TRF1 averts chromatin remodelling, recombination and replication dependent-break induced replication at mouse telomeres
Abstract
Telomeres are a significant challenge to DNA replication and are prone to replication stress and telomere fragility. The shelterin component TRF1 facilitates telomere replication but the molecular mechanism remains uncertain. By interrogating the proteomic composition of telomeres, we show that mouse telomeres lacking TRF1 undergo protein composition reorganisation associated with the recruitment of DNA damage response and chromatin remodellers. Surprisingly, mTRF1 suppresses the accumulation of promyelocytic leukemia (PML) protein, BRCA1 and the SMC5/6 complex at telomeres, which is associated with increased Homologous Recombination (HR) and TERRA transcription. We uncovered a previously unappreciated role for mTRF1 in the suppression of telomere recombination, dependent on SMC5 and also POLD3 dependent Break Induced Replication at telomeres. We propose that TRF1 facilitates S-phase telomeric DNA synthesis to prevent illegitimate mitotic DNA recombination and chromatin rearrangement.
Introduction
Telomeres are specialised nucleoprotein structures at the ends of chromosomes, composed of repetitive sequences (TTAGGG repeats in mammals) (Moyzis et al., 1988), long non-coding RNA called TERRA and six associated proteins, TRF1, TRF2, POT1a/b, RAP1 and TIN2, that form the shelterin complex (de Lange, 2005). These capping structures have the crucial function of maintaining genome stability by protecting the chromosome end from being recognised as DNA double strand breaks (DSBs) (Palm and de Lange, 2008). They also represent challenging structures for the replication machinery, which is associated to telomere fragile sites (Martínez et al., 2009; McNees et al., 2010; Sfeir et al., 2009; Vannier et al., 2012). Telomere fragility is identified by the formation of multitelomeric signals (MTS), where telomeres appear as broken or decondensed, resembling the common fragile sites (CFS) observed at non telomeric loci after treatment with aphidicolin (APH). TRF1 facilitates the progression of the replication fork at telomeres, by recruiting a specialised DNA helicase BLM, which in turn resolve secondary structures, similar to fission yeast ortholog Taz1 (Lee et al., 2018; Martínez et al., 2009; Miller et al., 2006; Sfeir et al., 2009).
During tumorigenesis, cancer cells can achieve replicative immortality by activation of telomere maintenance mechanisms. The majority of cancer cells reactivate telomerase, while a minority (10–15%) uses an alternative mechanism named ALT for alternative lengthening of telomeres (Bryan et al., 1997; Kim et al., 1994). Intriguingly, ALT is characterised by the appearance of ALT-associated PML bodies (APBs), specialised sites where a subset of telomeres co-localises with PML protein and several DNA repair and homologous recombination (HR) proteins (Draskovic et al., 2009; Wu et al., 2000; Yeager et al., 1999). ALT telomeres can be maintained by more than one mechanism of recombination. Indeed, in yeast, two different ALT-like pathways have been described: type I and type II, both dependent on Rad52. While Type I requires Rad51 to mediate the invasion of a homologous sequence, Type II is Rad51 independent but Rad50 (of the MRX/N complex) dependent. Both Type I and II mechanisms require the DNA polymerase Pol32, which initiates DNA synthesis for several kilobases, in a process known as Break Induced Replication (BIR) (Ira and Haber, 2002). Recently, multiple groups have revisited this Rad51 independent DNA synthesis repair pathway at mammalian ALT telomeres (Dilley et al., 2016; Garcia-Exposito et al., 2016; Roumelioti et al., 2016). Mammalian BIR is dependent on POLD3 and POLD4, subunits of DNA polymerase delta and orthologs of yeast Pol32. ALT cells present increased DNA damage response (DDR) and several studies have underlined the contribution of replication stress to ALT-mediated telomere extension (Arora et al., 2014; Cox et al., 2016; Pan et al., 2017). However, the molecular mechanisms initiating recombination in ALT cells are still unclear.
