260 | |
Commercial assay or kit | Aspartate Aminotransferase Colorimetric Activity Assay Kit | Cayman Chemical | Cat# 701,640 | |
Commercial assay or kit | Mouse Insulin ELISA Kit | Crystal Chem | Cat# 90,080 | |
Commercial assay or kit | PicoSens Free Fatty Acid Quantification Kit | BioMax | Cat# BM-FFA-100 | |
Commercial assay or kit | Β-hydroxybutyrate assay Kit | abcam | Cat# ab83390 | |
Chemical Compound, drug | Oleic acid | Sigma-Aldrich | Cat# O1008 | |
Chemical Compound, drug | Fenofibrate | Santa Cruz biotechnology | Cat# sc-204751 |
|
Cell culture
Request a detailed protocolHuman liver cells, HepG2 and Huh-7 cells were purchased from American Type Culture Collection (ATCC, Manassas, VA) and cultured in Dulbecco’s Modified Eagle’s Medium (DMEM) containing 10% fetal bovine serum (Gibco, BRL, Grand Island, NY) and 1% penicillin/streptomycin (ThermoFisher Scientific, Waltham, MA). miRNAs and siRNA were obtained from GenePharma (Shanghai, China). The miRNAs and siRNA used in this study are listed in the table below. HepG2 and Huh-7 cells were transfected with miRNA or siRNA using Lipofectamine RNAiMAX transfection reagent (ThermoFisher Scientific) according to the manufacturer’s instructions and following experiments were performed 48 hr after transfection. For intracellular lipid accumulation, cells were cultured in a medium with the addition of 1 mmol/L sodium oleic acid for 24 hr and then cells were harvested for further analysis. Images of cells stained with Oil Red O were obtained with EVOS FL (Thermo Fisher Scientific). For quantification, Oil Red O was extracted with isopropanol and absorbance was measured at 520 nm (Lim et al., 2012).
Name | Sequence |
---|---|
MIR NC | UCACAACCUCCUAGAAAGAGUAGA |
MIR20B/Mir20b (Mimic) | CAAAGUGCUCAUAGUGCAGGUAG |
anti-MIR20B/Mir20b (Inhibitor) | CUACCUGCACUAUGAGCACUUUG |
siPPARA | CGGCGAGGATAGTTCTGGAAGCTTT |
Human patients
Request a detailed protocolHuman liver tissue samples of 21 patients were acquired from the BioResource Center (BRC) of Asan Medical Center, Seoul, Republic of Korea. The process of 21 human tissue samples was officially approved by the Institutional Review Board of Asan Medical Center (IRB approval number: 2018–1512). Human samples used in this study were from hepatocellular carcinomas (HCC) liver resection. All samples were taken from non-tumor parts of the resected tissue and selected as 11 normal liver samples, 4 simple steatosis, and 6 NASH samples through histological analysis of pathologists using the grading and staging system for NASH (Brunt et al., 1999). Although limitations in completely excluding the effect of HCC, histologically normal tissue adjacent to the tumor has obvious distinctions in global transcriptome patterns for the tumor and can be used as a control (Aran et al., 2017). There were no significant differences in age and male/female ratio among the three groups. Specifically, serum AST, ALT, and fasting glucose levels were significantly increased with progression from normal to NASH, but total cholesterol was comparable as previously reported (Chung et al., 2020). In addition, all patients diagnosed with alcoholic liver disease, viral infected hepatitis, and toxic hepatitis were excluded.
Library preparation for transcriptome sequencing
Request a detailed protocolRNA-seq was performed on triplicate sample from HepG2 cell with or without overexpression of MIR20B. Total RNA was isolated using the RNeasy mini kit (Qiagen, Hilden, Germany) according to the manufacturer’s instructions. Library prep and RNA-seq were performed by Novogene (Hong Kong). A total amount of 1 μg RNA per sample was used as input material for the RNA sample preparations. Sequencing libraries were generated using NEBNext UltraTM RNA Library Prep Kit for Illumina (NEB, Ipswich, MA) following manufacturer’s recommendations and index codes were added to attribute sequences to each sample. PCR products were purified (AMPure XP system, Beckman Coulter Life Sciences, Indianapolis, IN) and library quality was assessed on the Agilent Bioanalyzer 2,100 system (Agilent Technologies, Inc, Santa Clara, CA). The clustering of the index-coded samples was performed on a cBot Cluster Generation System using PE Cluster Kit cBot-HS (Illumina, San Diego, CA) according to the manufacturer’s instructions. After cluster generation, the library preparations were sequenced on an Illumina platform (NovaSeq 6000 PE150) and paired-end reads were generated.
miRNA regulatory network analysis of nuclear receptor genes
Request a detailed protocolThe RNA-seq fold change data for 16,010 genes were obtained from the Supplementary file 1 of a previous work (Hoang et al., 2019). The miRNA target genes that shared evolutionarily conserved binding sites for 353 miRNAs ( were downloaded from TargetScan database (Ver.7.2)) (Agarwal et al., 2015). We used the 50 genes that belonged to the nuclear receptor transcription pathway in REACTOME database (Jassal et al., 2020; Liberzon et al., 2015) to analyze how miRNAs regulate the transcription of NR genes. Among them 17 genes were significantly downregulated in NASH patients (adjusted p-value < 0.1). The enrichment of these downregulated NR genes in the targets of the 353 miRNAs were assessed using hypergeometric distribution. The p-value of a miRNA indicates the probability of having downregulated nuclear receptors among the miRNA targets. The p-value is given as follows:
where N is the total number of genes analyzed, M is the number of candidate target genes of the miRNA, D is the downregulated NR genes, and O is the observed overlap between miRNA targets and the downregulated NR genes.
The miRNAs whose target genes were enriched in the downregulated NR genes with adjusted p-value ≤ 0.05 were used to construct the regulatory networks of the NR transcription pathway (Figure 1A).
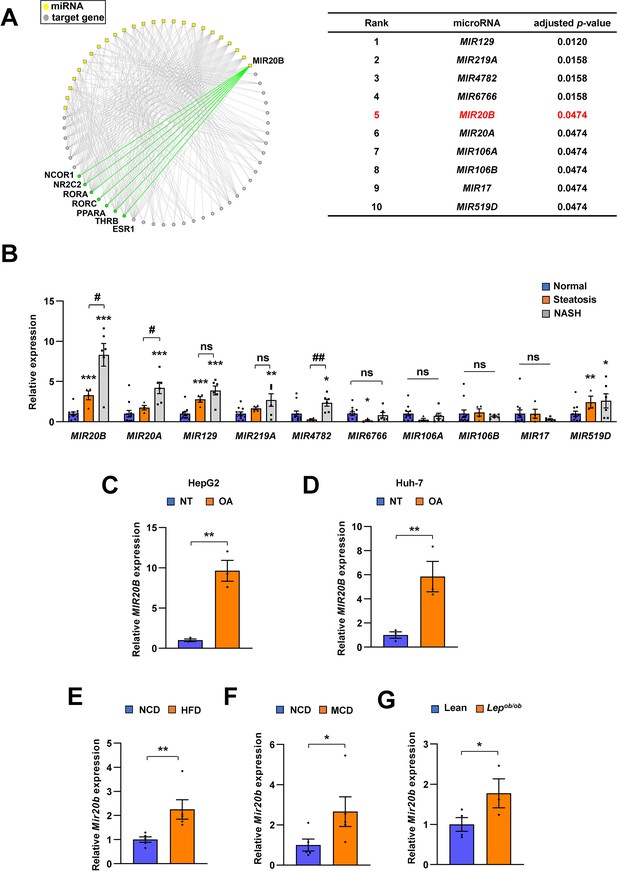
MIR20B expression is significantly increased in the livers of dietary obese mice and humans.
The miRNA regulatory networks for NR genes downregulated in the transcriptome of NAFLD patients. The adjusted p-values in the table represent the enrichment of miRNA targets in the downregulated NR genes (hypergeometric distribution) (A). The expression of miRNAs was measured in indicated condition by quantitative RT-PCR (B–G). Hepatic miRNA levels of patients with steatosis (n = 4) or NASH (n = 6) were normalized to those of normal individuals (n = 11). *p < 0.05, **p < 0.01 and ***p < 0.001 vs normal individuals. #p < 0.05 and ##p < 0.01 vs patients with steatosis (B). MIR20B levels from HepG2 cells (C) and Huh-7 cells (D) treated with OA for 24 hr were normalized to no treatment (NT). Hepatic Mir20b levels from C57BL/6 J mice fed high-fat diet (HFD) (E), or methionine-deficient diet (MCD) (F) were normalized to normal chow diet (NCD). Hepatic Mir20b levels from leptin-deficient Lepob/ob mice were normalized to lean wild mice (G). Values represent means ± SEM (n = 3–5). *p < 0.05, **p < 0.01 vs NT in cells or NCD-fed mice. Raw data can be found in a Source Data file named ‘Figure 1—source data 1’.
-
Figure 1—source data 1
MIR20B expression is significantly increased in the livers of dietary obese mice and humans.
- https://cdn.elifesciences.org/articles/70472/elife-70472-fig1-data1-v2.xlsx
Differential expression and gene-set enrichment analysis (GSEA)
Request a detailed protocolThe differential expression analysis of RNA-seq data were performed using limma package (Ritchie et al., 2015) where moderated t-test was applied for voom-transformed read counts. The resulting fold-change values between the test and control conditions were used for the pathway analysis. The preranked GSEA (R package) (Subramanian et al., 2005) was used for the pathway analysis of gene sets from WikiPathway (Martens et al., 2021), REACTOME (Jassal et al., 2020), KEGG (Kanehisa et al., 2017) databases (MSigDB) and the enrichment score plots.
Immunoblotting
Request a detailed protocolSupernatants containing protein contents were determined by Bradford Protein Assay (Bio-Rad Laboratories, Hercules, CA). Proteins were immunoblotted with anti-PPARα (ab24509, Abcam, Cambridge, MA) and anti-HSP90 (4,877 S, Cell Signaling Technology, Danvers, MA).
Quantitative PCR
Request a detailed protocolTotal mRNAs were isolated using TRIzol reagent purchased from Thermo Fisher Scientific. Reverse-transcription of the RNA was performed with ABI Reverse Transcription Kit (Thermo Fisher Scientific). Quantitative PCR was performed using 7900HT Fast Real-Time PCR System (Life Technologies, Carlsbad, CA) following the manufacturer’s instructions. Relative mRNA expression levels of each gene were normalized to TATA-binding protein TBP. The mtDNA copy number was evaluated based on the ratio of mtDNA to nuclear DNA by quantitative PCR. The mtDNA was quantified based on the mitochondrial gene, VIPR1, and MT-ATP6, respectively. The relative amounts of mtDNA were normalized to nuclear DNA, B2M. The primer pairs used in this study are listed in the table below.
