An atrial fibrillation-associated regulatory region modulates cardiac Tbx5 levels and arrhythmia susceptibility
Abstract
Heart development and rhythm control are highly Tbx5 dosage-sensitive. TBX5 haploinsufficiency causes congenital conduction disorders, whereas increased expression levels of TBX5 in human heart samples has been associated with atrial fibrillation (AF). We deleted the conserved mouse orthologues of two independent AF-associated genomic regions in the Tbx5 locus, one intronic (RE(int)) and one downstream (RE(down)) of Tbx5. In both lines, we observed a modest (30%) increase of Tbx5 in the postnatal atria. To gain insight into the effects of slight dosage increase in vivo, we investigated the atrial transcriptional, epigenetic and electrophysiological properties of both lines. Increased atrial Tbx5 expression was associated with induction of genes involved in development, ion transport and conduction, with increased susceptibility to atrial arrhythmias, and increased action potential duration of atrial cardiomyocytes. We identified an AF-associated variant in the human RE(int) that increases its transcriptional activity. Expression of the AF-associated transcription factor Prrx1 was induced in Tbx5RE(int)KO cardiomyocytes. We found that some of the transcriptional and functional changes in the atria caused by increased Tbx5 expression were normalized when reducing cardiac Prrx1 expression in Tbx5RE(int)KO mice, indicating an interaction between these two AF genes. We conclude that modest increases in expression of dose-dependent transcription factors, caused by common regulatory variants, significantly impact on the cardiac gene regulatory network and disease susceptibility.
Editor's evaluation
Molecular mechanisms of atrial fibrillation, a highly prevalent arrhythmia, have been challenging to contextualize. The investigators, in a series of experiments using the deletion of two loci in TBX5 (one intronic and one downstream), achieved a modest increase in Tbx5 in the atria. In a series of elegant experiments they show that this increase in TbX5 in turn impacted the cardiac gene regulatory network that resulted in a higher susceptibility to AF. These results will provide useful insights into the mechanisms of disease especially the variable phenotypes observed with functional variants of a gene.
https://doi.org/10.7554/eLife.80317.sa0Introduction
The lifetime risk of developing a common disease, such as cardiovascular or neurodegenerative conditions, is influenced by genetic predisposition resulting from large numbers of inherited common genetic variants (single-nucleotide polymorphisms, SNPs). Disease-associated variants are typically found in noncoding genomic regions and are thought to affect the functionality of regulatory elements (REs) such as enhancers or elements involved in chromatin conformation (Degtyareva et al., 2021; Deplancke et al., 2016; Hormozdiari et al., 2018; Maurano et al., 2012; Schaub et al., 2012). These regulatory variants are pleiotropic and their effects on target gene expression are often specific to particular cell-types, conditions or stages of development (GTEx Consortium, 2020; Sobreira et al., 2021; Strober et al., 2019; Watanabe et al., 2019). It remains challenging to identify the causal variants among the many associated variants in a disease-associated noncoding DNA region and the REs that are affected by such variants (Hormozdiari et al., 2017). Moreover, common variants typically have a small effect on phenotype, and the different functional variants may act additively, synergistically, or oppositely. As a consequence, very few biological mechanisms linking disease-associated variant(s) to phenotype have been uncovered (Lappalainen and MacArthur, 2021; Timpson et al., 2018; Visscher et al., 2017). Here, we set out to investigate how particular noncoding regions harboring clustered variants associated with a common disease modulate expression of a disease-associated transcription factor gene in a tissue-specific manner, and how this expression change affects phenotype in vivo.
Genome-wide association studies (GWAS) have identified many common variants in over 100 genetic loci associated with atrial fibrillation (AF) risk, the most prevalent arrhythmia associated with high comorbidity and increased mortality risk (Christophersen et al., 2017; Chugh et al., 2014; Low et al., 2017; Nielsen et al., 2018; Roselli et al., 2018; Staerk et al., 2017). The identification of functional variants, REs, and target genes underlying AF will provide important insights into the molecular mechanisms of disease (van Ouwerkerk et al., 2020). AF-associated variants have been identified in loci harboring transcription factor-encoding genes, including PITX2, TBX5, and PRRX1, suggesting that altered expression levels of such factors cause imbalances in gene regulatory networks that control heart rhythm and function (van Ouwerkerk et al., 2020). Indeed, using mouse models, insufficiency of these transcriptional regulators was shown to cause arrhythmia susceptibility (Bosada et al., 2021; Dai et al., 2019; Kirchhof et al., 2011; Laforest et al., 2019; Nadadur et al., 2016; Tao et al., 2014; Tucker et al., 2017; Wang et al., 2010; Zhang et al., 2019). Heterozygous loss- or gain of function variants in TBX5 can cause Holt-Oram syndrome in humans, characterized by congenital heart defects and cardiac conduction anomalies, as a result of profound changes in the gene regulatory networks controlling heart development and function (Basson et al., 1997; Bruneau et al., 1999; Kathiriya et al., 2021; Li et al., 1997; Mori et al., 2006; Moskowitz et al., 2004). Interestingly, duplications of TBX5 as well as intragenic duplications have been reported in families with (atypical) Holt-Oram syndrome including cardiac defects (Cenni et al., 2021; Kimura et al., 2015; Patel et al., 2012). Furthermore, a gain-of-function pathological missense variant in TBX5 causes paroxysmal AF (Postma et al., 2008; van Ouwerkerk et al., 2022). Moreover, a previous study uncovered a 30% increase, rather than a reduction, in cardiac TBX5 expression in human heart tissues has been associated with AF (Roselli et al., 2018). The effects of small but potentially physiologically relevant dosage increase in transcriptional regulators such as TBX5 are not well characterized.
We deleted the mouse orthologues of two AF variant-rich regions in the human TBX5 locus to investigate how variant regions associated with a common disease modulate phenotype in a tissue- and developmental stage-specific manner. Each deletion caused a modest 30% increase in Tbx5 expression in different heart compartments and at different stages of life. We report the relatively large effect of this modest increase in Tbx5 expression on atrial function including arrhythmia susceptibility, and on the gene regulatory network. Decreased expression of Prrx1 has been associated with AF in human and mouse models (Roselli et al., 2018; Bosada et al., 2021; Tucker et al., 2017). We observed a genetic interaction between Tbx5 and Prrx1, and found that some of the transcriptional and functional changes in the atria caused by increased Tbx5 expression were rescued by reducing cardiac Prrx1 expression.
Results
Identification of two AF-associated regulatory regions in the TBX5 locus
The topologically associated domain (Dixon et al., 2012; Nora et al., 2012) harboring Tbx5 shows very limited contact with the adjacent domains harboring Tbx3 and Rbm19, respectively (van Weerd et al., 2014). Because all AF-associated variants in this locus are found within the topologically associated domain of TBX5 (Figure 1A), we anticipate that REs affected by the risk variants modulate the expression of TBX5 only. Promoter capture Hi-C maps from iPSC-derived cardiomyocytes (Montefiori et al., 2018) show distinct contacts between the promoter of Tbx5 and distal AF-associated regions, including the region in the last intron of TBX5 (Figure 1A). To identify possible regulatory elements within the AF-associated regions, we analyzed epigenomic datasets in both human (Gilsbach et al., 2018; van Ouwerkerk et al., 2019) and mouse orthologous region (Figure 1A–B). We selected two regions: the first situated in the last intron (RE(int)), and the second immediately downstream (RE(down)) of Tbx5. Both fragments contain evolutionary conserved regions, and RE(int) harbors accessible chromatin sites in the left atria and ventricles, and EMERGE enhancer prediction signal (van Duijvenboden et al., 2019; van Ouwerkerk et al., 2019). Additionally, RE(int) and RE(down) contain regions associated with cardiac H3K4me1, and RE(int) with H3K27ac (Gilsbach et al., 2014; Figure 1B). The SNPs in the last intron and those downstream of the gene are clustered into two distinct haplotypes, suggesting that these two regions are independently inherited (Figure 1—figure supplement 1; Machiela and Chanock, 2015). Using CRISPR/Cas9 genome editing, we deleted these candidate REs from the mouse genome to test their function in vivo.
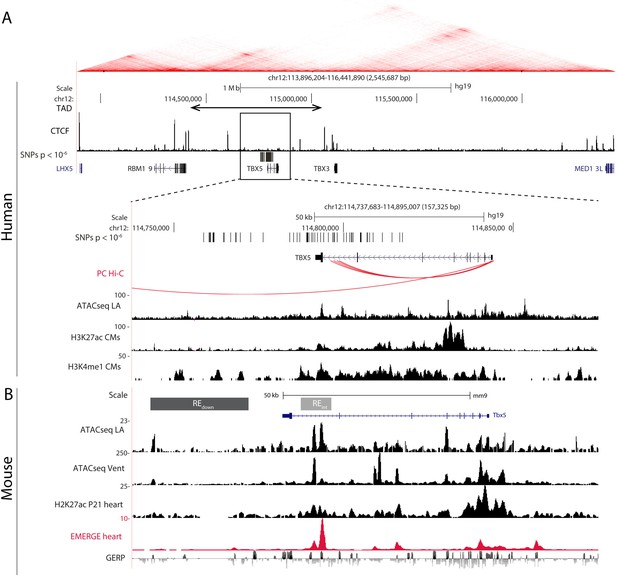
AF-associated noncoding variants are found in the TBX5 locus.