In order to gain insight into the chromatin composition of telomeres undergoing replication stress, we performed Proteomics of Isolated Chromatin segments (PICh), using TRF1 conditional knock-out Mouse Embryonic Fibroblasts (MEFs, telomerase positive). Surprisingly, we found that telomeres lacking TRF1 are enriched in SMC5/6, DNA polymerase δ (POLD3), and chromatin remodelling factors known to be associated with ALT telomeres. These cells also present additional DNA damage and recombination hallmarks such as formation of APBs, mitotic DNA synthesis at telomeres, a feature of BIR and increased TERRA levels. Further investigation using specific shRNAs against the SMC5/6 complex or POLD3 revealed how these two complexes are key regulators of the recombination signature identified in TRF1 deleted cells. Taken together, these results strongly identify TRF1 as a central player in preserving telomeric chromatin against HR, induced by DNA replication stress, and particularly POLD3 dependent-mitotic DNA synthesis.
Results
Capture of TRF1 depleted telomeres by PICh reveals drastic changes in the chromatin composition
To isolate and identify the chromatin composition of TRF1 depleted telomeres, we employed Proteomics of Isolated Chromatin segments (PICh), a powerful and unbiased technique that uses a desthiobiotinylated oligonucleotide complementary to telomeric repeat sequences to specifically pull down telomeric chromatin (Déjardin and Kingston, 2009). We performed PICh in MEFs harboring a TRF1 conditional allele. MEFs lacking TRF1 are well known to undergo replicative stress; however, they can grow for up to 8 days before entering senescence, making them optimal for investigating replication stress at telomeres (Martínez et al., 2009; Sfeir et al., 2009). Cells were transduced twice (day 0 and 3) with a CRE or GFP control adenovirus and collected 7 days after the first transduction, as indicated in the timeline (Figure 1A). Excision of exon 1 of TRF1 by CRE recombinase (Sfeir et al., 2009) resulted in the expected loss of TRF1 protein as determined by immunoblotting and deficient cells do not show drastic change in the cell cycle distribution (Figure 1—figure supplement 1). Cells were fixed and isolation of telomeres was performed using a probe complementary to TTAGGG repeats or a scrambled probe as a negative control. Finally, telomeric chromatin was isolated from both control cells (wt) and TRF1 deleted cells before mass spectrometry identification (Figure 1C). We identified a list of 1306 proteins that was subjected to refinement in order to remove unspecific bound proteins or contaminants found with the scrambled probe (see experimental procedure for detailed description). Based on the analysis of label free quantification (LFQ intensities), we found 119 proteins presenting a gain of abundance at TRF1 depleted telomeres (Log2<-2) and 206 factors were displaced from these telomeres (Log2>-2), considering that a cut-off for differential expression is set to log2 fold change (TRF1deletion/wt)> |2| and -Log (p-value) > 1 (Figure 1D). Amongst these 206 proteins, we found TRF1, as expected due to the knock-out of its gene, but also one component of the CST complex (CTC1), important player in the efficient restart of stalled replication forks at telomeres (Gu et al., 2012) and recruited through POT1b interaction (Wu et al., 2012). Interestingly, POT1b is also less abundant at TRF1 depleted telomeres (Figure 1D–E). On the other end, the group of 119 proteins enriched in TRF1 deleted cells includes several factors involved in structural maintenance of chromosomes (SMC), HR and DNA damage response (Figure 1D–E–F), such as the MRN complex (MRE11, RAD50 and NBS1). The identification of 53BP1 recruited to TRF1 depleted telomeres (Figure 1D–F) acts as a positive marker for the specificity of this proteomic analysis, as reported before in Martínez et al. (2009); Sfeir et al. (2009). Moreover, we could identify drastic and previously uncharacterised changes of the telomeric proteome at telomeres undergoing replication stress presented hereafter.