Sequences of the primers for qPCR | ||
---|---|---|
Human Gene | Forward primer | Reverse primer |
MIR20B | GCAAAGTGCTCATAGTGCAGGTAG | TCGCACTTGTCATACACCAG |
MIR129 | CTTTTTGCGGTCTGGGCTTGC | AGTGCAGGGTCCGAGGTATT |
MIR219A | TGATTGTCCAAACGCAATTC | GAACATGTCTGCGTATCTC |
MIR4782 | TTCTGGATATGAAGACAATCAA | CAAGATCTATACTTCTGTTAGT |
MIR6766 | CGGGTGGGAGCAGATCTTAT | GAACATGTCTGCGTATCTC |
MIR20A | TGCGCTAAAGTGCTTATAGTGC | CCAGTGCAGGGTCCGAGGTATT |
MIR106A | GATGCTCAAAAAGTGCTTACAGTGCA | TATGGTTGTTCTGCTCTCTGTCTC |
MIR106B | CAAGTACCCACAGTGCGGT | CTCGCTTCGGCAGCACA |
MIR17 | TCTAGATCCCGAGGACTG | ATCGTGACCTGAACC |
MIR519D | AGTGCCTCCCTTTAGAG | GAACATGTCTGCGTATCTC |
U6 | CTCGCTTCGGCAGCACA | AACGCTTCACGAATTTGCGT |
PPARA | GCTATCATTACGGAGTCCACG | TCGCACTTGTCATACACCAG |
CPT1A | AGGCGACATCAATCCGAAC | AAAGGCTACGAATGGGAAGG |
ACOX1 | CCACGTATGACCCTGAAACC | TCCATAGCATTTCCCCTTAGTG |
SREBF1 | CAACACAGCAACCAGAAACTC | CTCCACCTCAGTCTTCACG |
FASN | CAAGCTGAAGGACCTGTCTAG | CGGAGTGAATCTGGGTTGATG |
CD36 | GCCAGGTATTGCAGTTCTTTTC | TGTCTGGGTTTTCAACTGGAG |
FABP1 | GCAGAGCCAGGAAAACTTTG | AGCGGTGATGGTGAACTTG |
PPARGC1A | ACCAAACCCACAGAGAACAG | GGGTCAGAGGAAGAGATAAAGTTG |
SIRT1 | CCCTCAAAGTAAGACCAGTAGC | CACAGTCTCCAAGAAGCTCTAC |
VIPR1 | CTCCACCATTAGCACCCAAAGCTAAG | GATATTGATTTCACGGAGGATGGTGGTC |
MT-ATP6 | AACGAAAATCTGTTCGCTTCAT | ATGTGTTGTCGTGCAGGTAGAG |
HMGCL | GAGTTTTCAGAGGTTTGACGC | CAAGAGCACAGGAGACGTAC |
ACAT1 | CGGGCTAACTGATGTCTACAA | CAAATTTCCCAGCTTCCCATG |
ACAT2 | CCCAGAACAGGACAGAGAATG | AGCTTGGACATGGCTTCTATG |
RORA | GGTGATGCTTTTGTTCTTACTGG | TGTCTCCACAGATCTTGCATG |
RORC | TGGTGCTGGTTAGGATGTG | GGAGTGGGAGAAGTCAAAGATG |
THRB | CATCAAAACTGTCACCGAAGC | TCCAAGTCAACCTTTCCACC |
NRBP1 | GTTCCACCCAGCATTGTTTG | CAGGGATTTCAGCCAGTACG |
NAGK | TATTTCCAGGTGCCAGATCG | CTGAAGATATAGCGGGAAAGGG |
USP46 | ATACACCAAGCTGTCTTACCG | ATATAATGCCCACGATTAGGACC |
ITGB8 | CGTCTCATCTCGCTCTTGATAG | TTCTCTGAAAGTTGGCCTAGTG |
BMPR2 | GGCTGACTGGAAATAGACTGG | CACAGTCCCTCAAGTTCACAG |
ZNFX1 | CCGAGGATTGTCATAGTGGAAG | AGATCATACACGTTGGCACTG |
EPHA5 | AGATTGAGGCAGTGAATGGAG | GCCAAGACAAAGAGATGCTG |
E2F1 | TCTCCGAGGACACTGACAG | ATCACCATAACCATCTGCTCTG |
AGPAT1 | AGGACGCAACGTCGAGAAC | GCAGTACCTCCATCATCCCAAG |
AGPAT2 | GCCGAGTTCTACGCCAAGG | CGAACCAGCCGATGATGCT |
GPAT1 | GATGTAAGCACACAAGTGAGGA | TCCGACTCATTAGGCTTTCTTTC |
GPAT2 | TGTGGTCGTCAGGCTTTGG | GGTCCGTTATGCTTCTGTGGA |
DGAT1 | TATTGCGGCCAATGTCTTTGC | CACTGGAGTGATAGACTCAACCA |
DGAT2 | ATTGCTGGCTCATCGCTGT | GGGAAAGTAGTCTCGAAAGTAGC |
FATP2 | TTTCCGCCATCTACACAGTCC | CGTAGGTGAGAGTCTCGTCG |
FATP3 | CCCTGCTGGAATTAGCGATTT | GGGCGAGGTAGATCACATCTT |
FATP4 | CTCAGGTCTTGGAGAAGGAAC | ACAGCGGGTCTTTCACAATAG |
FATP5 | TGGAGGAGATCCTTCCCAAGC | TGGTCCCCGAGGTATAGATGAA |
FATP6 | CTTCTGTCATGGCTAACAGTTCT | AGGTTTCCGAGGTTGTCTTTTG |
CPT1B | ATCCTACTCCTATGACCCCG | TCTGCATTGAGACCCAACTG |
CPT2 | TTGAGTGCTCCAAGTACCATG | GCAAACAAGTGTCGGTCAAAG |
ACADM | TCATTGTGGAAGCAGATACCC | CAGCTCCGTCACCAATTAAAAC |
FATP1 | ATGAGGACACAATGGAGCTG | TGACATAGCCATCGAAGCG |
ACAA1 | GCGGTTCTCAAGGACGTGAAT | GTCTCCGGGATGTCACTCAGA |
ACSL1 | CCATGAGCTGTTCCGGTATTT | CCGAAGCCCATAAGCGTGTT |
TBP | CCACTCACAGACTCTCACAAC | CTGCGGTACAATCCCAGAACT |
B2M | TGCTGTCTCCATGTTTGATGTATCT | TCTCTGCTCCCCACCTCTAAGT |
Sequences of the primers for qPCR | ||
Mouse Gene | Forward primer | Reverse primer |
Mir20b | GCAAAGTGCTCATAGTGCAGGTAG | TCGCACTTGTCATACACCAG |
Mir129 | CTTTTTGCGGTCTGGGCTTGC | AGTGCAGGGTCCGAGGTATT |
Mir219a | TGATTGTCCAAACGCAATTC | GAACATGTCTGCGTATCTC |
Mir20a | TGCGCTAAAGTGCTTATAGTGC | CCAGTGCAGGGTCCGAGGTATT |
Mir106a | GATGCTCAAAAAGTGCTTACAGTGCA | TATGGTTGTTCTGCTCTCTGTCTC |
Mir106b | CAAGTACCCACAGTGCGGT | CTCGCTTCGGCAGCACA |
Mir17 | TCTAGATCCCGAGGACTG | ATCGTGACCTGAACC |
Mir519d | AGTGCCTCCCTTTAGAG | GAACATGTCTGCGTATCTC |
U6 | CTCGCTTCGGCAGCACA | AACGCTTCACGAATTTGCGT |
Ppara | TCAGGGTACCACTACGGAGT | CTTGGCATTCTTCCAAAGCG |
Cpt1a | AGTTCCATGACCCATCTCTGTC | TTCTTCTTCCAGAGTGCAGC |
Acox1 | TAACTTCCTCACTCGAAGCCA | AGTTCCATGACCCATCTCTGTC |
Srebf1 | GGAGCCATGGATTGCACATT | CTTCCAGAGAGGAGGCCAG |
Fasn | GGAGGTGGTGATAGCCGGTAT | TGGGTAATCCATAGAGCCCAG |
Cd36 | GCGACATGATTAATGGCACAG | GATCCGAACACAGCGTAGATAG |
Fabp1 | TCTCCGGCAAGTACCAATTG | TTGATGTCCTTCCCTTTCTGG |
Tnf | CCCTCACACTCAGATCATCTTCT | GCTACGACGTGGGCTACAG |
Ccl2 | TTAAAAACCTGGATCGGAACCAA | GTTCACCGTAAGCCCAATTT |
Il6 | TAGTCCTTCCTACCCCAATTTCC | TTGGTCCTTAGCCACTCCTTC |
Il1b | GCACTACAGGCTCCGAGATGAAC | TTGTCGTTGCTTGGTTCTCCTTGT |
Acta2 | GTGAAGAGGAAGACAGCACAG | GCCCATTCCAACCATTACTCC |
Col1a1 | CATAAAGGGTCATCGTGGCT | TTGAGTCCGTCTTTGCCAG |
Col3a1 | GAAGTCTCTGAAGCTGATGGG | TTGCCTTGCGTGTTTGATATTC |
Fn | CTTTGGCAGTGGTCATTTCAG | ATTCTCCCTTTCCATTCCCG |
Timp1 | CTCAAAGACCTATAGTGCTGGC | CAAAGTGACGGCTCTGGTAG |
Vim | CGTCCACACGCACCTACAG | GGGGGATGAGGAATAGAGGCT |
E2f1 | TGCAGAACAGATGGTCATAGTG | GGGCACAGGAAAACATCAATG |
Rora | GTGGAGACAAATCGTCAGGAAT | TGGTCCGATCAATCAAACAGTTC |
Rorc | TCCACTACGGGGTTATCACCT | AGTAGGCCACATTACACTGCT |
Thrb | GGACAAGCACCCATCGTGAAT | CTCTGGTAATTGCTGGTGTGAT |
Cpt1b | GACTTCCGGCTTAGTCGGG | GAATAAGGCGTTTCTTCCAGGA |
Cpt2 | CAGCACAGCATCGTACCCA | TCCCAATGCCGTTCTCAAAAT |
Acadm | AACACAACACTCGAAAGCGG | TTCTGCTGTTCCGTCAACTCA |
Fatp1 | CTG GGA CTT CCG TGG ACC T | TCT TGC AGA CGA TAC GCA GAA |
Acaa1 | TCT CCA GGA CGT GAG GCT AAA | CGC TCA GAA ATT GGG CGA TG |
Acsl1 | TGC CAG AGC TGA TTG ACA TTC | GGC ATA CCA GAA GGT GGT GAG |
Atgl | ATGTTCCCGAGGGAGACCAA | GAGGCTCCGTAGATGTGAGTG |
Hsl | GATTTACGCACGATGACACAGT | ACCTGCAAAGACATTAGACAGC |
Mgl | ACCATGCTGTGATGCTCTCTG | CAAACGCCTCGGGGATAACC |
Tbp | ACCCTTCACCAATGACTCCTATG | TGACTGCAGCAAATCGCTTGG |
Cellular oxygen consumption rate (OCR)
Request a detailed protocolOCR of HepG2 cells were analyzed by Seahorse XF24 extracellular flux analyzer (Seahorse Bioscience, North Billerica, MA) following the manufacturer’s instruction. The results were normalized with the protein quantity of each corresponding well.
Measurement of FA Β-oxidation and uptake
Request a detailed protocolFA β-oxidation were measured by the conversion of [9,10-3H(N)]-Palmitic Acid (PerkinElmer, Waltham, MA) to 3H2O. Cells were incubated with 1.25 mCi/L [9,10-3H(N)]-Palmitic Acid with cold palmitic acid in a final concentration of 200 μM for 4 hr. After incubation, medium were recovered and precipitated with an equal volume of 10% tricholoroacetic acid. The supernatant was transferred to new- and capless-microtube and the capless-tube was inserted into D.W-added Scintillation tube and incubated at 60 °C for 12 hr. The capless tube was removed from scintillation tube and measured the CPMA with scincillation counter oil using Tri-carb 2910TR liquid scintillation counter (PerkinElmer).
For FA uptake measurement, cells were incubated with 0.5 μCi/L [9,10-3H(N)]-Palmitic Acid with cold palmitic acid in a final concentration of 200 μM for 2 hours. Uptake was stopped by addition of 200 μM phloretin in 0.1% BSA and lysed in 0.1 N NaOH / 0.03% SDS buffer. The radioactivity of each lysate was counted using Tri-carb 2910TR liquid scintillation counter. The β-oxidation and uptake was normalized to lysate protein concentration determined by BSA assay.
Metabolites assay
Request a detailed protocolHepG2 cells were transfected with MIR20B, anti-MIR20B, or MIR NC, respectively. After 48 hr, HepG2 cells (20,000 cells per well) were seeded in 96-well MitoPlate S-1 plates and examined in mitochondrial metabolites activity following the manufacturer’s instructions (Biolog, Hayward, CA).
Mice
All animal experiments were performed according to procedures approved by the Ulsan National Institute of Science and Technology’s Institutional Animal Care and Use Committee (UNISTIACUC-19–04). Mice were maintained in a specific pathogen-free animal facility under a 12 hr light/dark cycle at a temperature of 21 °C and allowed free access to water and food. Seven-week-old male C57BL/6 J mice (DBL, Chungbuk, Republic of Korea) were fed a HFD (60% kcal fat, D12492, Research Diets Inc, New Brunswick, NJ) for 12 weeks or a MCD (A02082002BR, Research Diets Inc, New Brunswick, NJ, USA) for 4 weeks. Fenofibrate (100 mg/kg, sc-204751, Santa Cruz biotechnology, Dallas, TX) was administered orally for 4 weeks before mice were sacrificed.