(A) Hi-C heatmap from human lymphoblastoid line GM12878 shows AF-associated variants are found in the regulatory domain of TBX5. Zoom-in of the AF-associated region overlaid with promoter capture Hi-C (red arcs), regions of open chromatin in whole left atria (ATACseq LA), H3K27ac and H3K4me1 ChIPseq signatures in cardiomyocytes. (B) Mouse orthologue of the human region including ATACseq from left atrial and ventricular CMs, H3K27ac ChIPseq from whole juvenile hearts, EMERGE, and conservation tracks. CRISPR/Cas9-generated deletions in light gray (REint), and dark gray (REdown).
Tbx5 expression and arrhythmia predisposition are increased in atria of Tbx5RE(int)KO and Tbx5RE(down)KO mice
Before birth, expression of Tbx5 in the atria was not different between genotypes (Figure 2—figure supplement 1A). However, in juvenile atria of RE(int) KO mice (p=0.001), Tbx5 expression was slightly increased compared to controls (Figure 2A). Expression of Rbm19, Tbx3, and Med13l, which neighbor Tbx5, remained unchanged in atria or ventricles of both mutants (Figure 2—figure supplement 1). Both atria of Tbx5RE(int)KO adult mice expressed approximately 30% more Tbx5, whereas only the left atrium and lungs of Tbx5RE(down)KO adult mice expressed more Tbx5 (Figure 2B–C). While we were not able to assess quantitative differences in protein expression, we observed that Tbx5 protein was selectively present in PCM-1 +cardiomyocyte nuclei in left and right atria of both control and of Tbx5RE(int)KO mice (Figure 2—figure supplement 2). We confirmed absence of aberrant splicing caused by the intronic deletion in Tbx5RE(int)KO (Figure 2—figure supplement 3). Interestingly, eQTL analysis indicated that AF is associated with a statistically significant 30% increase in TBX5 expression in cardiac tissue (Roselli et al., 2018). This suggests that AF-associated variants in the corresponding human regions may mediate the increase in TBX5 expression observed in patients with risk variants.
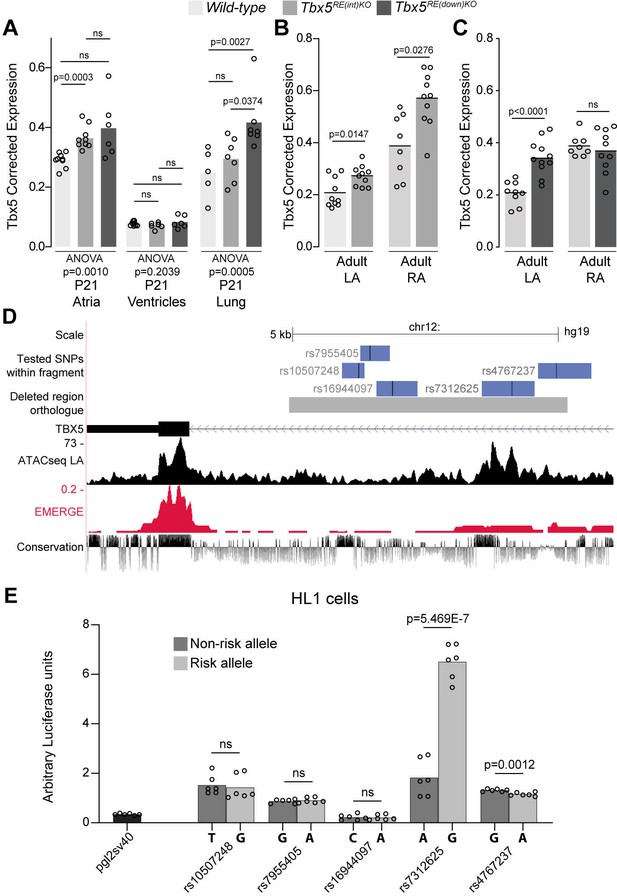
Deletion of AF-associated regions results in increased Tbx5 in adult atrial tissue.
(A) Tbx5 expression in atria, ventricles, and lungs from P21 control, Tbx5RE(int)KO, and Tbx5RE(down)KO mice determined by RT-qPCR. (B–C) Tbx5 expression levels in adult control and Tbx5RE(int)KO (B) or Tbx5RE(down)KO (C) left and right atria. (D) UCSC browser view of the human intronic region (gray) and tested fragments containing AF-associated SNPs (blue) overlaid with chromatin conformation, EMERGE, and conservation tracks. (E) Luciferase assay (n=6) shows enhancer activity differences between non-risk (dark gray) or risk (gray) alleles (Kruskal-Wallis p=0.0019). Statistical significance within each tissue type was determined with ANOVA followed by pairwise comparisons using Dunnett’s T3 multiple comparison test in A, unpaired t-tests in B, C, and Kruskal-Wallis tests followed by pairwise unpaired t-tests (p values shown in figure) in E.
To identify functional AF-associated variants within the human intronic RE region, we tested enhancer activity of five fragments containing an AF-associated SNPs (p<10–6) (Roselli et al., 2018) using luciferase assays in the atrial cardiomyocyte-like cell line HL-1 (Figure 2D). Of the tested fragments, the fragment containing rs7312625 showed increased enhancer activity, and the fragment containing rs4767237 showed minimal decreased activity (Figure 2E). Comparative TF motif analysis between the regions containing the major (A) or minor (G) allele of rs7312625 revealed that the minor (G) allele causes disruption of motifs for Arabidopsis SOL1/2 (TCX/TCX2) (Supplementary file 1), which are homologues of animal LIN54 (Marceau et al., 2016; Simmons et al., 2019), a DNA-binding component of the DREAM complex regulating cell cycle-dependent transcription (Sadasivam and DeCaprio, 2013). We also observed gain of a sine oculis (SIX) homeodomain transcription factor binding motif (Meurer et al., 2021; Supplementary file 1). Lin54 and both Six4 and Six5 are expressed in atrial tissue of mice (see Supplementary files 2 and 3).
Next, we recorded in vivo electrocardiograms (ECGs) to determine the functional consequences of such a modest increase in Tbx5. Both Tbx5RE(int)KO and Tbx5RE(down)KO mice had slower and more variable heart rates (R-R interval (RR); standard deviation of normal to normal R-R intervals (SDNN)) (Figure 3A and B). Additionally, we detected instances of sinus pauses and inverted P waves in mice with both deletions (Figure 3—figure supplement 1A). PR interval was significantly increased in Tbx5RE(int)KO mice, but remained unaffected in Tbx5RE(down)KO mice (Figure 3C). Other ECG parameters were not affected (Figure 3—figure supplement 1). Heart rate corrected sinus node recovery time (cSNRT) and Wenckebach cycle length (WBCL) measured during transesophageal pacing (Bosada et al., 2021; Verheule et al., 2004) were increased in both mouse models (Figure 3D and E), suggesting altered conduction system function. We next tested whether AA could be induced in both mouse lines using in vivo burst pacing (Figure 3G [typical AA traces]). The total duration of all AA episodes per mouse was greater in both deletion models when compared to control littermates (Figure 3F [top graph]). Yet, we were able to induce AAs more often in Tbx5RE(int)KO, but not Tbx5RE(down)KO mice compared to controls (Figure 3F [below graph]).
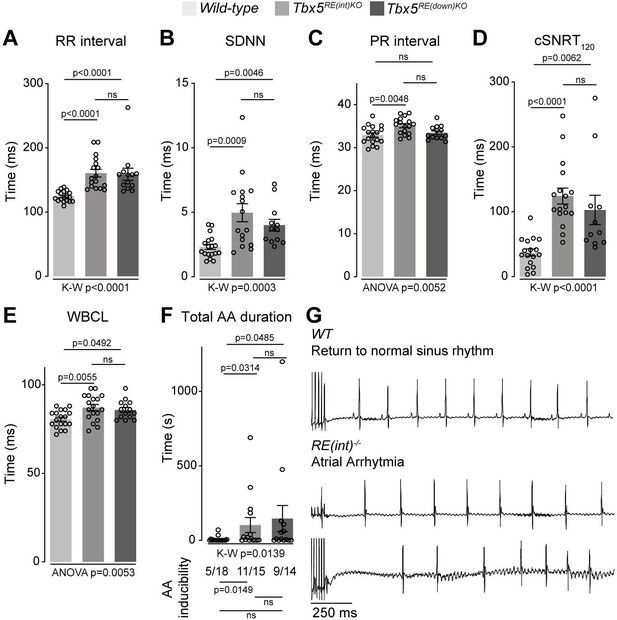
Increased Tbx5 in adult atria results in altered in vivo electrophysiology.
(A–C) Graphs show individual and average ECG measurements for heart rate (RR) (A), heart rate variation (SDNN) (B), and PR-interval (C) of adult wild-type, Tbx5RE(int)KO, and Tbx5RE(down)KO mice. (D, E) Graphs show changes in heart rate-corrected sinus node recovery times at 120ms (cSNRT120) (D), and Wenckebach cycle length (WBCL) (E). (F) Bar graph depicts the total time each mouse spent in an atrial arrhythmia (AA) episode after two pacing passes, with the number of mice in which at least one episode lasting >1 s was observed below each bar. (G) Representative traces from wild-type (top) and two Tbx5RE(int)KO (bottom) individuals showing disappearance of p waves or the start of atrial arrhythmia with variability in ventricular response after pacing stimulus. Significance for in vivo parameters was determined with Kruskal-Wallis test followed by Dunn’s multiple comparison tests in A, B, D, and AA duration in F (top graph), and one-way ANOVA followed by Tukey’s multiple comparisons test in C and E. AA inducibility significance was determined with pairwise Fisher’s exact test (F bottom of graph).