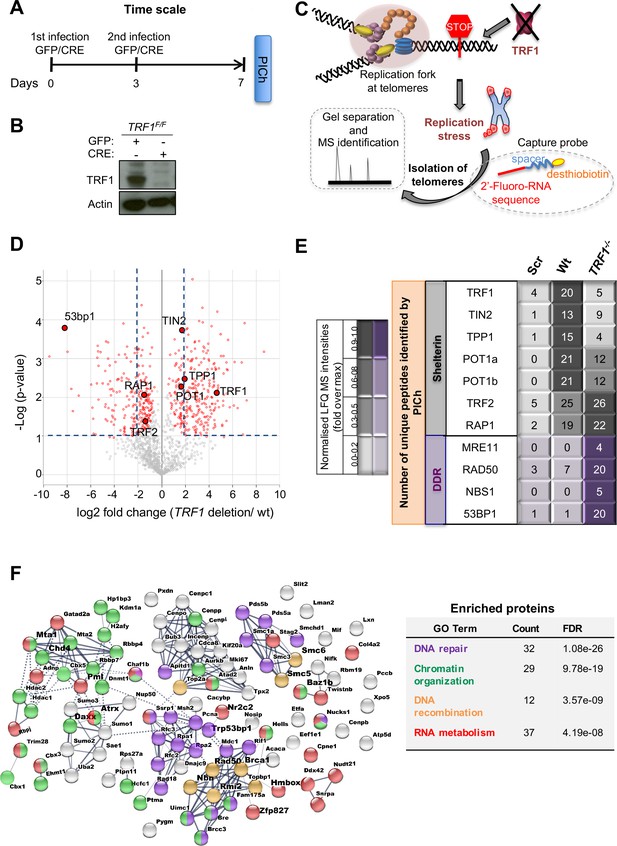
Proteomics of isolated chromatin segments (PICh) of TRF1 depleted mouse telomeres.
(A) Overview of experimental timeline aimed at performing PICh experiment after induction of TRF1 deletion. TRF1F/F MEFs were infected twice (day 0 and 3) with adenovirus containing either GFP-control or CRE and collected at day 7 for PICH experiments. (B) Western blot showing deletion of TRF1 in MEFs after infections with CRE Adenovirus, at day 7 as in A. (C) Schematic representation of the PICh analysis performed to detect chromatin changes occurring at telomeres upon TRF1 deletion. (D) Volcano Plot based on LFQ intensities of proteins. Cut off for differential expression were set to log2 fold change (TRF1deletion/wt)> |2| and -Log (p-value) > 1. (E) Table listing shelterin components and some of the DNA damage response (DDR) factors identified. The corresponding number of unique peptide isolated is indicated for each factor of interest. Relative LFQ intensity abundance profiles were visualised in the form of a heat-map, by scaling each protein intensity to the maximum intensity across conditions. Light to darker colours indicate increasing relative protein abundance. (F) Connectivity map for proteins recruited at telomeres upon TRF1 deletion using string-db.org software. Solid lines, represents strong direct interactions, while dashed lines represent no evidence for direct interaction. In violet, DNA damage and repair proteins; in orange, factors belonging to DNA repair specifically involved in DNA recombination process; while in green and red, important factors for chromosome maintenance and factors involved in RNA metabolism, respectively. Source data are provided as a Source Data File.
-
Figure 1—source data 1
TRF1F/F MEFs western blot.
Related to Figure 1B.
- https://cdn.elifesciences.org/articles/49817/elife-49817-fig1-data1-v4.xlsx
-
Figure 1—source data 2
PICh data (TRF1F/F telo vs TRF1-/- telo).
Related to Figure 1D.
- https://cdn.elifesciences.org/articles/49817/elife-49817-fig1-data2-v4.xlsx
-
Figure 1—source data 3
PICh data.
Related to Figure 1F.