Hepatocyte isolation
Request a detailed protocolBriefly, mice were anesthetized with isoflurane, and 24-gauge needle was inserted into the portal vein. Then the inferior vena cava was cut, and the mouse liver was perfused sequentially with solution I (142 μM NaCl, 6.7 μM KCl, 10 μM HEPES, and 2.5 mM EGTA), and solution II (66.7 mM NaCl, 6.7 mM KCl, 50 mM HEPES, 4.8 mM CaCl2·2H2O, and 0.01% Type IV collagenase (Sigma- Aldrich, St. Louis, MO)). After digestion, the liver was disrupted over a 70 μm cell strainer, and cell suspension was spun at 50 x g for 5 min at 4 °C. The supernatant was gently aspirated and the cells were resuspended in M199 with EBSS (M199/EBSS) medium and gently mixed with equal volume of Percoll working solution (48.6% Percoll). The cell suspension was spun at 100 x g for 5 min at 4 °C, and the pellet washed once with M199/EBSS. After viable cells were counted with trypan blue, the isolated hepatocytes were seed in M199/EBSS medium supplemented with 10% FBS, 1% penicillin/streptomycin, and 10 nM dexamethasone.
Production of AAV
Request a detailed protocolThe Mir20b and anti-Mir20b were cloned into the pOTTC385-pAAV CMV-IE IRES EGFP vector (Addgene plasmid # 102936) (Nelson et al., 2019) and co-transfected with pAAV-DJ vector and pAAV-Helper vector into HEK 293T cells to generate recombinant adeno-associated virus expressing Mir20b or anti-Mir20b according to the manufacturer’s protocol (Cell Biolabs, San Diego, CA). The AAVs were purified with AAVpro Purification Kit (Takara Bio, Shiga, Japan). When feeding HFD was started, purified AAV-Mir20b, AAV-anti-Mir20b, or AAV-Control (1 × 1010 PFU) was injected into mice via tail-vein. AAV-Control or AAV-anti-Mir20b was injected into mice via tail-vein before the initiation of MCD diet.
Lentivirus preparation, transduction, and stable cell line generation
Request a detailed protocolAll lentivirus-based shRNA clones used for making the viral transduction particles were purchased from Sigma-Aldrich. pLKO.1-Puro vector targeting human PPARA or non-target vector as a control was used. In short, 293T cells were plated at a density of 12 × 106 293 T cells in a 150 mm dish containing 20 mL of media. After 24 hr, the cells were overlaid with a complex containing a three-plasmid system (pLKO.1 shRNA, pCMV-VSVG, and pCMV-Δ8.9) at the ratio of 4:2:3 using lipofectamine (Jang et al., 2020). The supernatant was collected starting from 48 to 72 hr post-transfection. The virus particles were concentrated by ultracentrifugation at 25,000 rpm for 1 hr 30 min with a Beckman ultracentrifuge using the SW28 rotor, resuspended in PBS, and stored until use at −70 °C. Harvested virus was used to transduce target cells for 24 hr. Stable cell lines were selected in the presence of 3 μg/mL puromycin for 5 days. To minimize batch-to-batch differences, we compared only cells made from the same batch of cells.
Name | Sequence |
---|---|
shPPARA #1 | GAACAGAAACAAATGCCAGTA |
shPPARA #2 | GTAGCGTATGGAAATGGGTTT |
Metabolic analysis
Request a detailed protocolMice were fasted overnight (18 hr) before intraperitoneal injection of D-glucose (2 g/kg body weight) for glucose tolerance test. For insulin tolerance test, mice were fasted for 4 hr before intraperitoneal injection of insulin (0.75 U/kg body weight). Every glucose was examined with tail-vein blood at indicated intervals after injection using a glucometer. For analyzing metabolic parameters, insulin (90080, Crystal Chem, Elk Grove Village, IL), ALT (K752, Biovision Inc, Milpitas, CA), AST (K753, Biovision Inc), cholesterol (K603, BioVision Inc), TG (10010303, Cayman Chemical, Ann Arbor, MI), and FFA (BM-FFA-100, BioMax, Republic of Korea) were determined. Body composition of mice was measured using an EchoMRI100V, quantitative nuclear resonance system (Echo Medical Systems, Houston, TX).
Histological analysis
Request a detailed protocolLiver tissues were isolated from mice and immediately fixed with 4% formalin (Sigma- Aldrich, St. Louis, MO). Histological changes of lipid droplets were examined by H&E staining and Oil Red O staining. As counterstain, Mayer’s hematoxylin was used for every slide. Liver fibrosis was further examined by Sirius red with liver section. Images were obtained with Olympus BX53 microscope and DP26 camera. NAFLD activity score (NAS) and fibrosis score were measured by a pathologist according to the NASH Clinical Research Network scoring system (Brunt et al., 2011). For statistical analysis of fibrosis score, each grade (0~4) was calculated by giving a score from 1 to 6 points.
Statistical analysis
Request a detailed protocolAll data are represented as mean ± SEM. Statistically significant differences were assessed by the Student’s t-test. Statistical analyses and Pearson correlation analyses were performed using Microsoft Excel or GraphPad Prism 9.3.0 (RRID:SCR_002798). All the significance are expressed as *p < 0.05, **p < 0.01, ***p < 0.001, #p < 0.05, ##p < 0.01, ###p < 0.001, $p < 0.05, $$p < 0.01, and $$$p < 0.001. ns, not significant.
Results
MIR20B significantly increases in the livers of dietary obese mice and human
We constructed a regulatory network of NRs that were differentially expressed in NAFLD patients (Hoang et al., 2019) and microRNA targeting NRs based on miRNA target prediction (Agarwal et al., 2015), to identify the correlation between NR and microRNA in the development of NAFLD. As shown in Figure 1A, the top ten miRNAs were found to be highly correlated with the modulation of NR expression in NAFLD. To further prioritize key miRNAs, we assessed the expression of miRNA candidates in NASH, simple steatosis, and normal patient liver samples (Figure 1B, Figure 1—figure supplement 1). Among the selected miRNAs, the expression of MIR20B was significantly increased in simple steatosis and NASH compared to that in normal individuals, and the extent of increase in NASH was higher than that in simple steatosis. In particular, the expression of MIR20B showed a significant correlation with the BMI, AST, ALT, and fasting glucose during NAFLD progression (Figure 1—figure supplement 2).
MIR20B expression was also most significantly increased in both oleic acid (OA)-treated HepG2 and Huh-7 cells (Figure 1C and D, Figure 1—figure supplement 4A, B). Moreover, the expression of MIR20B was significantly upregulated in the fatty livers of high-fat diet (HFD)-fed mice, Lepob/ob mice, and methionine-deficient diet (MCD)-fed mice compared to that in the liver of normal chow diet (NCD)-fed wild mice (Figure 1E–G, Figure 1—figure supplement 3, and Figure 1—figure supplement 4C). Together, our results indicate that MIR20B expression is increased in NAFLD and is highly associated with the regulation of NRs in NAFLD.
PPARA is a direct target of MIR20B
Next, we characterized the physiological roles of MIR20B in NAFLD. Oil Red O staining showed that MIR20B expression increased intracellular lipid content, and this lipid accumulation was increased with OA treatment in HepG2 cells (Figure 2A). As expected, overexpression of MIR20B significantly upregulated TG content and cholesterol in OA-treated HepG2 cells (Figure 2B and C). To further investigate the functions and targets of MIR20B, HepG2 cells were transfected with MIR20B and two separate RNA-Seq libraries of non-targeting control (MIR NC) and forced MIR20B expression (MIR20B) were constructed. The six RNA-seq samples were clearly separated between the forced MIR20B expression and control conditions, implying a significant impact on gene expression in HepG2 cells (Figure 2D, Figure 2—figure supplement 1A). The NR metapathway was detected as the most significant pathways (adjusted p-value = 2.47-E8) by the gene-set enrichment analysis (GSEA) (Subramanian et al., 2005), indicating that NRs and related genes are the major targets of MIR20B (Figure 2E and F). The heatmap of RNA-seq data for the NR transcription pathway is shown in Figure 2G. Five NRs (PPARA, RORA, RORC, THRB, and NRBP1) were downregulated (adjusted p-value ≤ 0.05), and PPARA was the most significantly downregulated NR (adjusted p-value = 3.25-E5). Furthermore, GSEA showed that PPARA pathways and PPAR signaling pathways were significantly decreased in MIR20B overexpressed cells (Figure 2—figure supplement 1B, C). We validated the expression of these NRs and the PPARA, RORC, THRB, and NRBP1 expression was decreased by MIR20B in human liver cells and mouse primary hepatocytes. Consistent with the RNA-seq data, the expression change of PPARA at both the protein and mRNA levels with MIR20B transfection was the most distinct compared to the control (Figure 2H1). Moreover, among candidate targets from RNA-seq analysis, only PPARA was selected as an overlapped predicted target of MIR20B between various miRNA target prediction programs, including miRDB, picTAR, TargetSCAN, and miRmap (Figure 2J, Figure 2—figure supplement 2). While the expression of other candidates, including RORA, RORC, and THRB, remained unchanged, that of PPARA significantly decreased as NAFLD progressed to steatosis and NASH, and showed a negative correlation with that of MIR20B (Figure 2—figure supplement 3). In addition, PPARA decreased in diverse diets-induced NAFLD conditions with MIR20B (Figure 1C–E, Figure 2—figure supplement 4).
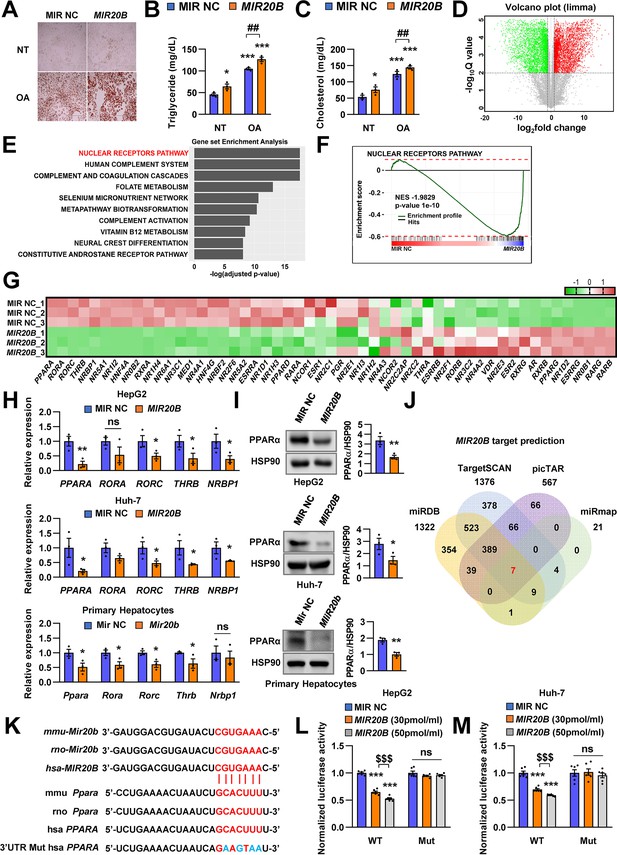
PPARA is a direct target of MIR20B.