To further characterize the electrophysiological phenotypes of Tbx5RE(int)KO mice we analyzed the properties of single isolated left atrial cardiomyocytes using patch-clamp. At 6 Hz stimulation, we observed APD prolongation in mutants at all measured repolarization stages, with no changes in AP upstroke velocity, resting membrane potential and maximal AP amplitude (Figure 4A–C). APD increase was present at all frequencies measured (Figure 4D). Because Tbx5 has important roles in intracellular Ca2+ handling (Dai et al., 2019; Laforest et al., 2019; Zhu et al., 2008), we measured intracellular Ca2+ concentration ([Ca2+]i) in isolated atrial CMs using fluorescent calcium indicator Indo-1. We did not observe any changes in systolic or diastolic [Ca2+]i concentration (Figure 4E). Together, our data show that a slight increase in Tbx5 disturbs atrial function and can predispose to arrhythmia.
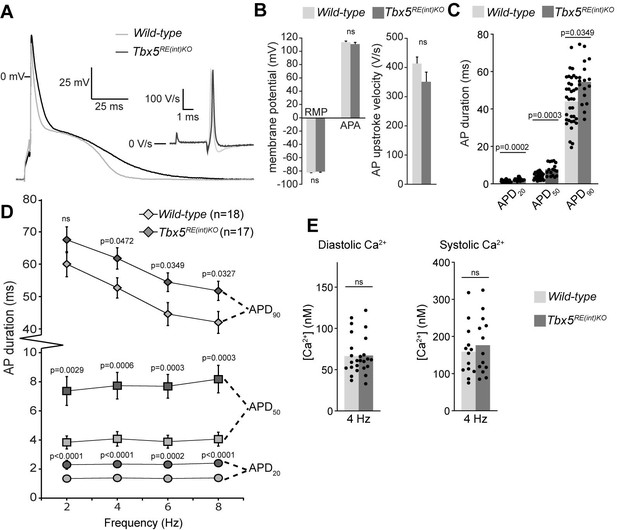
Atrial function abnormalities in Tbx5RE(int)KO mice.
(A) Typical action potentials (APs) and upstroke velocity measured at 6 Hz in single left atrial cardiomyocytes using the amphotericin-perforated patch clamp technique. (B) Average resting membrane potential (RMP), AP amplitude (APA), and AP upstroke velocity remain unchanged in mutants. (C) AP duration at 20, 50, and 90% of repolarization (APD20, APD50, APD90, respectively) is increased in mutants. (D) APD90 was prolonged at all tested frequencies in mutant left atrial cardiomyocytes. Error bars are SD. (E) Diastolic and systolic intracellular Ca2+ concentrations ([Ca2+]i) were not changed in Tbx5RE(int)KO compared to controls. Statistical significance in B was determined with unpaired t-tests with Welch’s correction, and unpaired t-tests were used in B. Experimental groups were compared using two-way repeated measures ANOVA (D, E), followed by pairwise unpaired t-tests (C). Error bars are SEM.
Sensitivity of the atrial gene regulatory network to modestly increased Tbx5 dosage
To gain insight into the mechanism underlying the changes in electrophysiological properties in mutants, we performed transcriptional profiling of whole left atria. We detected 13790 and 13564 different transcripts in Tbx5RE(int)KO and Tbx5RE(down)KO, respectively, of which 816 were significantly up- and 1235 were downregulated in Tbx5RE(int)KO (Figure 5A; adjusted p<0.05), and 112 were up- and 123 were downregulated in Tbx5RE(down)KO (Figure 5B; adjusted p<0.05). Both datasets shared a high proportion of deregulated genes, with fewer significantly deregulated genes observed in Tbx5RE(down)KO compared to Tbx5RE(int)KO (Figure 5C). These data indicate that transcriptomes in Tbx5RE(int)KO atria and in Tbx5RE(down)KO atria are comparably affected qualitatively, but not quantitatively, consistent with the slightly higher increase in Tbx5 expression in Tbx5RE(int)KO compared to Tbx5RE(down)KO. Gene Ontology analysis (Mi et al., 2019) revealed that processes involved in cellular compartment organization, ion transport, and cardiac conduction characterized the transcripts found in the upregulated genes, and extracellular matrix organization, vasculature development, and actin cytoskeleton organization were found in the downregulated set in both deletions (Figure 5D). Accordingly, several Tbx5 target genes known to affect APD or whose deregulation may result in electrophysiological changes, (Dai et al., 2019; Laforest et al., 2019; Nadadur et al., 2016; Zhu et al., 2008) were significantly deregulated in one or both deletion lines (Figure 5F, Figure 5—figure supplement 1).
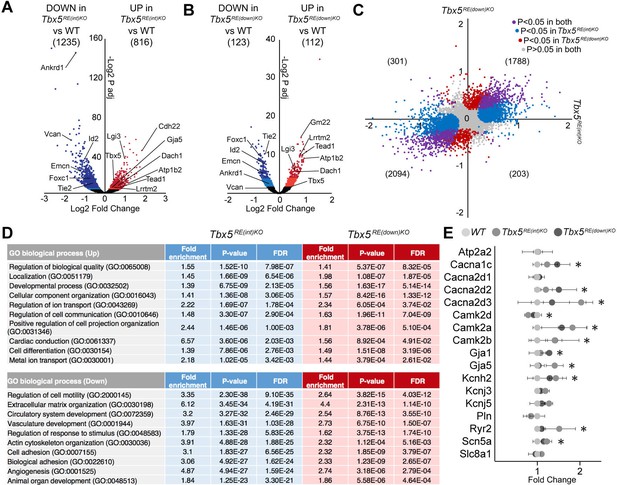
Transcriptomic analysis of Tbx5RE(int)KO left atria.
(A, B) Volcano plot showing differentially expressed transcripts in Tbx5RE(int)KO (n=3)(A) and Tbx5RE(down)KO (n=3) (B) from wild-type (n=4) left atria. Dark red and light red dots indicate significantly upregulated genes by raw p-value and p-adjusted for multiple testing, respectively. Correspondingly, dark blue and light blue dots indicate downregulated genes. p Values were adjusted for multiple testing using the false discovery rate (FDR) method of Benjamini-Hochberg. (C) X-Y plot of all transcripts in Tbx5RE(int)KO (x axis) and Tbx5RE(down)KO (y axis), with deregulated genes common to both deletion mutants in purple, Tbx5RE(int)KO deregulated genes in blue, and Tbx5RE(down)KO deregulated genes in red. (D) Gene ontology (GO) analysis of upregulated and downregulated genes in Tbx5RE(int)KO and Tbx5RE(down)KO mutants. (F) Graph depicts fold change expression in control and mutant samples of genes known to affect action potential duration. * denotes significantly deregulated in one or both mutant lines.
Next, we compared the transcriptional response to increased Tbx5 in left atria (Tbx5RE(int)KO) to that of a gain-of-function missense mutation (Tbx5G125R/+) (van Ouwerkerk et al., 2022), and noticed that it was unexpectedly divergent, with only 80 transcripts significantly deregulated in the same direction in both datasets (Figure 5—figure supplement 2A, Supplementary file 4). In contrast, comparison with left atria of inducible adult-specific Tbx5 deletion (Tbx5iKO) (Nadadur et al., 2016) revealed that the majority of significantly deregulated genes common to both datasets were changed in opposite direction (Figure 5—figure supplement 2B, Supplementary file 5), thus indicating the Tbx5-dependent regulatory network responds in opposite direction to increased- or decreased Tbx5 dosage, respectively. PITX2 is strongly associated with AF and functionally implicated in atrial rhythm control (Kirchhof et al., 2011; Tao et al., 2014; Wang et al., 2010; Zhang et al., 2019), and was previously found to be a Tbx5 target in the left atrium (Nadadur et al., 2016). However, we did not detect a change in Pitx2 expression in either the left or right atria of Tbx5RE(int)KO mice (Figure 5—figure supplement 3).
To determine whether a slight increase in Tbx5 expression in cardiomyocytes results in changes in chromatin accessibility, we performed ATAC-sequencing profiling (ATACseq) of Tbx5RE(int)KO left atrial cardiomyocytes (Buenrostro et al., 2015). After peak-calling, we found a total of 85,569 accessible sites common to both genotypes, and only 15 sites of increased accessibility and 32 of decreased accessibility in mutants (Figure 5—figure supplement 4A). Two of the sites with decreased accessibility were found in the locus of Tbx5 (one of which caused by the deletion of the intronic genomic fragment in Tbx5RE(int)KO mice), suggestive for increased direct transcriptional autoregulation in Tbx5RE(int)KO mice (Figure 5—figure supplement 4B). The very minor changes in chromatin accessibility indicate that a modest increase in Tbx5 expression does not significantly change transcription factor occupancy or epigenetic state.