- https://cdn.elifesciences.org/articles/49817/elife-49817-fig1-data3-v4.xlsx
TRF1 suppresses APBs formation and HR at telomeres
Interestingly, TRF1 deficient MEFs present a telomeric enrichment for factors involved in HR and chromatin remodelling (BRCA1, PML, SMC5/6, ATRX and, NurD associated factors) that are usually abundant at ALT telomeres (Figure 2A) (Conomos et al., 2014; Draskovic et al., 2009; Marzec et al., 2015; Potts and Yu, 2007). To validate the specific association of some of these factors with TRF1 depleted telomeres in telomerase positive MEFs, we carried out chromatin immunoprecipitation (ChIP) experiments using ChIP-grade specific antibodies followed by telomeric dot-blot. TRF1 antibody was used as a negative control for our experiment, while the recruitment of BAZ1b, BRCA1 and some subunits of the nucleosome remodelling and deacetylase (NurD) complex (p66a, MTA1, ZNF827 and CHD4) was assessed. For all these factors, with the exception of p66a for which no statistical significance was achieved, we observed a specific enrichment at telomeres upon TRF1 deletion (Figure 2—figure supplement 1, A). In addition, to confirm the presence of PML at telomeres lacking TRF1, as suggested by our PICh data (Figure 2A), we performed immuno-FISH and scored for the formation of APBs. We observed a two-fold increase in the number of co-localisations between PML and telomeres in TRF1-/- MEFs compared to control cells (Figure 2C). To test if the recruitment of some of these factors is a consequence of chronic replication stress or if TRF1 directly inhibit these events, we treated wt MEFs with low doses of APH (0.4 µM) for 3 days. Using ChIP-dot blot, we did not detect the recruitment of any of the chromatin remodellers and DNA repair factors BRCA1, MTA1, CHD4, ZNF827 and BAZ1b, present at TRF1 depleted telomeres (Figure 2—figure supplement 1, B). Furthermore, we show that wt MEFs treated with APH do not form APBs despite a general increase in PML foci (Figure 2—figure supplement 1, C). Overall these data demonstrate that telomeres depleted of TRF1 engage chromatin remodelling associated with the formation of APBs, platform of recombination (Cesare and Reddel, 2010). Hence, to test if TRF1 is a suppressor of recombination, we revisited the incidence of telomeric sister chromatid exchanges (T-SCE) using chromosome orientation FISH (CO-FISH) in TRF1 deficient cells (Figure 2D). We identified an increase in T-SCE in TRF1-/- MEFs (2.8%) compared to control cells (0.4%) (Figure 2D). This result is at odds with previous publications where T-SCE events detected at TRF1 depleted telomeres were not significantly enriched, with only 1% of T-SCEs detected compared to 0.1% in wt cells (Martínez et al., 2009; Sfeir et al., 2009). In fact, this discrepancy might be explained by the difference in timing for the analysis of T-SCEs in TRF1 deficient cells. Both publications report the lack of recombination effect by T-SCEs at 3 or 4 days after TRF1 loss, while we generally carry our investigations at day 7. Therefore, we repeated the experiments in TRF1-/- cells at different time points post infection: day 4 and day 7, finding respectively 1.6% and 2.8% of T-SCEs per chromosome end (Figure 2—figure supplement 2, A-left graph), indicating a lower % of T-SCE events happening at earlier time point. A second distinct difference with previous reports is the type of telomere signal exchanges that we analysed. As in Sfeir et al. (2009), all types of telomere signal exchanges (e.g. the exchanges appearing at single chromatids and the reciprocal exchanges at both chromatids) were considered. However, Martínez et al. (2009) only refers to reciprocal exchanges at both chromatids. Thus, we next classified T-SCEs detected in TRF1 deficient MEFs into these two different types (single and double) and found that 4 days post infection only T-SCEs at single chromatids were significantly increased (Figure 2—figure supplement 2, A-right graph), while the reciprocal exchanges were not enhanced at TRF1 depleted telomeres (Figure 2—figure supplement 2, A-middle graph). Therefore, our detailed analysis of the nature and timing of T-SCEs in TRF1 deficient MEFs not only demonstrates an unappreciated role of TRF1 in suppressing HR, but also suggests that single chromatid exchanges, characteristic of conservative DNA synthesis – Break Induced Replication (Roumelioti et al., 2016), are the initial events arising in TRF1-/- MEFs. To further discriminate between suppression of recombination by TRF1 and replication-dependent recombination, we quantified T-SCEs in mouse cells treated with APH. Like for TRF1-depleted telomeres, we also detected an increase in single exchanges in APH-treated MEFs (Figure 2—figure supplement 2, B-right panel). The absence of reciprocal exchanges between telomere chromatids indicates that APH-dependent replication stress does not trigger an HR repair but only BIR, albeit we cannot completely exclude that longer replication stress could lead to HR (Figure 2—figure supplement 2, B-left panel). Overall our data highlights the specific function of TRF1 in suppressing chromatin remodeling, APBs and HR.