Overexpressed MIR20B induces hepatic lipid accumulation in HepG2 cells treated with OA (1 mM). Oil Red O staining showed intracellular lipid accumulation in HepG2 cells. Scale bar is 400 µm (A). TG (B) and Cholesterol levels (C) were examined in OA-treated HepG2 cells transfected with MIR NC or MIR20B. RNA-seq was performed on sample from HepG2 cell with or without overexpression of MIR20B.Volcano plot of the gene expressions (log2 fold change) compared to the negative control from RNA-seq analysis (D). Top ranked GSEA in overexpressed MIR20B compared to MIR NC in HepG2 cells (E). The primary ranked enrichment plot of nuclear receptors pathway (F). Heatmap of the genes in NR pathway upon MIR20B overexpression compared to control (G). Expression of primary ranked nuclear receptors pathway genes from RNA-seq analysis in HepG2 cells, Huh-7 cells, and primary hepatocytes transfected with MIR20B were normalized to each cells transfected with MIR NC (H). Western blot analysis of PPARα on MIR NC or MIR20B transfected cells. The intensity of PPARα blot was normalized to that of HSP90 (I). Venn diagram of predicted targets for MIR20B in four major database system (J). Graphic image of the conserved binding motifs of MIR20B within 3’UTR mRNA of PPARA (K). Luciferase activities of MIR20B-transfected HepG2 cells and Huh-7 cells containing the luciferase reporter DNA constructs for wild-type or mutated 3’UTR of PPARA were normalized to those or MIR NC-transfected cells (L, M). Values represent means ± SEM (n = 3). *p < 0.05, **p < 0.01, ***p < 0.001 vs MIR NC. ##p < 0.01 vs MIR NC+ OA. $$$ < 0.001 vs MIR20B (30 pmol/ml). Raw data can be found in a Source Data file named ‘Figure 2—source data 1’.
-
Figure 2—source data 1
PPARA is a direct target of MIR20B.
- https://cdn.elifesciences.org/articles/70472/elife-70472-fig2-data1-v2.xlsx
Notably, the 3’UTR of PPARA mRNA includes MIR20B binding sites that are well conserved between humans and mice, suggesting that MIR20B may have a direct inhibitory effect on PPARA expression (Figure 2K). Using a luciferase reporter construct including the 3’UTR of PPARA, we revealed that MIR20B suppressed the luciferase activity in both HepG2 and Huh-7 cells in a dose-dependent manner. Furthermore, we built the mutant construct of the predicted MIR20B-binding sites within the 3’UTR of PPARA; the inhibitory effect of MIR20B on luciferase activity was completely blunted (Figure 2L and M). To further validate the functional role of 3’UTR regulated by MIR20B, we introduced the wild-type PPARA open-reading frame (ORF) followed by either the wild type (WT) or mutant (Mut) 3’UTR of PPARA in HepG2 cells (Figure 2—figure supplement 5A). As shown in Figure 2—figure supplement 5C, MIR20B significantly suppressed the expression of PPARA and its target genes in PPARA-3’UTR WT expressing cells. Furthermore, Oil Red O staining showed that MIR20B increased the intracellular lipid content in these cells (Figure 2—figure supplement 5B). However, MIR20B did not have an effect on either the expression of PPARA and its target genes or the intracellular lipid content in PPARA-3’UTR Mut expressing cells (Figure 2—figure supplement 5B-D). Taken together, these results indicate that MIR20B inhibits the expression of PPARA by interacting with its 3’UTR.
The effects of MIR20B are mediated by PPARα
Next, we confirmed the effect of MIR20B on function of PPARα. Since PPARα is a master regulator of lipid metabolism such as FA utilization and oxidation, we investigated the effects of MIR20B on expression of genes involved in lipid metabolism. Transfection of MIR20B into HepG2 cells reduced the expression and activity of PPARα, but co-transfected PPARA expression vector restored them (Figure 3A and B). Overexpression of MIR20B reduced the expression of genes involved in FA β-oxidation and FA uptake, including CPT1A, ACOX1, CD36, and FABP1, but not de novo lipogenesis such as SREBF1 and FASN, which was significantly restored by the forced expression of PPARα (Figure 3C). Next, we tested whether anti-MIR20B enhanced activity of PPARα and the effects of anti-MIR20B was specific to PPARα. Ectopic expression of anti-MIR20B increased the expression and activity of PPARα (Figure 3D and E), and consequently enhanced the expression of genes involved in FA utilization and oxidation (Figure 3F). PPARA-targeting siRNA (siPPARA) suppressed the expression of PPARA and its activity (Figure 3D and E). The increased expression of genes by anti-MIR20B was also suppressed by siPPARA (Figure 3F). In addition, fenofibrate, a PPARα agonist, increased the expression of PPARα and its transcriptional activity in HepG2 cells transfected with MIR20B, but could not restore as much on its own effects (Figure 3G and H). Interestingly, fenofibrate treatment increased the expression of genes involved in FA β-oxidation and FA uptake which are regulated by PPARα, but could not overcome the inhibitory effect of MIR20B (Figure 3I). Taken together, these results indicate that MIR20B affects lipid metabolism through direct inhibition of PPARA.
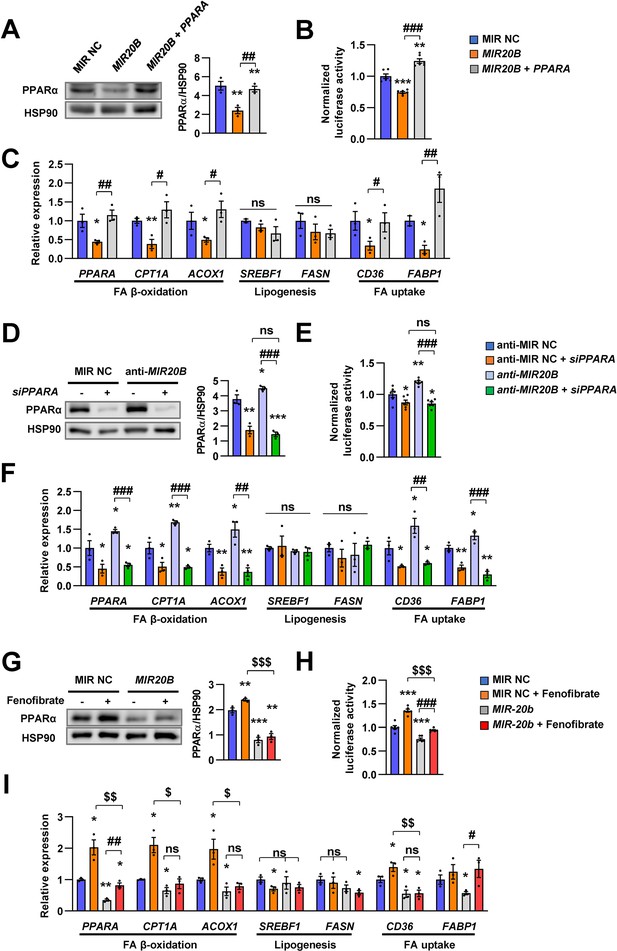
The effects of MIR20B are mediated by PPARα.
HepG2 cells transfected with indicated miRNA, siRNA, or PPARA expression vector. Western blot analysis of PPARα. The intensity of PPARα blot was normalized to that of HSP90 (A, D). Luciferase activity using the luciferase reporter DNA constructs containing PPRE (PPAR response element) was transfected in HepG2 cells. Luciferase activity was normalized to renilla activity (B, E). mRNA level of genes involved in FA β-oxidation, lipogenesis, and FA uptake was analyzed by real-time qPCR (C and F). After transfected with MIR NC or MIR20B, HepG2 cells were treated with fenofibrate (100 µM). Protein level of PPARα was analyzed by western blot and normalized to that of HSP90 (G). The transcriptional activity of PPARα was measured (H). Genes involved in of FA β-oxidation, lipogenesis and FA uptake were determined by real-time qPCR (I). Relative values are normalized to MIR NC. Values represent means ± SEM (n = 3). ns, not significant. *p < 0.05, **p < 0.01, ***p < 0.001 vs MIR NC. #p < 0.05, ##p < 0.01, ###p < 0.001 vs MIR20B or anti-MIR20B. $p < 0.05, $$p < 0.01, $$$p < 0.001 vs MIR NC+ fenofibrate. Raw data can be found in a Source Data file named ‘Figure 3—source data 1’.
-
Figure 3—source data 1
The effects of MIR20B are mediated by PPARα.
- https://cdn.elifesciences.org/articles/70472/elife-70472-fig3-data1-v2.xlsx
MIR20B regulates fatty acid metabolism
We investigated the effects of MIR20B on lipid metabolism to reveal the functional contribution of increased MIR20B to NAFLD. Unlike in HepG2 cells (Figure 2A–C), MIR20B alone did not induce lipid accumulation in primary hepatocytes without OA treatment, but MIR20B significantly increased lipid accumulation in the presence of OA (Figure 4—figure supplement 2A-D). The mRNA levels of genes involved in FA β-oxidation, FA uptake, including CPT1A, ACOX1, CD36, and FABP1, and PPARα target genes were decreased by overexpression of MIR20B compared to the control in HepG2 cells and primary hepatocytes (Figure 4A and B, Figure 4—figure supplement 1A), whereas the genes associated with de novo lipogenesis and ketogenesis were not affected by MIR20B (Figure 4B, Figure 4—figure supplements 2E; Figure 4—figure supplement 3A, B). Interestingly, DGAT1, rate limiting enzyme for TG synthesis, was increased in HepG2 cells upon OA treatment (Figure 4C). MIR20B overexpressed HepG2 cells showed reduced levels of palmitoyl-carnitine, a substrate of β-oxidation, and acetyl-CoA, a product of β-oxidation. Subsequently, TCA cycle intermediate levels, including citrate and succinate, also decreased (Figure 4D).
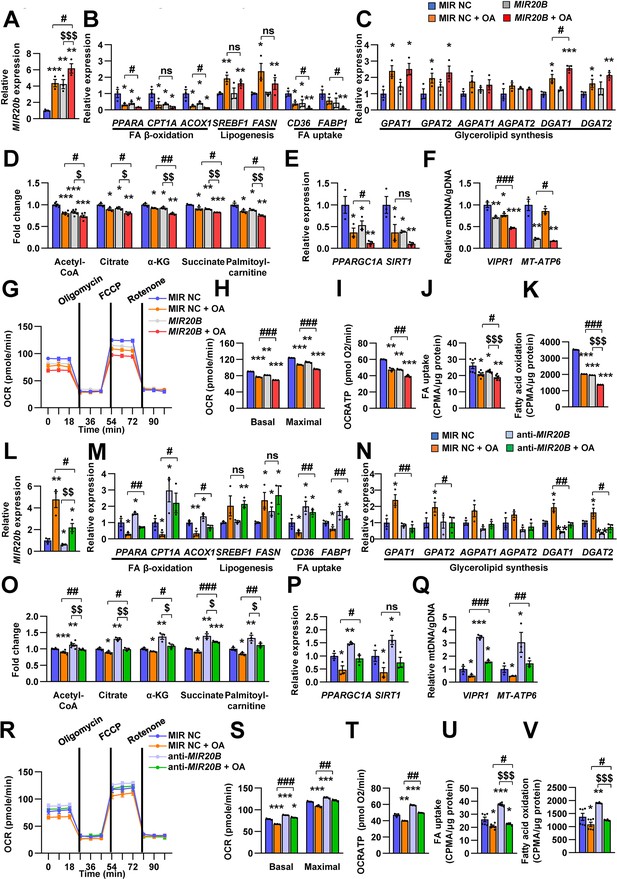
MIR20B regulates fatty acid metabolism.
HepG2 cells were transfected with MIR20B or anti-MIR20B and treated with OA for 24 hr. The expression of MIR20B (A, L) and genes related to FA β-oxidation, lipogenesis, FA uptake (B, M) and glycerolipid synthesis (C, N) were measured by quantitative RT-PCR. Representative mitochondrial metabolites were measured in HepG2 cells (D, O). The expression of genes related to mitochondrial biogenesis (E, P) were measured by quantitative RT-PCR. The mitochondrial copy of VIPR1 and MT-ATP6 were determined (F, Q). OCR (G, R), basal and maximal OCR (H, S), and ATP levels (I, T) were measured in HepG2 cells. FA uptake (J, U) and β-oxidation (K, V) activity were measured using [9,10-3H(N)]-Palmitic Acid and normalized to the total protein content. Relative values are normalized to MIR NC. Values represent means ± SEM (n = 3–5). ns, not significant. *p < 0.05, **p < 0.01, ***p < 0.001 vs MIR NC. #p < 0.05, ##p < 0.01, ###p < 0.001 vs MIR NC+ OA. $p < 0.05, $$p < 0.01, $$$p < 0.001 vs MIR20B or anti-MIR20B, respectively. Raw data can be found in a Source Data file named ‘Figure 4—source data 1’.