An interaction between Tbx5 and Prrx1, two AF-associated genes
We considered whether the presence of two AF-risk alleles would exacerbate the phenotype(s) consistent with AF. PRRX1, encoding the transcription factor Paired Related Homeobox 1, has been linked to AF-predisposition (Bosada et al., 2021; Roselli et al., 2018; Tucker et al., 2017). Reduced PRRX1 expression in human cardiac tissues has been associated with AF (Roselli et al., 2018). We previously deleted the mouse orthologous variant region near PRRX1 (Prrx1(enh)) to investigate its role in gene regulation and rhythm control (Bosada et al., 2021). Prrx1(enh)KO mice express less Prrx1 specifically in cardiomyocytes compared to controls, and show atrial conduction slowing, lower AP upstroke velocity (indicative for lower Na+ current densities; Berecki et al., 2010), as well as increased systolic and diastolic [Ca2+]i concentration that culminate in increased susceptibility to atrial arrhythmia induction (Bosada et al., 2021). To explore whether and how these two AF-risk genes may interact, we intercrossed Tbx5RE(int)KO with Prrx1(enh)KO mice (decreased Prrx1 in cardiomyocytes), and investigated cardiac transcriptomes and phenotypes across genotypes. We isolated cardiomyocyte and non-cardiomyocyte nuclei from whole hearts using a PCM-1 antibody and interrogated Tbx5 and Prrx1 expression across all genotypes. Tbx5 was upregulated in Tbx5RE(int)KO cardiomyocytes, as expected, but not deregulated in Prrx1(enh)KO or double mutant cardiomyocytes (Figure 6A). Prrx1 levels were increased in Tbx5RE(int)KO cardiomyocytes and decreased in Prrx1(enh)KO, as expected, and also decreased in double mutant cardiomyocytes (Figure 6B). There were no statistically significant changes detected in the non-cardiomyocyte fractions (Figure 6A and B). These data indicate that in cardiomyocytes, Tbx5 regulates Prrx1, and that a regulatory feedback loop modulates Tbx5 levels when Prrx1 expression is reduced due to the Prrx1(enh) deletion.
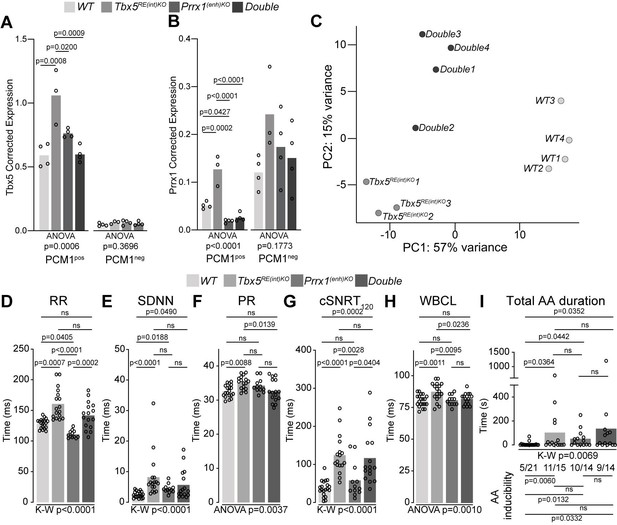
A genetic interaction between Tbx5 and Prrx1.
(A, B) Graph shows reference gene-corrected expression of Tbx5 (A), and Prrx1 (B) in PCM1-positive and -negative nuclei fractions of wild-type, Tbx5RE(int)KO, Prrx1(enh)KO and double mutant whole hearts determined by RT-qPCR. (C) Principal component analysis of transcriptomes of wild-type, Tbx5RE(int)KO and DoubleKO left atrial samples. (D–H) Graphs show individual and average ECG measurements for RR (D), heart rate variation (SDNN) (E), PR interval (F), cSNRT at 120ms pacing (G), and WBCL (H) of wild-type, Tbx5RE(int)KO, Prrx1(enh)KO and DoubleKO mice. (I) Bar graph depicts the total time each mouse spent in an AA episode after two pacing passes, with the number of mice in which at least one episode lasting >1 s was observed below each bar. Statistical significance was determined using one-way ANOVA followed by Tukey’s test for pairwise comparisons in A, B, F, H, Kruskal-Wallis test followed by Dunn’s test for pairwise comparisons in D, E, G, and AA duration in I (top of graph), Fisher’s exact test for pairwise comparisons of AA inducibility in I (bottom of graph).
We next performed transcriptome analysis and found that deregulated genes in left atria of Tbx5RE(int)KO;Prrx1(enh)KO double mutants were more similar to the transcript changes found in Tbx5RE(int)KO than the ones found in Prrx1(enh)KO, when comparing all lines to WT, suggesting a dominant contribution of Tbx5RE(int)KO to the transcriptional changes in double mutants (Figure 6—figure supplement 1A-C, Supplementary files 6-7). Using principal component analysis, we found that transcriptomes clustered according to genotype (Figure 6C). Compared to atrial transcriptomes of Tbx5RE(int)KO mice, those of Tbx5RE(int)KO;Prrx1(enh)KO double homozygous showed less variance with WT atrial transcriptomes (Figure 6C, Figure 6—figure supplement 1, Table 1). This suggested an interaction between Tbx5 and Prrx1, in which reduced Prrx1 expression partially normalizes increased Tbx5-induced transcriptomic changes.
Gene ontology (GO) analysis of upregulated and downregulated genes in Tbx5RE(int)KO, Prrx1(enh)KO, and Double homozygous adult left atria.
Prrx1(enh)KO | Tbx5RE(int)KO | Double | ||||||||||
---|---|---|---|---|---|---|---|---|---|---|---|---|
GO biological process | UP/DOWN | Fold enrich | P-value | FDR | UP/DOWN | Fold enrich | P-value | FDR | UP/DOWN | Fold enrich | P-value | FDR |
Regulation of biological quality (GO:0065008) | DOWN | 1.64 | 1.7E-08 | 3E-06 | UP | 1.55 | 1.5E-10 | 8E-07 | UP | 1.56 | 3.4E-08 | 1.5E-05 |
Localization (GO:0051179) | DOWN | 2.11 | 1.5E-14 | 1.6E-11 | UP | 1.45 | 1.7E-09 | 6.5E-06 | UP | 1.44 | 2.9E-07 | 8E-05 |
Developmental process (GO:0032502) | UP | 2.33 | 6.1E-20 | 1.6E-16 | UP | 1.39 | 6.8E-09 | 2.1E-05 | UP | 1.61 | 1.8E-14 | 2.9E-10 |
Cellular component organization (GO:0016043) | DOWN | 1.85 | 4.3E-08 | 6.9E-06 | UP | 1.41 | 1.4E-08 | 3.1E-05 | UP | 1.54 | 1.3E-10 | 2.2E-07 |
Regulation of ion transport (GO:0043269) | DOWN | 2.68 | 8.7E-08 | 1.3E-05 | UP | 2.22 | 1.7E-07 | 0.00018 | UP | 2.36 | 7.6E-07 | 0.00018 |
Regulation of cell communication (GO:0010646) | DOWN | 2.18 | 2.8E-18 | 1.1E-14 | UP | 1.48 | 3.3E-07 | 0.00029 | UP | 1.57 | 3.4E-07 | 8.9E-05 |
Positive regulation of cell projection organization (GO:0031346) | DOWN | 2.07 | 0.00033 | 0.0158 | UP | 2.44 | 1.5E-06 | 0.001 | UP | 2.08 | 3.9E-07 | 0.0001 |
Cardiac conduction (GO:0061337) | DOWN | 7.1 | 0.00011 | 0.00597 | UP | 6.57 | 3.6E-06 | 0.00203 | UP | 7.36 | 1E-05 | 0.0015 |
Cell differentiation (GO:0030154) | UP | 2.25 | 2.8E-11 | 8.2E-09 | UP | 1.39 | 7.9E-06 | 0.00276 | UP | 1.65 | 4.8E-10 | 5.8E-07 |
Metal ion transport (GO:0030001) | DOWN | 3.43 | 4.5E-08 | 7.3E-06 | UP | 2.18 | 1E-05 | 0.00342 | UP | 2.25 | 0.00069 | 0.049 |
Regulation of cell motility (GO:2000145) | UP | 2.96 | 2.1E-14 | 1.2E-11 | DOWN | 3.35 | 2.3E-38 | 9.1E-35 | DOWN | 2.28 | 2.5E-05 | 0.00564 |
Extracellular matrix organization (GO:0030198) | UP | 7.57 | 2E-24 | 3.1E-20 | DOWN | 6.12 | 3.5E-34 | 4.2E-31 | DOWN | 7.87 | 3.2E-17 | 5.1E-13 |
Circulatory system development (GO:0072359) | UP | 3.3 | 2.8E-17 | 3.7E-14 | DOWN | 3.2 | 3.3E-32 | 2.5E-29 | DOWN | 3.14 | 2.3E-10 | 9.1E-07 |
Vasculature development (GO:0001944) | UP | 4.11 | 4.6E-17 | 4.8E-14 | DOWN | 3.97 | 1.6E-31 | 1E-28 | DOWN | 3.23 | 3.2E-07 | 0.00017 |
Regulation of response to stimulus (GO:0048583) | UP | 1.91 | 1.8E-17 | 2.6E-14 | DOWN | 1.79 | 1.3E-28 | 5.8E-26 | DOWN | 1.61 | 2.2E-06 | 0.00072 |
Actin cytoskeleton organization (GO:0030036) | DOWN | 3.17 | 4.6E-08 | 7.3E-06 | DOWN | 3.91 | 4.9E-28 | 1.9E-25 | DOWN | 3.26 | 7.9E-07 | 0.00034 |
Cell adhesion (GO:0007155) | UP | 2.92 | 3.9E-12 | 1.5E-09 | DOWN | 3.1 | 1.8E-27 | 6.6E-25 | DOWN | 2.99 | 1.1E-08 | 1.9E-05 |
Biological adhesion (GO:0022610) | UP | 2.94 | 2E-12 | 8.2E-10 | DOWN | 3.06 | 4.9E-27 | 1.6E-24 | DOWN | 2.95 | 1.4E-08 | 2E-05 |
Angiogenesis (GO:0001525) | UP | 4.5 | 4.9E-12 | 1.8E-09 | DOWN | 4.87 | 4.9E-27 | 1.6E-24 | DOWN | 4.17 | 3.7E-07 | 0.00019 |
Animal organ development (GO:0048513) | UP | 2.03 | 8.4E-17 | 7.4E-14 | DOWN | 1.84 | 1.3E-23 | 3.3E-21 | DOWN | 1.78 | 3.4E-07 | 0.00018 |
Next, we considered whether the adult electrophysiological changes observed in Tbx5RE(int)KO would also be rescued by the presence of this second AF-risk allele. In vivo ECGs and burst pacing experiments revealed that RR, PR interval, and WBCL were normalized in double homozygous mice, whereas SDNN, cSNRT, and AA inducibility remained unchanged (Figure 6D–I).