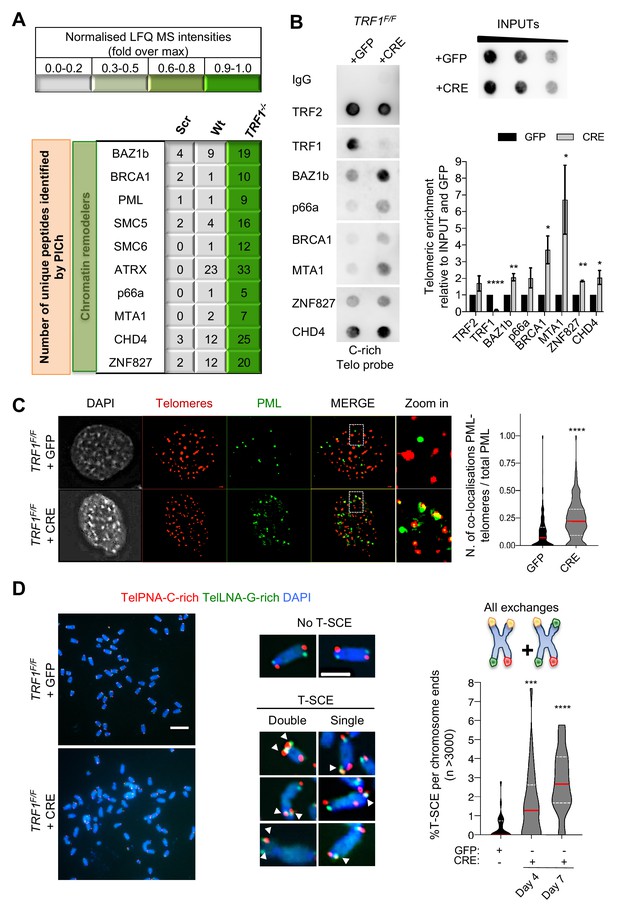
Recombination factors are recruited at TRF1 depleted telomeres.