-
Figure 4—source data 1
MIR20B regulates fatty acid metabolism.
- https://cdn.elifesciences.org/articles/70472/elife-70472-fig4-data1-v2.xlsx
Enforced expression of MIR20B in HepG2 cells under both basal and OA treatments decreased the expression of PPARGC1A and SIRT1, which are involved in mitochondrial biogenesis (Figure 4E). The copy number of two mtDNA genes, VIPR1 and MT-ATP6, was decreased by MIR20B overexpression following OA treatment (Figure 4F). Consistently, mitochondrial function that was analyzed via OCR (oxygen consumption rate) was reduced by MIR20B under both basal and OA treatment conditions compared to the control (Figure 4G). In particular, the basal respiration and maximal respiratory capacity were significantly suppressed by MIR20B (Figure 4H). Furthermore, the level of ATP production, FA uptake, and FA oxidation was reduced in MIR20B overexpressed cells compared with that in the control under both basal and OA-treated conditions (Figure 4I–K).
To further clarify the role of MIR20B in hepatic fatty acid metabolism, anti-MIR20B was delivered into OA-treated HepG2 cells and primary hepatocytes, and which reduced the expression of MIR20B (Figure 4L, Figure 4—figure supplement 4G). Oil red O staining showed that anti-MIR20B remarkably decreased intracellular lipid accumulation upon OA treatment (Figure 4—figure supplement 4A, D). As expected, MIR20B inhibition reduced the levels of both TG and cholesterol under OA conditions compared to the control (Figure 4—figure supplement 4B, C, E, and F). Lipid consumption-associated genes and PPARα target genes, not de novo lipogenic genes, were significantly upregulated in MIR20B inhibited HepG2 cells and primary hepatocytes compared to those in the control under both basal and OA conditions (Figure 4M, Figure 4—figure supplements 1B; 4H and I). TG synthesis enzymes, such GPAT1 and AGPAT1, were decreased by anti-MIR20B in HepG2 cells under OA-treated condition, however DGAT1 and DGAT2 were reduced under both basal and OA-treated conditions (Figure 4N). Inhibition of MIR20B increased the levels of palmitoyl-carnitine, acetyl-CoA, and TCA cycle intermediates (Figure 4O), whereas ketogenesis was not affected (Figure 4—figure supplement 3). Furthermore, the expression of mitochondrial biogenesis genes (Figure 4P) and the copy number of mitochondrial DNA genes were increased in both basal and OA conditions (Figure 4Q). Consequently, anti-MIR20B treatment significantly upregulated the mitochondrial activity, FA uptake, and FA oxidation (Figure 4R–V). Taken together, these results demonstrated that MIR20B contributes to hepatic lipid accumulation by controlling lipid oxidation, mitochondrial function and TG synthesis through changes in gene expression, further contributing to the progression of NAFLD.
Mir20b promotes hepatic steatosis in HFD-fed mice
To confirm the in vivo roles of Mir20b in obesity model mice, we introduced Mir20b using an adenovirus-associated vector (AAV), referred to as AAV-Mir20b, into C57BL/6 mice that had been fed a normal chow diet (NCD) or a high-fat diet (HFD). Administration of AAV- Mir20b led to high expression levels of Mir20b in the livers of NCD- and HFD-fed mice compared to AAV-Control injection (Figure 5A). However, the expression level of Mir20b was not changed in adipose tissues including white and brown adipose tissues compared to NCD- or HFD-fed AAV-Control injected mice, respectively (Figure 5—figure supplement 1). Consequently, AAV-Mir20b injected mice exhibited a reduction in the expression of Ppara and PPARα target genes compared with AAV-Control injected mice on both NCD and HFD (Figure 5B and R, and Figure 5—figure supplement 2).
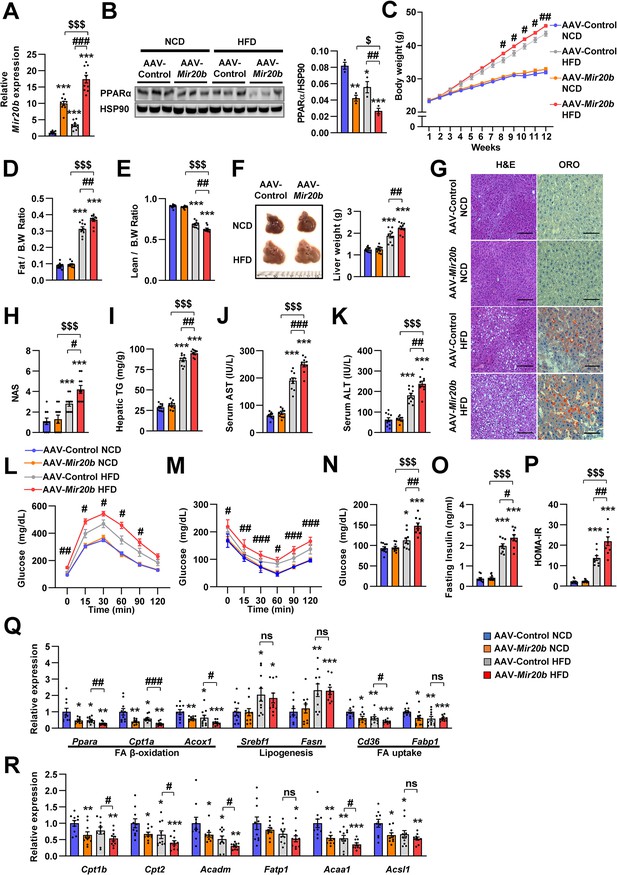
Mir20b promotes hepatic steatosis in HFD-fed mice.
C57BL/6 J mice were fed on normal chow diet (NCD, n = 10 per group) or high fat diet (HFD, n = 10 per group) for 12 weeks and administered with indicated AAVs. Hepatic expression of Mir20b (A) and PPARα (B), body weight (C), the ratio of fat mass to body weight (D), the ratio of lean mass to body weight (E), representative images and weight of liver (F), representative images of H&E staining and Oil Red O staining of liver slides (G), NAFLD activity score (NAS) (H), hepatic TG (I), serum AST (J), serum ALT (K), glucose tolerance (L), insulin tolerance (M), fasting glucose (N), fasting insulin (O), and HOMA-IR (P) were analyzed in indicated mice. Genes related to FA β-oxidation, lipogenesis and FA uptake (Q) and PPARα target genes (R) were determined by quantitative RT-PCR. Relative values are normalized to AAV-Control NCD. The intensity of PPARα blot was normalized to that of HSP90. Values represent means ± SEM (n = 10). ns, not significant. *p < 0.05, **p < 0.01, ***p < 0.001 vs AAV-Control NCD. #p < 0.05, ##p < 0.01, ###p < 0.001 vs AAV-Control HFD. $p < 0.05, $$$p < 0.001 vs AAV-Mir20b NCD. Scale bar is 100 µm. Raw data can be found in a Source Data file named ‘Figure 5—source data 1’.
-
Figure 5—source data 1
Mir20b promotes hepatic steatosis in HFD-fed mice.
- https://cdn.elifesciences.org/articles/70472/elife-70472-fig5-data1-v2.xlsx
Alterations in body weight were not detected in NCD-fed mice after AAV-Mir20b administration; however, AAV-Mir20b led to a significant increase in the body weight of HFD-induced obese mice (Figure 5C). The ratio of fat mass to body weight in AAV-Mir20b administration HFD-fed mice was higher than that in AAV-Control treated mice (Figure 5D and Figure 5—figure supplement 3); however, the ratio of lean mass to body weight was decreased (Figure 5E). Consistently, AAV-Mir20b administration increased liver weight, steatosis, and NAFLD activity score (NAS) in HFD-fed mice (Figure 5F–H). The hepatic TG level, serum activities of aspartate aminotransferase (AST) and alanine aminotransferase (ALT), markers of liver injury, were significantly increased with AAV-Mir20b administration compared with AAV-Control administration in HFD-fed mice (Figure 5I–K).
Additionally, we observed that delivery of AAV-Mir20b to HFD-fed mice significantly impaired glucose tolerance and insulin sensitivity compared to the AAV-Control (Figure 5L and M). Fasting glucose, insulin, and homeostasis model assessment of insulin resistance (HOMA-IR) levels were also increased in AAV-Mir20b administrated HFD-fed mice (Figure 5N–P). We observed that genes involved in FA β-oxidation and FA uptake pathways were downregulated by AAV-Mir20b compared to AAV-Control in both NCD- and HFD-fed mice, whereas lipogenesis genes were not altered in AAV-Mir20b administrated mice (Figure 5Q). These results suggest that Mir20b could aggravate NAFLD by dysregulating lipid metabolism in a HFD-induced obesity model.
Inhibition of Mir20b alleviates hepatic steatosis in HFD-fed mice
Next, we introduced anti-Mir20b into HFD-fed mice. Administration of AAV-anti-Mir20b led to decrease of Mir20b in the livers of NCD- and HFD-fed mice compared to AAV-Control injection (Figure 6A and Figure 6—figure supplement 1). AAV-anti-Mir20b significantly increased expression of Ppara and its target genes in the livers of both NCD- and HFD-fed mice (Figure 6B and R and Figure 6—figure supplement 2). Administration of AAV-anti-Mir20b in HFD-fed mice reduced the body weight compared to that of AAV-Control administrated mice (Figure 6C). We further determined that alterations in body weight were associated with fat mass loss and reduced trend of epididymal white adipose tissue (Figure 6D and Figure 6—figure supplement 3). While the ratio of lean mass to body weight of AAV-anti-Mir20b administrated HFD-fed mice was increased, the lean mass was comparable to that of the control (Figure 6E). We next observed that AAV-anti-Mir20b administration reduced liver weight and hepatic steatosis in HFD-fed mice than in AAV-Control mice (Figure 6F). H&E and Oil Red O staining demonstrated that delivery of AAV-anti-Mir20b significantly attenuated the size and number of lipid droplets in the liver and NAS compared to AAV-Control administration in HFD-fed mice (Figure 6G and H). In accordance with histological changes, metabolic parameters were reduced in AAV-anti-Mir20b administrated mice compared with the AAV-Control administrated mice (Figure 6I–K). Furthermore, AAV-anti-Mir20b significantly improved glucose tolerance (Figure 6L) and insulin sensitivity (Figure 6M) compared to the AAV-Control in HFD-fed mice. Consistently, we determined that fasting glucose, fasting insulin, and HOMA-IR levels were decreased by AAV-anti-Mir20b (Figure 6N–P). Delivery of AAV-anti-Mir20b increased the expression of genes associated with FA β-oxidation and FA uptake compared with the administration of AAV-Control (Figure 6Q). Together, these results suggest that suppression of Mir20b could ameliorate NAFLD by recovering lipid metabolism in a HFD-induced obesity model.
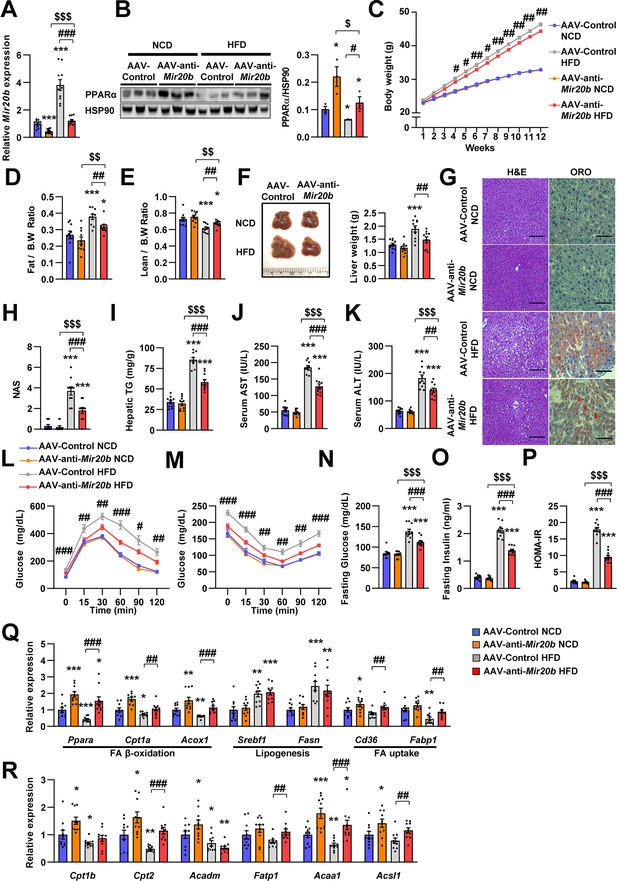
Inhibition of Mir20b alleviates hepatic steatosis in HFD-fed mice.