Taken together, our findings suggest that an interaction between Tbx5 and Prrx1 exists; in which Prrx1 expression is induced by Tbx5, where Prrx1 is required to induce Tbx5 expression in Prrx1(enh)KO mice, and increased Tbx5 imposes changes in expression and electrophysiological properties, in part through increasing Prrx1 expression in cardiomyocytes.
Discussion
Our study reveals that mouse orthologues of two independent variant regions in the TBX5 locus modulate Tbx5 expression in tissue- and stage-specific manners, causing distinct specific phenotypes. While the impact of 50–100% dose reduction of Tbx5 in humans and model systems has been well-investigated (Bamshad et al., 1997; Bruneau et al., 2001; Kathiriya et al., 2021Li et al., 1997; Luna-Zurita et al., 2016; Mori et al., 2006; Moskowitz et al., 2004), we here show the effect of physiologically relevant increases in expression of Tbx5. This is significant as both decreased and increased expression of genes has been associated with increased AF risk.(Roselli et al., 2018). Our study reveals that atrial- and postnatal-specific increase in Tbx5 levels of only 30% affects postnatal atrial gene regulation, function and arrhythmia propensity. Furthermore, we provide an example of the interaction between the effect of two independent AF-associated variant regions (TBX5 and PRRX1) on phenotype. Our models provide insight into the mechanisms underlying the pleiotropic effects and interactions of disease-associated regulatory variants, which typically cause small changes in target gene expression in particular cell-types, conditions or stages of development (GTEx Consortium, 2020; Sobreira et al., 2021; Strober et al., 2019; Watanabe et al., 2019).
We found that the mouse orthologues of two noncoding regions studied here containing AF-associated variants in the TBX5 locus harbor REs that independently controlled Tbx5 expression in the heart in vivo. Deletion of either of the two RE-containing regions only affected Tbx5 expression, consistent with the notion that Tbx5 and surrounding RE regions are largely confined to one topologically associated domain not shared with adjacent genes (van Weerd et al., 2014). The mechanism of the repressive action of the REs and cross-talk with the other REs in the locus (Smemo et al., 2012) remain to be established. For RE(int), which showed the largest effect size in atria upon deletion, we identified two AF-associated SNPs that caused differential transcriptional activity of the RE sub fragment in HL1 atrial cardiomyocyte-like cells; one variant caused ~sixfold increase and the other minimally decreased activity. The risk allele of the first variant, rs7312625, disrupts a potential Lin54 motif, implying loss of interaction with the DREAM complex (Sadasivam and DeCaprio, 2013), and thus suggesting possible cell cycle-dependent regulation that remains to be elucidated. We speculate that in individuals carrying the variant that increases the activity of RE(int) in HL-1 cells, the repressive activity of RE(int) is reduced in the context of the entire regulatory system, thus causing increased TBX5 expression. The opposite could be true for the second variant, yet the small effect would likely be experimentally undetectable. On the other hand, the AF-associated variants in RE(down) may increase Tbx5 expression in both the left atrium and pulmonary vein myocardium. The pulmonary veins have been strongly implicated in AF as they are the most common source of triggered activity (Haïssaguerre et al., 1998; Jai¨s et al., 1997). TBX5 levels in pulmonary vein myocardium may influence gene regulation independently from the atrial TBX5 levels (Steimle et al., 2020). The variants in RE(down) segregate independently from those in RE(int), indicating these variant RE regions act through distinct tissue-specific transcriptional mechanisms that are influenced by AF-predisposing common variants. A further implication is they may cumulatively increase AF predisposition in a manner dependent on risk variant dose, in which homozygous carriers of risk haplotypes in RE(int) and RE(down) have the largest relative predisposition. These relations could be addressed in future genotype-phenotype analyses of human atrial and pulmonary vein samples.
Heart rates of the Tbx5RE(int)KO mice were slower and more variable than of controls. In addition, PR interval was prolonged in Tbx5RE(int)KO mice combination with increased Wenckebach cycle lengths. The latter is in line with the important role of Tbx5 in sinus node and AV node development and function (Mori et al., 2006; Moskowitz et al., 2007; Moskowitz et al., 2004; van Eif et al., 2018). Moreover, atrial arrhythmias could be more easily induced in Tbx5RE(int)KO mice suggesting electrophysiological remodeling of the atria. It has been shown that mice haploinsufficient for Tbx5 show atrial downregulation of genes encoding proteins involved in cardiac conduction (e.g. Gja1/Cx43 and Scn5a/Nav1.5) and Ca2+ handling (e.g Atp2a2/Serca2a and Ryr2/Ryr2) (Nadadur et al., 2016; Zhu et al., 2008). This leads to slower conduction, reduced Ca2+ concentration in the sarcoplasmic reticulum [Ca2+]SR, increased incidence of early and delayed afterdepolarization, and accordingly, increased AF propensity (Laforest et al., 2019; Nadadur et al., 2016). In the atria of our Tbx5RE(int)KO mice, where expression of Tbx5 was slightly elevated, transcriptomic analysis indicated upregulation of genes involved in cardiac conduction including Scn5a and Cx43. The Ca2+ handling genes Atp2a2/Serca2a and Ryr2/Ryr2 were not differently expressed between Tbx5RE(int)KO mice and controls. Accordingly, in our mice, we did not observe any changes in Ca2+ transients and long decay constants as seen in Tbx5 haploinsufficient mice (Laforest et al., 2019). Expression of Cacna1c and Cacna2d2/Cacna2d3, encoding subunits of the L-type Ca2+ channel, was upregulated in the atrium of Tbx5RE(int)KO mice. This is consistent with the observed longer atrial APD in Tbx5RE(int)KO mice compared to controls, which may predispose to increased arrhythmia inducibility, as seen in mice harboring the pathogenic TBX5-G125R variant or Prrx1 enhancer deletion (Bosada et al., 2021; van Ouwerkerk et al., 2022). Although shortened APD is typically found in AF patients with sustained arrhythmia (Kim et al., 2002; Wu et al., 2001), prolonged APD has been previously associated with increased risk of developing AF (Bosada et al., 2021; Lee et al., 2016; Olson et al., 2006; Simpson et al., 1988; van Ouwerkerk et al., 2022).
The molecular mechanisms underlying electrophysiological remodeling have remained unclear. For example, atrial cardiomyocytes of Tbx5 iKO (Tbx5 deletion induced in adult mice), of Tbx5+/G125R (heterozygous for a gain of function missense mutation) and of Tbx5RE(int)KO mice show APD prolongation (Nadadur et al., 2016; van Ouwerkerk et al., 2022). When comparing the transcriptional changes in left atria of these mouse models, we observed that it was unexpectedly divergent between the Tbx5RE(int)KO and Tbx5+/G125R models, whereas significantly differentially expressed genes common to both Tbx5RE(int)KO and Tbx5 iKO models were changed in opposite direction (Figure 5—figure supplement 2, Supplementary files 4 and 5). This suggests that the degree and direction of change of expression of, for example, genes implicated in APD (including Cacna2d3, Camk2a, Kcnh2, and Ryr2) do not necessarily predict changes in APD. Furthermore, Pitx2 was downregulated in left atria of Tbx5 iKO mice and upregulated in right atria of Tbx5+/G125R mice (van Ouwerkerk et al., 2022), and Tbx5 and Pitx2 were found to work antagonistically in the left atrium to tightly regulate the expression of genes impacting on cardiac electrophysiology (Nadadur et al., 2016). Yet, we did not detect a change in Pitx2 expression in the atria of Tbx5RE(int)KO mice. Together, these findings indicate that decreasing or increasing Tbx5 dose or changing Tbx5 function induces a large number of divergent transcriptional responses that disturb the balance in the genetic networks underlying functional (electrophysiological) properties of the atria, such as APD, leading to similar phenotypic outcomes.
The risk of developing complex diseases such as AF is strongly influenced by a large number of pleiotropic variants that each confers a small change in overall risk. We found that Tbx5 drives Prrx1 expression, and that Tbx5 levels are Prrx1-dependent (or Prrx1 target-dependent) in specific contexts. We then asked whether combining two alleles modeling the changes in gene expression conferred by AF-associated variant regions would cause a greater effect on heart phenotype, and by extension, arrhythmia predisposition, than each allele alone. Here, we found that introduction of this AF-susceptibility allele Tbx5RE(int)KO mice rescued heart rate, PR interval, and atrioventricular node phenotypes. This example shows that two variant regions that are both independently associated with increased risk for a particular disease, do not necessarily cumulatively increase disease predisposing phenotype, but may neutralize each other’s effect. Previously, the atrial electrophysiological phenotype of Tbx5 haploinsufficient mice was observed to be rescued by haploinsufficiency of either Pitx2 or Gata4, both of which have been associated with AF as well (Laforest et al., 2019; Nadadur et al., 2016). Although the direction of change of expression of the transcription factors in these models does not necessarily correspond to the direction of change in AF patients, this highlights the inherent robustness of the underlying gene regulatory networks, which in general remain stable when individual quantitative parameters such as transcription factor dose or binding sites affinity change (Albergante et al., 2014). This also implies that evaluating specific interactions between AF risk loci will be necessary for ascertaining individual risk from genetic association data.