(A) Table listing chromatin remodellers identified. The corresponding number of unique peptide isolated is indicated for each factor of interest. Same as in Figure 1, light to darker colours indicate increasing relative protein abundance. (B) Validation of chromatin remodeller factors by ChIP-dot blot analysis in wt (+GFP) and TRF1-/- (+CRE) conditions using ChIP grade antibodies against chosen factors after chromatin preparation from MEFs. The blot was revealed with a DIG-Tel-C-rich probe. ChIP signals were normalised to DNA input and GFP control. Data are represented as telomeric enrichment of proteins relative to GFP ± SEM of at least three independent biological replicates. P values, two-tailed student t-test (*, p<0.05; **, p<0.01; ****, p<0.0001). (C) Representative image of Immunofluorescence showing co-localisation of Telomeres (red) with PML (green) in MEFs nuclei (DAPI) treated with GFP and CRE. Data from two independent biological replicates are represented as number of Telomeres-PML co-localising foci divided by the total number of PML present per nucleus (n = 300 nuclei) with a violin plot, where the median is underlined in red and quartiles in white. P values, two-tailed student t-test (****, p<0.0001). Source data are provided as a Source Data File. (D) Representative images of the chromosome-oriented CO-FISH assay with denaturation, used to score for telomeric T-SCEs in TRF1F/F MEFs infected with GFP or CRE. Telomeres are labelled with TelPNA-C-rich-Cy3 (red) and TelLNA-G-rich-FAM (green), while chromosomes are counterstained with DAPI (blue). Scale bar, 10 µm. Enlarged intersections show the difference between a chromosome with No T-SCE (top) and a chromosome with T-SCE (bottom). T-SCE images show double T-SCEs (left) and single chromatid events (right). Scale bar, 2 µm. For quantification, T-SCE was considered positive when involved in a reciprocal exchange of telomere signal with its sister chromatid (both telomeres yellow) and for asymmetrical exchanges at single chromatid (one telomere yellow). Data are indicated as % of T-SCE per sister telomere (n = > 3000 chromsome ends) and are represented with a violin plot, where the median is underlined in red and quartiles in white. P value, two-tailed student t-test (****, p<0.0001).
-
Figure 2—source data 1
Control specificity of proteomic binding (TRF1-/- telo vs TRF1-/- scbl).
Related to Figure 2A.
- https://cdn.elifesciences.org/articles/49817/elife-49817-fig2-data1-v4.xlsx
-
Figure 2—source data 2
ChIP quantification in TRF1F/F MEFs.
Related to Figure 2B.
- https://cdn.elifesciences.org/articles/49817/elife-49817-fig2-data2-v4.xlsx
-
Figure 2—source data 3
Quantification of APBs in TRF1F/F MEFs.
Related to Figure 2C.
- https://cdn.elifesciences.org/articles/49817/elife-49817-fig2-data3-v4.xlsx
-
Figure 2—source data 4
T-SCEs quantification in TRF1F/F MEFs.
Related to Figure 2D.
- https://cdn.elifesciences.org/articles/49817/elife-49817-fig2-data4-v4.xlsx
TRF1 depletion causes TERRAs upregulation in mouse cells
Since depleting telomeres of TRF1 induces chromatin remodelling, formation of APBs and increase of HR, we next decided to revisit the effect of TRF1 on TERRA molecules, which are proposed to regulate telomere recombination (Yu et al., 2014). Previous studies have reported in-vivo interactions between TRF1 and TERRA (Deng et al., 2009) and also a possible transcriptional regulation by TRF1 through a mechanism involving RNA polymerase II - TRF1 interaction (Schoeftner and Blasco, 2008). However, the role of TRF1 regulating telomere transcription appears complex since contrasting results have been reported by different groups in both human and mouse cell lines (Lee et al., 2018; Schoeftner and Blasco, 2008; Sfeir et al., 2009). We performed both RNA dot-blot and Northern-blot analyses showing a significant increase in TERRA molecules upon loss of TRF1 in immortalised MEFs, 7 days after transduction (Figure 3A–B) but also at earlier time point (day 4) and in primary MEFs (Figure 3—figure supplements 1, A–B–C). Collectively, we identify an increase in TERRA molecules upon TRF1 removal from telomeres, which suggests a TRF1 function in suppressing mouse TERRAs. Particularly, we noticed that these TERRAs have high molecular weight and can only be detected when an alkaline treatment is performed during Northern-blotting (Figure 3—figure supplement 1, D). In addition, we carried out TERRA-FISH (Figure 3C) in MEFs deficient for TRF1, confirming a significant increase in numbers and intensity of TERRA foci per nucleus (Figure 3C). To determine whether this phenotype is a direct consequence of replication stress, MEFs were treated with low doses of APH and TERRA analysis was carried out by RNA dot-blot and Northern blot. Similar to TRF1 depleted cells, APH-treated MEFs exhibited a significant increase in TERRAs (Figure 3—figure supplement 1, E–F). Taken together, these results suggest that TRF1-dependent replication stress enhances telomere transcription or TERRAs stability.