C57BL/6 J mice were fed on normal chow diet (NCD, n = 10 per group) or high fat diet (HFD, n = 10 per group) for 12 weeks and administered with indicated AAVs. Hepatic expression of Mir20b (A) and PPARα (B) and body weight (C), the ratio of fat mass to body weight (D), the ratio of lean mass to body weight (D), representative images and weight of liver (F), representative images of H&E staining and Oil Red O staining of liver slides (G), NAFLD activity score (NAS) (H), hepatic TG (I), serum AST (J), and serum ALT (K), glucose tolerance (L), insulin tolerance (M), fasting glucose (N), fasting insulin (O), and HOMA-IR (P) were analyzed in indicated mice. Genes related to FA β-oxidation, lipogenesis and FA uptake (Q) and PPARα target genes (R) were determined by quantitative RT-PCR. Relative values are normalized to AAV-Control NCD. The intensity of PPARα blot was normalized to that of HSP90. Values represent means ± SEM (n = 10). *p < 0.05, **p < 0.01, ***p < 0.001 vs AAV-Control NCD. #p < 0.05, ##p < 0.01, ###p < 0.001 vs AAV-Control HFD. $p < 0.05, $$p < 0.01, $$$p < 0.001 vs AAV-anti-Mir20b NCD. Scale bar is 100 µm. Raw data can be found in a Source Data file named ‘Figure 6—source data 1’.
-
Figure 6—source data 1
Inhibition of Mir20b alleviates hepatic steatosis in HFD-fed mice.
- https://cdn.elifesciences.org/articles/70472/elife-70472-fig6-data1-v2.xlsx
The effects of fenofibrate are limited in Mir20b-introduced mice
Next, we tested whether NAFLD treatment with fenofibrate was affected by Mir20b expression in vivo. Administration of AAV-Mir20b led to elevated hepatic levels of Mir20b compared to AAV-Control injection in HFD-fed mice, and the level was slightly decreased by fenofibrate treatment (Figure 7A). Interestingly, we observed that administration of AAV-Control with fenofibrate increased the level of Ppara; however, fenofibrate could not restore the reduced Ppara expression by AAV-Mir20b (Figure 7B). Administration of fenofibrate reduced the body and liver weights of AAV-Control injected mice; however, AAV-Mir20b injected mice exhibited no significant differences by fenofibrate (Figure 7C and D). The ratio of fat to body weight also displayed no alterations between AAV-Mir20b and AAV-Mir20b with fenofibrate (Figure 7E). While the ratio of lean mass to body weight was increased by fenofibrate in AAV-Mir20b injected mice, the lean mass was comparable (Figure 7F). H&E staining, Oil Red O staining, NAS and hepatic TG levels demonstrated that fenofibrate significantly attenuated lipid accumulation in the liver of HFD-fed mice, but the effect of fenofibrate was suppressed by AAV-Mir20b (Figure 7G–I). Serum AST and ALT levels were decreased by fenofibrate, but this benefit was did not detected in AAV-Mir20b injected mice (Figure 7J and K). We further observed that blood glucose tolerance and insulin sensitivity were improved by fenofibrate; however, AAV-Mir20b offset the improvement by fenofibrate (Figure 7L and M). Fasting glucose, fasting insulin, and HOMA-IR levels were markedly decreased by fenofibrate in HFD-fed mice (Figure 7N–P). In AAV-Mir20b injected mice, fenofibrate did not reduce fasting insulin levels, but decreased fasting glucose and HOMA-IR levels. Fenofibrate also did not restore the suppressed expression of genes regulating FA β-oxidation in AAV-Mir20b-injected mice (Figure 7Q). Taken together, the effect of fenofibrate to ameliorate NAFLD-like symptoms was limited in AAV-Mir20b administrated HFD-fed mice because of the targeting PPARα by Mir20b.
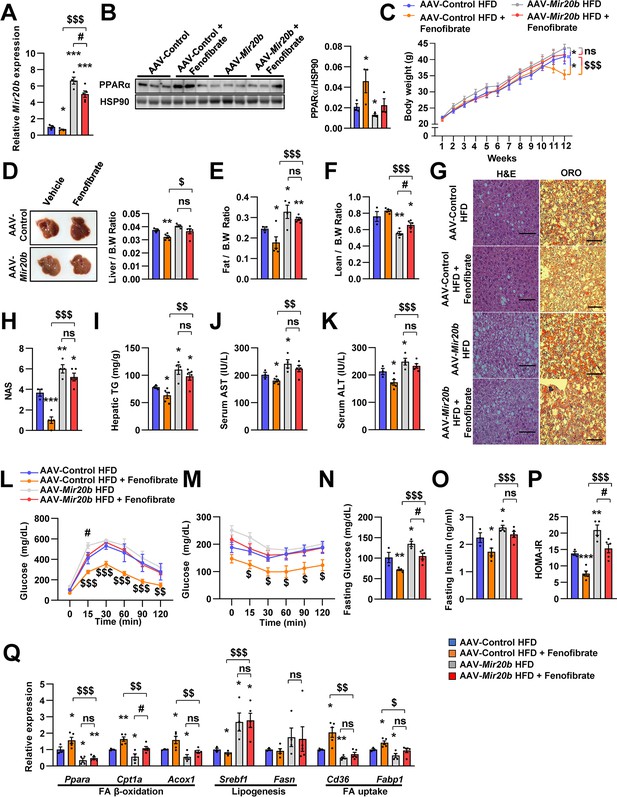
The effects of fenofibrate are limited in Mir20b-introduced mice.
C57BL/6 J mice were fed on high fat diet (HFD) for 12 weeks and administered with indicated AAVs (n = 3–5 per group). Then, mice injected with vehicle or fenofibrate (100 mg/kg) for 4 weeks. Hepatic Mir20b (A) and PPARα expression (B), body weight (C), representative images and weight of liver (D), the ratio of fat mass to body weight (E), the ratio of lean mass to body weight (F), representative images of H&E staining and Oil Red O staining of liver slides (G), NAFLD activity score (NAS) (H), hepatic TG (I), serum AST (J), and serum ALT (K), glucose tolerance (L), insulin tolerance (M), fasting glucose (N), fasting insulin (O), and HOMA-IR (P) were analyzed in indicated mice. Genes related to FA β-oxidation, lipogenesis and FA uptake were determined by quantitative RT-PCR (Q). Relative values are normalized to AAV-Control HFD. The intensity of PPARα blot was normalized to that of HSP90. The intensity of PPARα blot was normalized to that of HSP90. Values represent means ± SEM (n = 3–5). ns, not significant, *p < 0.05, **p < 0.01, ***p < 0.001 vs AAV-Control HFD. #p < 0.05 vs AAV-Mir20b HFD. $p < 0.05, $$p < 0.01, $$$p < 0.001 vs AAV-Control HFD+ fenofibrate. Scale bar is 100 µm. Raw data can be found in a Source Data file named ‘Figure 7—source data 1’.
-
Figure 7—source data 1
The effects of fenofibrate are limited in Mir20b-introduced mice.
- https://cdn.elifesciences.org/articles/70472/elife-70472-fig7-data1-v2.xlsx
Mir20b promotes liver inflammation and fibrosis in MCD-fed mice
We further demonstrated that RNA-seq data have a significant correlation of fold change values with previously published RNA-seq data under both NASH and liver fibrosis conditions within the NR transcription (Hoang et al., 2019 Figure 8A and B). This implies that MIR20B is able to set up an NR transcription program similar to that of NASH and liver fibrosis. To test this hypothesis, AAV-Control or AAV-anti-Mir20b was administered to C57BL/6 mice placed on a methionine/choline-deficient diet (MCD), which is the most widely used diet to induce NAFLD/NASH. Administration of AAV-anti-Mir20b led to decrease of Mir20b in the livers of NCD- and MCD-fed mice compared to AAV-Control injection (Figure 8C). We observed that the expression of Ppara was increased in MCD-fed mice and administration of AAV-anti-Mir20b displayed an elevation of PPARα, both at the mRNA and protein levels (Figure 8D–F). We next observed that AAV-anti-Mir20b administration significantly reduced hepatic steatosis in MCD-fed mice than in AAV-Control mice (Figure 8G). Liver sections clearly showed a decrease in both steatosis and fibrosis with AAV-anti-Mir20b administration in MCD-fed mice (Figure 8H–J). Consistently, AAV-anti-Mir20b administration decreased the levels of hepatic TG, AST, and ALT activity compared to AAV-Control injection (Figure 8K–M). Moreover, AAV-anti-Mir20b significantly reduced the expression of genes related to hepatic inflammation, including Tnf and Il6 (Figure 8N), and fibrosis, including the NASH-relevant genes, such as Acta2, Col1a1, Col3a1 and Timp1 (Figure 8O), in MCD-fed mice. Taken together, these results indicate that Mir20b plays an important role in the development of fibrosis, inflammation, and hepatic steatosis in NAFLD progression.
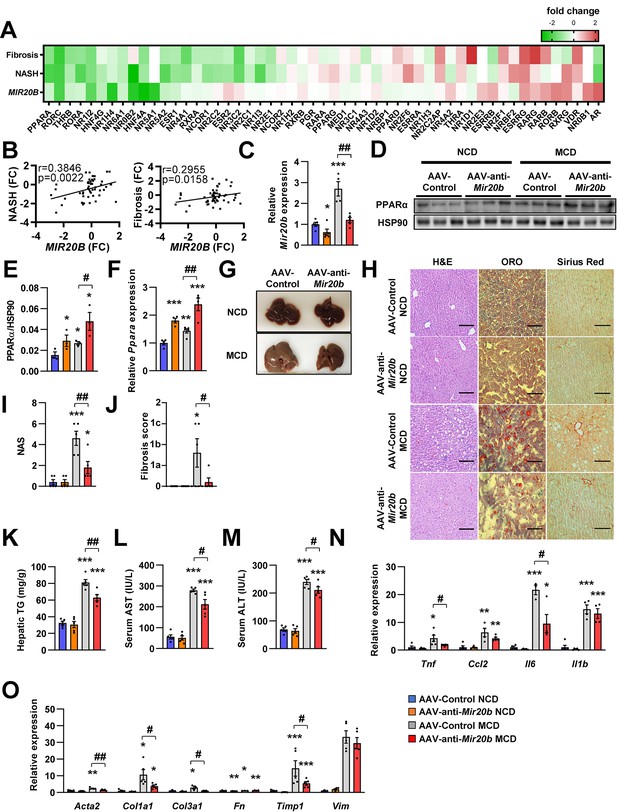
Mir20b promotes liver inflammation and fibrosis in MCD-fed mice.
Heatmap (A) and correlation (B) of hepatic nuclear receptor gene expression in RNA-seq (Figure 2) with public databases of liver fibrosis or NASH patients. The values are fold change (FC) compared to each control samples. C57BL/6 J mice were fed on normal chow diet (NCD, n = 5 per group) or methionine-deficient diet (MCD, n = 5 per group) for 4 weeks and administered with indicated AAVs. Before 1 week of MCD challenge, mice were injected with AAV-Control or AAV-anti-Mir20b. Hepatic Mir20b (C) and PPARα expression (D, E, F), representative images of liver (G), H&E staining, Oil Red O, staining and Sirius Red staining of liver slides (H), NAFLD activity score (NAS) (I), fibrosis score (J), hepatic TG (K), serum AST (L), and serum ALT (M) were analyzed in indicated mice. Genes related to inflammation (N) and fibrosis (O) were determined by quantitative RT-PCR. Relative values are normalized to AAV-Control NCD. The intensity of PPARα blot was normalized to that of HSP90. Values represent means ± SEM (n = 5). *p < 0.05, **p < 0.01, ***p < 0.001 vs AAV-Control NCD. #p < 0.05, ##p < 0.01 vs AAV-Control MCD. Scale bar is 100 µm. Raw data can be found in a Source Data file named ‘Figure 8—source data 1’.