In conclusion, our data provide unique mechanistic insights into the biological effects of variant region-driven modest physiologically relevant changes in expression of crucial transcriptional regulators, and into the impact of interactions between transcriptional output-modulating risk loci on atrial biology.
Materials and methods
Usage of epigenomic datasets
Request a detailed protocolThe following publicly available epigenomic datasets were used: Hi-C (Rao et al., 2014), AF variants (Roselli et al., 2018), promoter capture Hi-C maps from iPSC-derived cardiomyocytes (Montefiori et al., 2018), accessible chromatin in human and mouse left atria (van Ouwerkerk et al., 2019), cardiac H3K27ac and H3K4me1 ChIP-seq signatures in human (Gilsbach et al., 2014), and EMERGE enhancer prediction signal (van Duijvenboden et al., 2019; van Ouwerkerk et al., 2019).
Generation of mutant mice
Request a detailed protocolMutant mice were generated using CRISPR/Cas9. Guide RNA (sgRNA) constructs were designed with ZiFiT Targeter (Sander et al., 2010). The sgRNA constructs were transcribed in vitro using MEGAshortscript T7 (Invitrogen AM1354) and mMessage Machine T7 transcription kit (Invitrogen AM1344) according to manufacturer instructions. One-cell FVB/NRj zygotes were microinjected with 10 ng/µL each sgRNA and 25 ng/µL Cas9 mRNA to generate mouse founders. Deletions were validated by PCR and Sanger sequencing. The sgRNA target sequences are the following: RE(int) guide 1 (GGGAAATCGCCTTACCTTTC), guide 2 (GGACTGTTGGGTCACCTTGT), and RE(down) guide 1 (GGCTCCTTCGTCAGTAAATA), guide 2 (AAACAAGGGCTCTCTGGCGTTT). The deleted coordinates are the following in mm10: RE(int) chr5:119,874,013–119,880,148, and RE(down) chr5:119,891,017–119,915,744. Founders were backcrossed with wildtype FVB/NJ mice to obtain stable lines. Downstream experiments were performed on F3-F7 mice, backcrossed with wild-type FVB mice. All transgenic mice were maintained on a FVB/NJ background commercially obtained from Jackson laboratory (stock number 100800).
For tissue harvest, animals were euthanized by 20% CO2 inhalation followed by cervical dislocation.
EdU cardiomyocyte proliferation assay
Request a detailed protocolTimed pregnant female mice received intraperitoneal injection of 100 mg/kg EdU one hour before sacrifice by isoflurane and cervical dislocation. E14.5 fetuses were isolated in 1xPBS on ice, the head was removed and the body fixed in 4% PFA for 24 hr. 7 μm sections were stained with mouse-anti-actin (1:400; Sigma A9357), goat-anti-nkx2.5 (1:150; Santa Cruz sc-14033), DAPI (1:1000) and EdU Click-IT (ThermoFisher, C10340) before imaging and counting. Nkx2.5 positive nuclei were designated cardiomyocytes, Nkx2.5 and EdU double positive nuclei were designated proliferating cardiomyocytes. Ratios were normalized within each litter.
qPCR
Request a detailed protocolTotal RNA was isolated from atria, ventricle and lungs from P21 and left and right atria from adult male and female mice using ReliaPrep RNA Tissue Miniprep System (Promega, Z6112) according to the manufacturer’s protocol. cDNA was reverse transcribed with oligo dT primers from 500 ng of total RNA, or random hexamers from 500 pg of CM nuclear RNA, according to the manufacturer’s protocol of the Superscript II Reverse Transcriptase system (Thermo Fisher Scientific, 18064014). Expression levels of candidate target genes were determined by quantitative real-time PCR using a LightCycler 480 Instrument II (Roche Life Science, 05015243001). Expression levels were measured using LightCycler 480 SYBR Green I Master (Roche, 04887352001) and the primers had a concentration of 1 pmol/L. The amplification protocol consisted of 5 min 95 °C followed by 45 cycles of 10 s 95 °C, 20 s 60 °C and 20 s 72 °C. Relative start concentration (N0) was calculated using LinRegPCR (Ruijter et al., 2009). Values were normalized to the geometric mean of two reference genes per experiment (Hprt, Ppia, or Rpl32) (Ruiz-Villalba et al., 2017). The primer sequences are as follows: (all 3’ to 5’) Hprt: TGTTGGATATGCCCTTGACT, GATTCAACTTGCGCTCATCT; Ppia: GGGTGGTGACTTTACACGCC, CTTGCCATCCAGCCATTCAG; Rpl32: GCCTCTGGTGAAGCCCAAG, TTGTTGCTCCCATAACCGATGT; Tbx5: CCCGGAGACAGCTTTTATCG, TGGTTGGAGGTGACTTTGTG; Prrx1: CACAAGCAGACGAAAGTGTGG, GTTGTCCTGTTTCTCCGCTG; Tbx3: CGCCGTTACTGCCTATCAGAA; GCCATTGCCAGTGTCTCGAA.
Cell culture and transfection luciferase assays
Request a detailed protocolRE sub fragments were cloned into a modified pGL2-Basic plasmid containing an SV40 minimal promoter and an adjusted multiple cloning site for in vitro analysis by a transfection luciferase assay. HL1 cells (RRID:CVCL_0303) were grown in 24-well plates in Claycomb medium (Sigma-Aldrich, 51,800 C) supplemented with chemically defined HL-1 FBS substitute (Lonza, 77227), Glutamax (ThermoFisher Scientific, 35050–061) and Pen/Strep (ThermoFisher Scientific, 15070–063). Cells were transfected using polyethylenimine 25 kDa (PEI, Brunschwig, 23966–2) at a 1:3 ratio (DNA:PEI). Standard transfections were carried out using 200 ng of reporter construct per well. 24 hr after transfection, cells were lysed using Renilla luciferase assay lysis buffer (Promega, E291A-C) and luciferase activity measured. Luciferase measurements were performed using a GloMax Explorer (Promega, GM3500). During the measurement, 100 µL D-Luciferin (p.j.k, 102111) was injected (150 µL/s) followed by a 1-s delay and 5 s of measurement. Transfections were carried out at least three times and measured in duplicates. HL-1 cell cultures were routinely tested negative for mycoplasma contamination. HL-1 cell lines were easily distinguished based on cellular morphology and contractility. HL-1 is not found in the database of commonly misidentified cell lines that is maintained by the International Cell Line Authentication Committee.
In vivo electrophysiology
Request a detailed protocol12–20 week old male mice were anesthetized with 5% Isoflurane (Pharmachemie B.V. 061756) and placed on thermostated mat (36 °C) with a steady flow of 1.5% isoflurane during all experiments. Electrodes were inserted subcutaneously in the limbs and connected to an ECG amplifier (Powerlab 26T, AD Instruments). The electrocardiogram (ECG) was measured for 5 min. ECG parameters were determined in Lead II (L-R) based on the last 60 s of the recording. For atrial stimulation, an octapolar CIB’er electrode (NuMED) was advanced through the esophagus to achieve atrial capture. Atrial capture thresholds were determined for each mouse, and all pacing protocols were performed at 2 x threshold. For sinus node recovery time (SNRT) measurements, a 4-s pacing train with a cycle length of 120 or 100ms was used. SNRT was defined as the interval between the last pacing stimulus and onset of the first P wave. To control for differences in sinus rate, SNRT was normalized to resting heart rate (cSNRT = SNRT – RR interval). To determine the Wenckebach cycle length (WBCL) we applied a 4s pacing train starting at a cycle length of 100ms, and decreasing by 2ms until atrioventricular (AV) block was first observed. Atrial arrhythmia (AA) was induced by 1 or 2 s bursts starting with a cycle length of 60ms, decreasing successively with a 2 ms decrement, down to a cycle length of 10ms. AA duration was the sum of time each mouse spent under and AA episode after completion of the two passes. Atrial arrhythmia (AA) inducibility was scored as the number of mice in which at least one episode lasting >1 s of arrhythmia was induced after pacing. Mice of both sexes were used for atrial arrhythmia induction experiments.
Cellular electrophysiology
Request a detailed protocolSingle cells were isolated from left atria of adult male mice by enzymatic dissociation. Therefore, excised hearts were perfused for 5 min in a Langendorff system with a modified Tyrode’s solution containing (in mmol/L): NaCl 140, KCl 5.4, CaCl2 1.8, MgCl2 1.0, glucose 5.5, HEPES 5.0; pH 7.4 (set with NaOH). Subsequently, the hearts were perfused with Tyrode’s solution containing a low Ca2+-concentration (10 μmol/L) for 10 min, after which Liberase TM research grade (Roche Diagnostics, GmbH, Mannheim, Germany, 5401119001) and Elastase from porcine pancreas (Bio-Connect B.V., Huissen, Netherlands, W59168R) were added for 12 min at a concentration of 0.038 mg/mL and 0.01 mg/mL, respectively. All solutions were saturated with 100% O2 and the temperature was maintained at 37 °C. To obtain single cells, the digested left atria was cut into small pieces which were triturated for 4 min through a pipette (tip diameter: 0.8 mm) in the low Ca2+ Tyrode’s solution, supplemented with 10 mg/ml Bovine Serum Albumin (Roche Diagnostics, essential fatty free, fraction V). Single cells were stored at room temperature for at least 45 min before they were used. Quiescent single rod-shaped cells with smooth surfaces were selected for electrophysiological measurements.