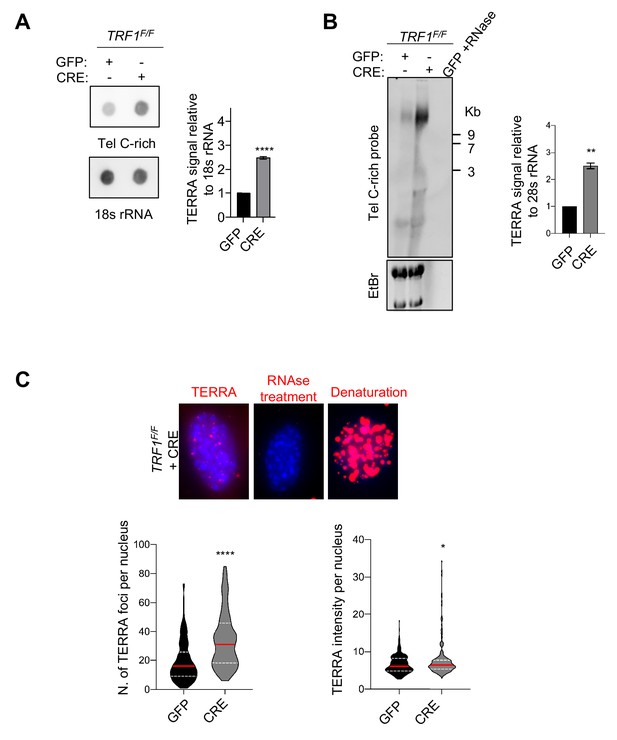
TRF1 depletion causes TERRAs upregulation.
(A) RNA dot blot analysis in wt and TRF1 deleted MEFs. The blot was revealed with a DIG-Tel-C-rich probe or 18 s rRNA as a control. TERRA signals were normalised to 18 s rRNA and GFP control ± SEM of at least three independent biological replicates. P values, two-tailed student t-test (****, p<0.0001). (B) TERRA detection by Northern blotting upon TRF1 deletion. The blot was revealed with a DIG-Tel-C-rich probe (upper part). Ethidium bromide (EtBr) staining (bottom) of rRNAs was used as loading control. TERRA signals were normalized to 28 s rRNA signal from EtBr staining ± SEM of 2 independent biological replicates. P values, two-tailed student t-test (**, p<0.01). (C) Representative images of TERRA-FISH experiment (top panel) showing the difference between cells stained with TERRA (red), negative control with RNAse A treatment and positive control after denaturation. TERRA-FISH quantification (bottom panel) in wt (+GFP) and TRF1-/- (+CRE) conditions. Violin plots are representing the number of TERRA foci (left) and TERRA intensity (right) (n = 250) per nucleus, where the median is underlined in red and quartiles in white, two-tailed student t-test (****, p<0.0001); Mann-Whitney test used for TERRA intensity quantification (*, p<0.05).
-
Figure 3—source data 1
Quantification of Telomeric RNA molecules by dot-blot in TRF1F/F MEFs.
Related to Figure 3A.
- https://cdn.elifesciences.org/articles/49817/elife-49817-fig3-data1-v4.xlsx
-
Figure 3—source data 2
Quantification of TERRAs by Northern.
Related to Figure 3B.
- https://cdn.elifesciences.org/articles/49817/elife-49817-fig3-data2-v4.xlsx
-
Figure 3—source data 3
Quantification of number of TERRA foci.
Related to Figure 3C.