-
Figure 8—source data 1
Mir20b promotes liver inflammation and fibrosis in MCD-Fed Mice.
- https://cdn.elifesciences.org/articles/70472/elife-70472-fig8-data1-v2.xlsx
Anti-Mir20b enhances efficacy of fenofibrate in MCD-fed mice
Recently, drug development strategies for NAFLD/NASH are moving toward combination therapies (Dufour et al., 2020). However, the efficacy of developing drugs, including fenofibrate, against NAFLD/NASH is limited (Fernández-Miranda et al., 2008). Thus, we tested whether the combination of AAV-anti-Mir20b and fenofibrate would improve NAFLD in MCD-fed mice. The levels of hepatic Mir20b were reduced after administration of AAV-anti-Mir20b in MCD-fed mice compared to those in mice administered with AAV-Control, and this reduction was also observed after fenofibrate treatment (Figure 9A). Interestingly, the combination of AAV-anti-Mir20b and fenofibrate increased the levels of Ppara to a greater extent than AAV-anti-Mir20b alone (Figure 9B and C). AAV-anti-Mir20b or fenofibrate administration significantly reduced the liver weight and hepatic TG levels, and co-administration further reduced hepatic steatosis (Figure 9D and E). Histological sections showed that the combination of AAV-anti-Mir20b and fenofibrate improved NAFLD, as evidenced by the effects on both lipid accumulation and fibrosis in the liver (Figure 9F–H). Consistently, the levels of AST and ALT were significantly lower after combined treatment with AAV-anti-Mir20b and fenofibrate than after a single treatment (Figure 9I and J). In addition, the expression of genes related to hepatic inflammation, such as Tnf and Il6 (Figure 9K), and fibrosis, such as Acta2, Col1a1, Fn, and Timp1, (Figure 9L), was further decreased by the combination of AAV-anti-Mir20b and fenofibrate. These results suggest that AAV-anti-Mir20b may increase the efficacy of fenofibrate, especially its effect on fibrosis, and provide a more effective option for improving NAFLD/NASH.
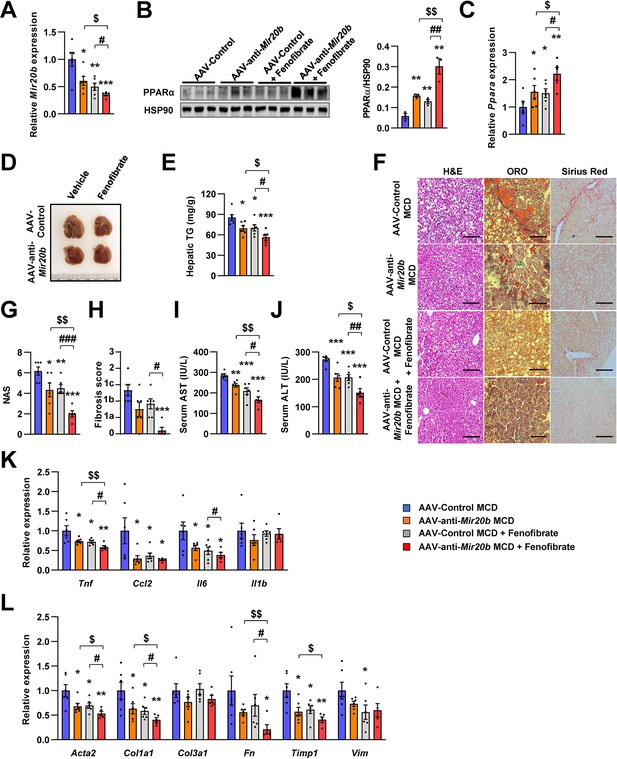
Inhibition of Mir20b with fenofibrate ameliorates liver inflammation and fibrosis in MCD-fed mice.
C57BL/6 J were fed a methionine-deficient diet (MCD) for 4 weeks with administration of indicated AAVs (n = 5–6 per group). Then, mice injected with vehicle or fenofibrate (100 mg/kg) for 4 weeks. Hepatic Mir20b (A) and PPARA expression (B, C). Representative images of liver (D), hepatic TG (E), H&E staining, Oil Red O staining, Sirius Red staining of liver slides (F), NAFLD activity score (NAS) (G), fibrosis score (H), serum AST (I), and serum ALT (J) were analyzed in indicated mice. Genes related to inflammation (K) and fibrosis (L) were determined by quantitative RT-PCR. Relative values are normalized to AAV-Control MCD. The intensity of PPARα blot was normalized to that of HSP90. Values represent means ± SEM (n = 5–6). *p < 0.05, **p < 0.01, ***p < 0.001 vs AAV-Control MCD. #p < 0.05, ##p < 0.01, ###p < 0.001 vs AAV-Control MCD+ fenofibrate. $p < 0.05, $$p < 0.01 vs AAV-anti-Mir20b MCD. Scale bar is 100 µm. Raw data can be found in a Source Data file named ‘Figure 9—source data 1’.
-
Figure 9—source data 1
Inhibition of Mir20b with fenofibrate ameliorates liver inflammation and fibrosis in MCD-fed mice.
- https://cdn.elifesciences.org/articles/70472/elife-70472-fig9-data1-v2.xlsx
Discussion
Obesity has been widely demonstrated to be central to the pathogenesis of NAFLD. Among other peripheral tissues, the liver plays a dominant role in the regulation of lipid homeostasis (Pawlak et al., 2015). Although abnormal regulation of metabolic homeostasis in the liver has been recognized in diabetes and NAFLD, the underlying molecular mechanisms remain to be elucidated. Growing evidence has demonstrated that MIR20B levels are significantly upregulated in the plasma miRNA profiles of NAFLD patients (Jin et al., 2012). Moreover, plasma MIR20B levels were highly elevated in T2DM/NAFLD patients compared to those in T2DM patients (Ye et al., 2018). However, the molecular mechanism through which MIR20B regulates NAFLD progression remains unknown. In this study, we demonstrated that MIR20B promotes NAFLD progression by modulating lipid metabolism, including FA β-oxidation, FA uptake, and TG synthesis, as well as ATP production by mitochondrial biogenesis. Our data clearly showed the regulatory mechanism of PPARα by MIR20B, and MIR20B may serve as a novel biological marker in NAFLD.
Decreased PPARα is contributed to development of NAFLD (Francque et al., 2015). A few miRNAs were reported to regulate PPARA expression in NAFLD. miR-34a targets PPARA and SIRT1, associating with FA oxidation and cholesterol synthesis. However, the effect of MIR34A on inflammation and fibrosis is not clear (Ding et al., 2015). MIR21 also decreases the expression of PPARA in NASH, however, activated PPARα by MIR21 suppression reduces inflammation, liver injury, and fibrosis without improvement in FA β-oxidation and lipid accumulation (Loyer et al., 2016). In the present study, we demonstrate the novel miRNA which has different mode of action. MIR20B showed the deteriorating effects on FA oxidation, steatosis, inflammation, and fibrosis in HFD- or MCD-fed mice. How these miRNAs targeting PPARA have different regulatory mechanisms should be further studied.
A previous study demonstrated that upregulated MIR20B levels in obesity-induced metabolic disorders such as T2DM were considered to prevent several targets, such as STAT3, CD36, and PTEN, which are involved in glucose and lipid homeostasis. The MIR20B/STAT axis, which is involved in the insulin signaling pathway, alters glycogen synthesis in human skeletal muscle cells (Katayama et al., 2019). Moreover, MIR20B directly targets PTEN involved in PI3K/Akt/mTOR signaling pathway that modulates glucose metabolism in gastric cells (Streleckiene et al., 2020). Hosui et al., 2017 proposed a model in which the fatty acid transporter CD36 is also a potential target of MIR20B, which crucially regulates hepatic lipid metabolism in STAT5 KO mice models. But, it has been reported that CD36 and FABP1 are direct PPARα target genes (Rakhshandehroo et al., 2010). In this study, we observed that MIR20B downregulated PPARA and suppressed the expression of CD36 and FABP1. Thus, PPARA is the primary target of MIR20B in regulating hepatic lipid metabolism.
MIR20B was induced by OA, but FA uptake was decreased by overexpression of MIR20B, and was accompanied by a considerable decrease in CD36 expression (Figure 4B and J). However, other lipid transporters such as FATPs, except for PPARα target FATP1, were not significantly altered (Figure 4—figure supplements 1 and 5), suggesting that FA uptake is continued by these transporters. The expression of CD36 is relatively low in normal hepatocytes, and the molecule may not be the primary fatty acid transporter in these cells (Wilson et al., 2016). Furthermore, the decrease in FA uptake upon CD36 KO is modest even during a HFD (Wilson et al., 2016). In addition, we observed that the expression of MIR20B is induced and increased for up to 24 hr by OA treatment (Figure 4—figure supplement 6). This is followed by a slight decrease, remaining at a constant elevated level. Together, the findings indicated that other fatty acid transporters contributing to FA uptake account for the entry of OA into cells and MIR20B induction.
Hepatic steatosis could be affected by adipose tissue through FFA release and hepatic uptake of circulating FFAs (Rasineni et al., 2021). Our results showed that the epididymal adipose tissue of HFD-fed mice was enlarged upon AAV-Mir20b treatment; however, the serum FFA levels in these mice were comparable to those in mice treated with the AAV-Control (Figure 5—figure supplement 4B). Of note, the expression of genes related to lipolysis did not change in adipose tissues, and that of hepatic FA transporter, CD36, was decreased by AAV-Mir20b treatment (Figure 5Q and Figure 5—figure supplement 4A). In addition, excess hepatic TGs are secreted as very low-density lipoproteins (VLDLs), and the secretion rate increases with the TG level (Fabbrini et al., 2008). VLDLs deliver TGs from the liver to adipose tissue and contributes expansion of adipose tissue (Chiba et al., 2003). Together, these reports suggest that adipose tissue is also remodeled by the liver in HFD-fed mice and patients with NAFLD. Therefore, the increase of hepatic TGs by AAV-Mir20b is unlikely affected by AAV-Mir20b on epididymal adipose tissue, and the increase in fat content (Figure 5—figure supplement 3) may be a consequence of increased hepatic TG levels.
The expression of glycerolipid synthetic genes, including AGPATs, GPATs, and DGATs, was increased by OA treatment, but MIR20B overexpression did not influence the expression of these genes except for that of DGAT1. However, treatment with anti-MIR20B significantly reduced the expression of glycerolipid synthetic genes, including GPATs and DGATs, under OA treatment (Figure 4C and N). These results suggested that MIR20B is necessary but not sufficient to induce the expression of glycerolipid synthetic genes under OA treatment. We have shown that OA induces the expression of MIR20B (Figure 1C and D), which can explain why MIR20B overexpression did not show an additional enhancement under OA treatment. The increase in DGAT1 expression induced by MIR20B might contribute to the increase in TG formation and capacity to store OA. This could change the flux of oleyl-CoA to TG synthesis, not β-oxidation with reduced expression of lipid oxidation-associated genes (Figure 4B). Thus, we can expect that the decrease in OA uptake and increase in TG formation induced by MIR20B resulted in reduced amounts of OA or oleyl-CoA inside the cell. However, as lipid consumption through FA oxidation is decreased by MIR20B, free OA or oleyl-CoA might be maintained at a stably increased level compared to that of OA-untreated MIR NC or MIR20B condition, and the impact of the changes in OA or oleyl-CoA levels on the transcriptional phenotype might not be significant as found in a constant elevated level of MIR20B by OA.