Action potentials (APs) were recorded with the amphotericin-B perforated patch-clamp technique using an Axopatch 200B amplifier (Molecular Devices Corporation, Sunnyvale, CA). Voltage control and data acquisition were performed as described previously (Kamel et al., 2021), and APs were low-pass filtered at 5 kHz and digitized at 40 kHz. Potentials were corrected for the estimated liquid junction potentials (Barry and Lynch, 1991).
APs were recorded at 36 ± 0.2°C using the modified Tyrode’s solution. Pipettes (borosilicate glass (Harvard Apparatus, UK)) were filled with solution containing (in mmol/l): K-gluc 125, KCl 20, NaCl 5, amphotericin-B 0.44, HEPES 10, pH 7.2 (KOH). APs were elicited at 2–8 Hz by 3ms,~1.2 × threshold current pulses through the patch pipette. We analyzed resting membrane potential (RMP), maximal AP amplitude (APA), maximum AP upstroke velocity, and AP duration at 20, 50, and 90% repolarization (APD20, APD50, and APD90, respectively). Parameters from 10 consecutive APs were averaged.
Intracellular Ca2+ measurements
Request a detailed protocolIntracellular calcium concentrations: [Ca2+]i were measured at 25 °C in HEPES solution ((mmol/l): Na+ 156, K+ 4.7, Ca2+ 1.3, Mg2+ 2.0, Cl− 150.6, HCO3 4.3, HPO2− 1.4, HEPES 17, Glucose 11 and 1% fatty acid free albumin, pH 7.3) using the fluorescent probe Indo-1 as described previously (Verkerk et al., 2003). In brief, isolated myocytes were exposed to 5 µmol/l of the acetoxymethyl esters of indo-1 during 30 min at 37 °C. Myocytes were attached to a poly-D-lysine (0.1 g/l) treated cover slip placed on a temperature-controlled microscope stage of an inverted fluorescence microscope (Nikon Diaphot) with quartz optics. A temperature-controlled perfusion chamber (height 0.4 mm, diameter 10 mm, volume 30 µL, temperature 37 °C), with two needles at opposite sides for perfusion purposes, was tightly positioned over the cover slip. The contents of the chamber could be replaced within 100ms. Bipolar square pulses for field stimulation (40 V/cm) were applied through two thin parallel platinum electrodes at a distance of 8 mm. One quiescent single myocyte was selected (myocytes with more than one spontaneous oscillation per 10 s were excluded) and the measuring area was adjusted to the cell surface with a rectangular diaphragm. The wavelength of excitation of Indo-1 was 340 nm, applied with a stabilized xenon-arc lamp (100 W). Fluorescence was measured in dual emission mode at 410 and 516 nm. Emitted light passed a barrier filter of 400 nm, a dichroic mirror (450 nm) and respective narrow band interference filters in front of two photomultipliers (Hamamatsu R-2949). Signals were digitized at 1 kHz and corrected for background signals recorded from Indo-1 free myocytes. Ten subsequent Ca2+ transients were averaged from which apparent [Ca2+]i was calculated according to the ratio equation (Grynkiewicz et al., 1985).
Isolation of CM nuclei
Request a detailed protocolNuclei isolation was performed as follows: snap frozen adult left and right atria from adult male and female mice were trimmed and homogenized in lysis buffer containing RNase inhibitor using an Ultra-Turrax homogenizer. Samples were further homogenized with a loose pestle douncer (10 strokes). After a 10 min incubation in the lysis buffer, an additional 10 strokes were performed with a tight pestle. The lysis procedure was monitored by light microscopy to ensure complete tissue and cell lysis and efficient nuclear extraction. The crude lysate was successively passed through 100 and 30 µm mesh filters. The final lysate was spun at 1000 × g for 5 min and the resulting pellet was resuspended in 500 µl staining buffer (5% BSA in PBS) supplemented with RNAse inhibitor. Isolated nuclei were incubated with rabbit polyclonal antibodies specific for pericentriolar material 1 (PCM1) (Sigma-Aldrich; HPA023370) at a dilution of 1:400 for 1 hr rotating at 4 °C. Next, Alexa Fluor 647-conjugated donkey-anti-rabbit 647 antibodies (ThermoFisher Scientific A-31573; 1:500 dilution), and DAPI (1:1000 dilution) were added and the incubation was continued for an additional hour. Samples were spun at 1000 × g for 10 min and washed with 500 µl staining buffer before resuspension in 500 µl staining buffer supplemented with RNAse inhibitor. Intact CM nuclei were sorted on a BD Influx FACS on the basis of DAPI and Alexa Fluor 647 positivity into cold BL +TG buffer from the ReliaPrep RNA Tissue Miniprep System (Promega, Z6112) for RNA isolation, and into resuspension buffer for ATACseq (Buenrostro et al., 2015). RNA was isolated following a gDNA depletion step according to the manufacturer’s instructions. RNA yield and purity was assessed using an Agilent 2100 Bioanalyzer in combination with the RNA Pico chips.
Library preparation and sequencing
Request a detailed protocolFor RNA isolated from left atria, 500 ng was used for library generation with the KAPA mRNA HyperPrep kit (Roche) and sequenced on the HiSeq4000 system (Illumina) with 50 bp single-end reads. RNAseq sample sizes are as follows: Whole adult left atria: 4 WT, 3 Tbx5RE(int)KO, 3 Tbx5RE(down)KO, 4 Prrx1(enh)KO, and 3 Double homozygous.
Differential expression analysis
Request a detailed protocolReads were mapped to the mm10 build of the mouse transcriptome using STAR (Dobin et al., 2013). Differential expression analysis was performed using the DESeq2 package based on a negative binomial distribution model (Love et al., 2014). p-Values were corrected for multiple testing using the false discovery rate (FDR) method of Benjamini-Hochberg. We have used 0.05 as FDR control level. Unsupervised hierarchical clustering was performed on differentially expressed genes using the R package pheatmap version 1.0.8. (http://cran.rproject.org/web/packages/pheatmap/index.html). PANTHER (Mi et al., 2019) was used for gene ontology (GO) biological process analysis. Benjamini–Hochberg correction was performed for multiple testing-controlled p values. Statistically significant enriched terms were functionally grouped and visualized.
ATACseq on CM nuclei
Request a detailed protocolATACseq on FACS-sorted PCM1 +nuclei was performed and analyzed as described in Buenrostro et al., 2015. Approximately 50 k nuclei were used as input. The library was sequenced (paired-end 125 bp) and data was collected on a HiSeq4000.
Peak-calling and motif analysis of ATACseq
Request a detailed protocolReads from ATAC-seq data were mapped to mm10 build of the mouse genome using BWA (Li and Durbin, 2009), the default settings were used. The BEDTools suite was used to distribute the genome wide signal into bins of 500 bp (Quinlan and Hall, 2010). Bins with less than 89 cumulative tags across all 10 samples were discarded as noise. Differential accessibility was assessed using the DESeq2 package based on a model using the negative binomial distribution (Love et al., 2014). p-Values were corrected for multiple testing using the false discovery rate (FDR) method of Benjamini-Hochberg. We have used 0.05 as FDR control level. Continuous bins with differential signal were subsequently merged together using the BEDTools suite.
200 bp summits were determined of ATAC-seq performed in atrial cells (Fernandez-Perez et al., 2019). In total, HOMER (Heinz et al., 2010) was performed on sequences of 2000 neutral called peaks (randomly sampled out of 85,570 peaks), 2000 peaks up (randomly sampled out of 3551 peaks), and 1776 peaks down in Tbx5RE(int)KO, all with random genome as background in HOMER.
Unsupervised hierarchical clustering was performed on differentially detected peaks and genes in RNA-seq and ATAC-seq using the R package pheatmap, version 1.0.8. (http://cran.r-project.org/web/packages/pheatmap/index.html).
Usage of EMERGE
Request a detailed protocolThe genome-wide heart enhancer prediction track generated by EMERGE (van Duijvenboden et al., 2016) was used as a proxy for the presence of putative heart enhancers. The particular prediction used aimed at identifying robustly active heart enhancers. This was accomplished by further annotating the available true positives (Visel et al., 2007) to train the algorithm with on the basis of the consistency in heart activity patterns shown. Full details are outlined in van Ouwerkerk et al., 2019.