Recent reports suggest that some transcription factors regulate metabolic homeostasis by directly mediating the expression of miRNAs (Yang and Wang, 2011). E2F1, which is a member of the E2F transcription factor family, regulates myoblast differentiation and proliferation via the auto-regulatory feedback loop between E2F1 and Mir20b in muscle (Luo et al., 2016). Both the hepatic expression and activity of E2F1 are increased during obesity. E2f1 deficiency protects against obesity- and diabetes-induced liver steatosis in mouse models (Zhang et al., 2014). Additionally, E2F1-induced chronic inflammation and hepatic lipid metabolism during NAFLD development (Denechaud et al., 2016). Consistent with these results, we observed that the expression of E2F1 was significantly increased in the fatty liver of both mice and humans, and its expression was positively correlated with that of MIR20B (Figure 10). These results suggest that E2F1 may be an upstream regulator of MIR20B in the liver, and this upregulation of MIR20B regulates lipid metabolism in the pathogenesis of NAFLD.
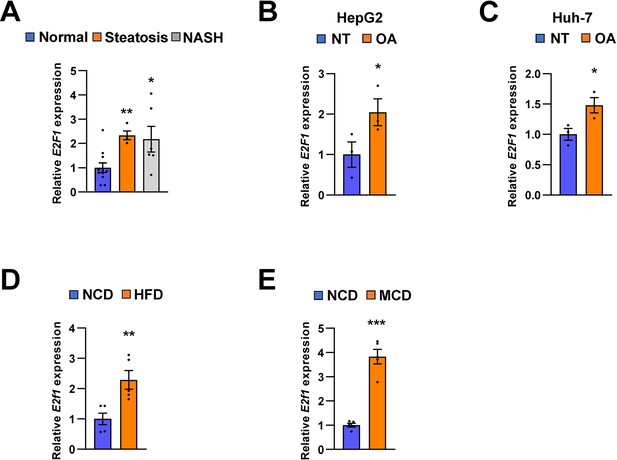
E2F1 is upregulated in both NAFLD patients and mice model.
The expression of E2F1 was analyzed by quantitative RT-PCR. Hepatic E2F1 expression levels of steatosis or NASH patients were normalized to those of normal patients. *p < 0.05 and **p < 0.01 vs normal patients (A). E2F1 expression levels from HepG2 cells (B) and Huh-7 cells (C) treated with OA for 24 hours were normalized to no treatment (NT). Hepatic E2f1 expression levels from C57BL/6 J mice fed a HFD (D) and a MCD (E) were normalized to NCD. Values represent means ± SEM (n = 3–11). *p < 0.05, **p < 0.01, ***p < 0.001 vs NT in cells or NCD-fed mice, respectively. Raw data can be found in a Source Data file named ‘Figure 10—source data 1’.
-
Figure 10—source data 1
E2F1 is upregulated in both NAFLD patients and mice model.
- https://cdn.elifesciences.org/articles/70472/elife-70472-fig10-data1-v2.xlsx
NAFLD patients can develop NASH, which is characterized by hepatic steatosis complicated by chronic hepatocellular damage and severe inflammation with fibrosis, potentially developing into cirrhosis and HCC (Corey and Kaplan, 2014). This indicates that suppression of NAFLD progression is the primary option for preventing the development of HCC. Recent reports suggest that Ppara KO mice fed an MCD developed much more severe NASH than wild-type mice, and the expression of Ppara in HCC tissue was significantly lower than that in normal liver tissue (Montagner et al., 2016). PPARα activation contributes to the inhibition of HCC cell proliferation Thus, hepatic PPARα plays a crucial role in tumorigenesis in the liver (Lefebvre et al., 2006). Interestingly, it has been reported that upregulated Mir20b highly regulates cancer cell proliferation and promotes proliferation of H22 hepatocellular carcinoma cells (Peng et al., 2019; Xia et al., 2020). Thus, plasma MIR20B can be a promising target in liver cancer development. Indeed, we observed that the level of MIR20B was increased in NAFLD patients, but even robustly increased in the NASH stage. Furthermore, we observed that the hepatic function of Mir20b dramatically regulates the genes involved in inflammation and fibrosis by directly repressing Ppara in MCD-fed mice. Thus, our study strongly suggested that MIR20B regulates the pathogenesis of NAFLD, but might also be relevant in the development of severe stages of liver fibrosis and even in HCC.
Fibrates are FDA-approved medications for the treatment of patients with dyslipidemia (hypercholesterolemia, mixed dyslipidemia, hypertriglyceridemia) (Katsiki et al., 2013). Fibrates are well-known PPARα agonists, and PPARα is a widely accepted promising target for the treatment of NAFLD and NASH. In animal studies, fenofibrate improved hypertriglyceridemia and hepatic steatosis. In a small pilot trail, fenofibrate improved ALT and AST levels and hepatocellular ballooning degeneration, but did not show significant improvements in steatosis, lobular inflammation, fibrosis, or NAS score (Fernández-Miranda et al., 2008). Clofibrate, another PPARα agonist, did not show clinical benefit in the treatment of NASH (Laurin et al., 1996). Recently, the effect of omega-3 poly-unsaturated FAs (n-3 PUFA), which are ligands for PPARα, on patients with NAFLD was evaluated. Omacor, which consists of purified docosahexaenoic and eicosapentaenoic acids as ethyl esters, showed a linear decrease in liver fat percentage without improvements in fibrosis score (Scorletti et al., 2014). Omacor has undergone a phase three clinical trial but the results have not been disclosed (NCT01277237). These results show that the effect of PPARα agonists on NAFLD is limited. Our results suggest one of the possible reasons for this limited effect. Here, we show that PPARA expression is suppressed by increased MIR20B expression, which may weaken the efficacy of fibrates. Indeed, our results showed that the effects of fenofibrate were limited in AAV-Mir20b-treated mice, and that the combination of fenofibrate and AAV-anti-Mir20b further improved the NAFLD phenotype in MCD-fed mice. Nowadays, it is generally accepted that treatment with PPARα agonists alone is limited and that a combination strategy is necessary for NAFLD. The inhibition of MIR20B presents an opportunity for the development of novel therapeutics for NAFLD.
Data availability
Sequencing data have been deposited in GEO under accession codes GSE168484. Other data generated or analysed during this study are included in the manuscript. Source data files have been provided.
-
NCBI Gene Expression OmnibusID GSE168484. Hepatic miR20b promotes nonalcholic fatty liver diseases by targeting PPARα.
-
NCBI Gene Expression OmnibusID GSE130970. Gene expression predicts histological severity and reveals distinct molecular profiles of nonalcoholic fatty liver disease.
References
-
Comprehensive analysis of normal adjacent to tumor transcriptomesNature Communications 8:1077.https://doi.org/10.1038/s41467-017-01027-z
-
Nonalcoholic steatohepatitis: a proposal for grading and staging the histological lesionsThe American Journal of Gastroenterology 94:2467–2474.https://doi.org/10.1111/j.1572-0241.1999.01377.x
-
VLDL induces adipocyte differentiation in ApoE-dependent mannerArteriosclerosis, Thrombosis, and Vascular Biology 23:1423–1429.https://doi.org/10.1161/01.ATV.0000085040.58340.36
-
Nonalcoholic Fatty Liver Disease Is Associated with Benign Prostate HyperplasiaJournal of Korean Medical Science 35:e164.https://doi.org/10.3346/jkms.2020.35.e164
-
Obesity and liver disease: the epidemic of the twenty-first centuryClinics in Liver Disease 18:1–18.https://doi.org/10.1016/j.cld.2013.09.019
-
Non-alcoholic fatty liver disease: the mist gradually clearsJournal of Hepatology 48 Suppl 1:S104–S112.https://doi.org/10.1016/j.jhep.2008.01.009
-
E2F1 mediates sustained lipogenesis and contributes to hepatic steatosisThe Journal of Clinical Investigation 126:137–150.https://doi.org/10.1172/JCI81542
-
A pilot trial of fenofibrate for the treatment of non-alcoholic fatty liver diseaseDigestive and Liver Disease 40:200–205.https://doi.org/10.1016/j.dld.2007.10.002
-
The reactome pathway knowledgebaseNucleic Acids Research 48:D498–D503.https://doi.org/10.1093/nar/gkz1031
-
Transition from hepatic steatosis to steatohepatitis: unique microRNA patterns and potential downstream functions and pathwaysJournal of Gastroenterology and Hepatology 27:331–340.https://doi.org/10.1111/j.1440-1746.2011.06864.x
-
KEGG: new perspectives on genomes, pathways, diseases and drugsNucleic Acids Research 45:D353–D361.https://doi.org/10.1093/nar/gkw1092
-
The role of fibrate treatment in dyslipidemia: an overviewCurrent Pharmaceutical Design 19:3124–3131.https://doi.org/10.2174/1381612811319170020
-
Sorting out the roles of PPAR alpha in energy metabolism and vascular homeostasisThe Journal of Clinical Investigation 116:571–580.https://doi.org/10.1172/JCI27989
-
Wedelolactone inhibits adipogenesis through the ERK pathway in human adipose tissue-derived mesenchymal stem cellsJournal of Cellular Biochemistry 113:3436–3445.https://doi.org/10.1002/jcb.24220
-
Nuclear receptors in nonalcoholic Fatty liver diseaseJournal of Lipids 2012:139875.https://doi.org/10.1155/2012/139875
-
WikiPathways: connecting communitiesNucleic Acids Research 49:D613–D621.https://doi.org/10.1093/nar/gkaa1024
-
Minireview: Evolution of NURSA, the Nuclear Receptor Signaling AtlasMolecular Endocrinology 23:740–746.https://doi.org/10.1210/me.2009-0135
-
Regulation of BTG3 by microRNA-20b-5p in non-small cell lung cancerOncology Letters 18:137–144.https://doi.org/10.3892/ol.2019.10333
-
Peroxisome proliferator-activated receptor alpha target genesPPAR Research 2010:612089.https://doi.org/10.1155/2010/612089
Article and author information
Author details
Funding
National Research Foundation of Korea (2020R1F1A1061267)
- Jang Hyun Choi
National Research Foundation of Korea (2018R1A5A1024340)
- Jang Hyun Choi
National Research Foundation of Korea (NRF-2021R1I1A2041463)
- Jang Hyun Choi
National Research Foundation of Korea (2020R1I1A1A01074940)
- Hyun-Jun Jang
Korea Mouse Phenotyping Project (2016M3A9D5A01952411)
- Jang Hyun Choi
Future-leading Project Research Fund (1.210034.01)
- Jang Hyun Choi
National Research Foundation of Korea (2016M3C9A394589324)
- Dougu Nam
The funders had no role in study design, data collection and interpretation, or the decision to submit the work for publication.
Acknowledgements
This research was funded by Korea Mouse Phenotyping Project (2016M3A9D5A01952411), the National Research Foundation of Korea (NRF) grant funded by the Korea government (2020R1F1A1061267, 2018R1A5A1024340, NRF-2021R1I1A2041463), the Future-leading Project Research Fund (1.210034.01) of UNIST to J.H.C., the National Research Foundation of Korea (NRF) grant funded by the Korea government (2020R1I1A1A01074940) to H-J.J., and the National Research Foundation of Korea (NRF) grant funded by the Korea government (2016M3C9A394589324) to D.N.
Ethics
Human subjects: Human liver tissue samples of 21 patients were acquired from the BioResource Center (BRC) of Asan Medical Center, Seoul, Republic of Korea. The process of 21 human tissue samples was officially approved by the Institutional Review Board of Asan Medical Center (IRB approval number: 2018-1512).
All animal experiments were performed according to procedures approved by the Ulsan National Institute of Science and Technology's Institutional Animal Care and Use Committee (UNISTIACUC-19-04).
Copyright
This is an open-access article, free of all copyright, and may be freely reproduced, distributed, transmitted, modified, built upon, or otherwise used by anyone for any lawful purpose. The work is made available under the Creative Commons CC0 public domain dedication.
Metrics
-
- 2,380
- views
-
- 519
- downloads
-
- 36
- citations
Views, downloads and citations are aggregated across all versions of this paper published by eLife.
Citations by DOI
-
- 36
- citations for umbrella DOI https://doi.org/10.7554/eLife.70472