Statistics
The experimenters were blind to mouse genotype during all measurements and outcome assessment. Datasets were tested for normality using Shapiro-Wilk test unless specified otherwise. Whole tissue RT-qPCR fold change vs WT in fetal ventricles and adult whole left atria corrected expression changes were analyzed with unpaired t-tests and reference gene-corrected Tbx5 in juvenile whole tissue was analyzed with Welch’s ANOVA followed by Dunnet’s T3 multiple comparison tests within each tissue type. Luciferase assays on HL1 cells were analyzed using Kruskal-Wallis test. In vivo electrophysiology was analyzed with Kruskal-Wallis followed by Dunn’s multiple comparison tests or one-way ANOVA followed by Tukey’s multiple comparison tests. Significance of atrial arrhythmia (AA) duration was determined with Mann Whitney Wilcoxon test and differences in AA inducibility were tested using Fisher’s exact test. Conduction velocity was analyzed with unpaired t-tests with Welch’s correction. For single cell and whole tissue action potential (AP) duration (APD) normality and equal variance assumptions were tested with the Kolmogorov-Smirnov and the Levene median test, respectively. Two groups were compared with unpaired t-test or repeated measures ANOVA followed by pairwise comparison using the Student-Newman-Keuls test. Differences in Ca2+ transient amplitude as well as changes in diastolic and systolic Ca2+ concentrations in atria were tested using two-way ANOVA. Multiple testing corrections were performed independently within each hypothesis. Data are presented as individual data points and mean or mean ± standard error of the mean (SEM) or standard deviation (SD), as indicated, and <0.05 defines statistical significance. Statistical analysis was performed using GraphPad Prism 9.
Data availability
Adult left atrial RNAseq and ATACseq have been deposited under GEO accession numbers GSE189342 and GSE189498.
-
NCBI Gene Expression OmnibusID GSE189342. Tbx5 regulatory element controls atrial function and heart size.
-
NCBI Gene Expression OmnibusID GSE189498. Tbx5 regulatory element controls atrial function and heart size.
References
-
Liquid junction potentials and small cell effects in patch-clamp analysisThe Journal of Membrane Biology 121:101–117.https://doi.org/10.1007/BF01870526
-
A variant noncoding region regulates Prrx1 and predisposes to atrial arrhythmiasCirculation Research 129:420–434.https://doi.org/10.1161/CIRCRESAHA.121.319146
-
Chamber-Specific cardiac expression of Tbx5 and heart defects in Holt-Oram syndromeDevelopmental Biology 211:100–108.https://doi.org/10.1006/dbio.1999.9298
-
Atac-seq: a method for assaying chromatin accessibility genome-wideCurrent Protocols in Molecular Biology 109:21.https://doi.org/10.1002/0471142727.mb2129s109
-
Tbx3 and tbx5 duplication: a family with an atypical overlapping holt-oram/ulnar-mammary syndrome phenotypeEuropean Journal of Medical Genetics 64:104213.https://doi.org/10.1016/j.ejmg.2021.104213
-
Regulatory snps: altered transcription factor binding sites implicated in complex traits and diseasesInternational Journal of Molecular Sciences 22:6454.https://doi.org/10.3390/ijms22126454
-
Star: ultrafast universal RNA-seq alignerBioinformatics 29:15–21.https://doi.org/10.1093/bioinformatics/bts635
-
A new generation of Ca2+ indicators with greatly improved fluorescence propertiesThe Journal of Biological Chemistry 260:3440–3450.https://doi.org/10.1016/S0021-9258(19)83641-4
-
Spontaneous initiation of atrial fibrillation by ectopic beats originating in the pulmonary veinsThe New England Journal of Medicine 339:659–666.https://doi.org/10.1056/NEJM199809033391003
-
Widespread allelic heterogeneity in complex traitsAmerican Journal of Human Genetics 100:789–802.https://doi.org/10.1016/j.ajhg.2017.04.005
-
Action potential duration restitution kinetics in human atrial fibrillationJournal of the American College of Cardiology 39:1329–1336.https://doi.org/10.1016/S0735-1097(02)01760-6
-
Novel Tbx5 duplication in a Japanese family with Holt-Oram syndromePediatric Cardiology 36:244–247.https://doi.org/10.1007/s00246-014-1028-x
-
Atrial fibrillation risk loci interact to modulate Ca2+-dependent atrial rhythm homeostasisThe Journal of Clinical Investigation 129:4937–4950.https://doi.org/10.1172/JCI124231
-
The six family of transcription factors: common themes integrating developmental and cancer biologyFrontiers in Cell and Developmental Biology 9:707854.https://doi.org/10.3389/fcell.2021.707854
-
Panther version 14: more genomes, a new Panther GO-slim and improvements in enrichment analysis toolsNucleic Acids Research 47:D419–D426.https://doi.org/10.1093/nar/gky1038
-
Tbx5-dependent rheostatic control of cardiac gene expression and morphogenesisDevelopmental Biology 297:566–586.https://doi.org/10.1016/j.ydbio.2006.05.023
-
Pitx2 modulates a tbx5-dependent gene regulatory network to maintain atrial rhythmScience Translational Medicine 8:354ra115.https://doi.org/10.1126/scitranslmed.aaf4891
-
Kv1.5 channelopathy due to KCNA5 loss-of-function mutation causes human atrial fibrillationHuman Molecular Genetics 15:2185–2191.https://doi.org/10.1093/hmg/ddl143
-
Tbx5 intragenic duplication: a family with an atypical holt–oram syndrome phenotypeEuropean Journal of Human Genetics 20:863–869.https://doi.org/10.1038/ejhg.2012.16
-
Multi-Ethnic genome-wide association study for atrial fibrillationNature Genetics 50:1225–1233.https://doi.org/10.1038/s41588-018-0133-9
-
Amplification of nonspecific products in quantitative polymerase chain reactions (qPCR)Biomolecular Detection and Quantification 14:7–18.https://doi.org/10.1016/j.bdq.2017.10.001
-
The DREAM complex: master coordinator of cell cycle-dependent gene expressionNature Reviews. Cancer 13:585–595.https://doi.org/10.1038/nrc3556
-
ZiFiT (zinc finger targeter): an updated zinc finger engineering toolNucleic Acids Research 38:W462–W468.https://doi.org/10.1093/nar/gkq319
-
Thresholds, refractory periods, and conduction times of the normal and diseased human atriumAmerican Heart Journal 116:1080–1090.https://doi.org/10.1016/0002-8703(88)90163-9
-
Regulatory variation in a TBX5 enhancer leads to isolated congenital heart diseaseHuman Molecular Genetics 21:3255–3263.https://doi.org/10.1093/hmg/dds165
-
Atrial fibrillation: epidemiology, pathophysiology, and clinical outcomesCirculation Research 120:1501–1517.https://doi.org/10.1161/CIRCRESAHA.117.309732
-
Pitx2, an atrial fibrillation predisposition gene, directly regulates ion transport and intercalated disc genesCirculation. Cardiovascular Genetics 7:23–32.https://doi.org/10.1161/CIRCGENETICS.113.000259
-
Genetic architecture: the shape of the genetic contribution to human traits and diseaseNature Reviews. Genetics 19:110–124.https://doi.org/10.1038/nrg.2017.101
-
Novel mutation in flnc (filamin c) causes familial restrictive cardiomyopathyCirculation. Cardiovascular Genetics 10:e001780.https://doi.org/10.1161/CIRCGENETICS.117.001780
-
Transcriptional regulation of the cardiac conduction systemNature Reviews Cardiology 15:617–630.https://doi.org/10.1038/s41569-018-0031-y
-
Epigenetic and transcriptional networks underlying atrial fibrillationCirculation Research 127:34–50.https://doi.org/10.1161/CIRCRESAHA.120.316574
-
Vista enhancer browser -- a database of tissue-specific human enhancersNucleic Acids Research 35:D88–D92.https://doi.org/10.1093/nar/gkl822
-
10 years of GWAS discovery: biology, function, and translationAmerican Journal of Human Genetics 101:5–22.https://doi.org/10.1016/j.ajhg.2017.06.005
-
Progressive action potential duration shortening and the conversion from atrial flutter to atrial fibrillation in the isolated canine right atriumJournal of the American College of Cardiology 38:1757–1765.https://doi.org/10.1016/s0735-1097(01)01606-0
Article and author information
Author details
Funding
CardioVasculair Onderzoek Nederland (Project 2014-18 CONCOR-genes Young Talent Program)
- Fernanda M Bosada
Fondation Leducq (14CVD01)
- Vincent M Christoffels
Dutch Cardiovascular Alliance (OUTREACH)
- Vincent M Christoffels
ZonMw (TOP 91217061)
- Vincent M Christoffels
Dutch Research Council (OCENW.GROOT.2019.029)
- Vincent M Christoffels
The funders had no role in study design, data collection and interpretation, or the decision to submit the work for publication.
Acknowledgements
We thank Berend de Jonge for technical assistance. This work was supported by CardioVasculair Onderzoek Nederland (CVON) project 2014–18 CONCOR-genes Young Talent Program (to FMB), CVON project 2014–18 CONCOR-genes (to VMC), Leducq Foundation 14CVD01 (to VMC), Dutch CardioVascular Alliance OUTREACH (to VMC) and ZonMW TOP 91217061 (to VMC).
Ethics
Housing, husbandry, all animal care and experimental protocols were in accordance with guidelines 310 from the Directive 2010/63/EU of the European Parliament and Dutch government. Protocols were 311 approved by the Animal Experimental Committee of the Amsterdam University Medical Centers. 312 Animal group sizes were determined based on previous experience.
Copyright
© 2023, Bosada et al.
This article is distributed under the terms of the Creative Commons Attribution License, which permits unrestricted use and redistribution provided that the original author and source are credited.
Metrics
-
- 1,143
- views
-
- 183
- downloads
-
- 8
- citations
Views, downloads and citations are aggregated across all versions of this paper published by eLife.
Citations by DOI
-
- 8
- citations for umbrella DOI https://doi.org/10.7554/eLife.80317