- https://cdn.elifesciences.org/articles/49817/elife-49817-fig3-data3-v4.xlsx
mTRF1-dependent APBs formation and BIR are conserved in human cells
The presence of APBs, BIR, HR and TERRAs, in TRF1-/- MEFs indicates some similarities with ALT telomeres. However, the absence of telomere heterogeneity, c-circle formation and still presence of telomerase activity (Figure 3—figure supplements 2, A–B–C) also suggest that this ALT-like phenotype is not complete. To test whether the function of TRF1 in suppressing these ALT-like features is conserved in human cells, we used small RNA interference against TRF1 in HT1080-ST cells (long telomeres), performing all the analysis 6 days post-transfection (Figure 4A). TRF1-depleted HT1080-ST cells present a modest but significant increase in APBs formation (Figure 4B). This phenotype appears to be milder as compared to TRF1-/- MEFs, probably because HT1080/ST present higher levels of APBs compared to other human (Pickett et al., 2009) or mouse cells (Figure 2C). We conclude that TRF1 function is somewhat maintained between the two species. To assess the human TRF1 function in suppressing HR and BIR, we measured sister chromatid exchanges at telomeres in HT1080-ST depleted for TRF1 (Figure 4C). We observed a significant increase in single exchanges (Figure 4C-right panel) however, we could not detect double exchanges in cells transfected with TRF1 siRNA compared to the ctl (Figure 4C-left panel). Finally, TERRA expression was analysed by DNA dot blot and no differences were observed between ctr and TRF1 depleted cells (Figure 4—figure supplement 1, A). To evaluate whether long term depletion of TRF1 in human cells would cause any increase in TERRA expression, as observed in TRF1-/- MEFs, we used a doxycycline inducible TRF1 CRISPR/Cas9 HeLa cell line (Figure 4—figure supplement 1, B) (Kim et al., 2017). However, even 15 days after hTRF1 deletion, no significant increase in TERRAs was detected. Human TRF1 KO cells did not show any growing defects after 15 days in culture, which is remarkably different from TRF1 deficient MEFs that fail to proliferate due to a rapid induction of senescence (Karlseder et al., 2003; Martínez et al., 2009).
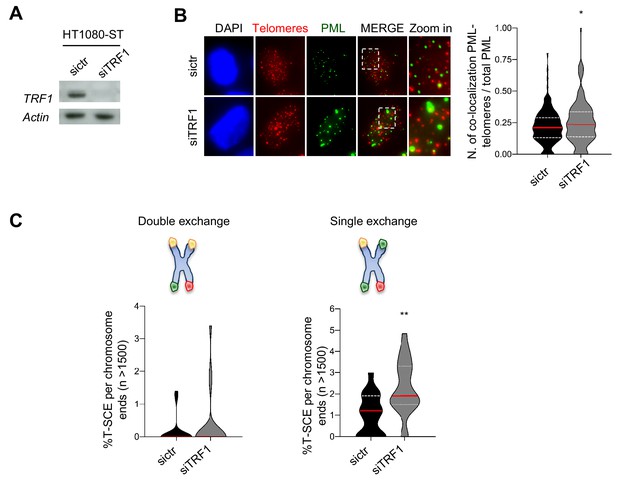
Human TRF1 suppresses APBs formation and BIR.
(A) Western blotting showing expression of TRF1 and Actin (loading control) proteins in human HT1080-ST cell line after depletion of TRF1 with siRNAs (6 days post transfection). sictr is used as negative control for transfection. (B) Representative image of Immunofluorescence showing co-localisation of Telomeres (red) with PML (green) in HT1080-ST nuclei (DAPI) transfected with sictr or siTRF1. Data are represented as number of Telomeres-PML co-localising foci divided by the total number of PML present per nucleus (n = 150 nuclei) and are shown as a violin plot, where the median is underlined in red and quartiles in white. P values, two-tailed student t-test (*, p<0.05). Source data are provided as a Source Data File. (C) Quantification of telomeric T-SCEs in HT1080-ST transfected with sictr or siTRF1. Telomeric exchanges are classified as double exchanges (reciprocal, both chromatids-left graph) and single exchanges (asymmetrical, single chromatid-right graph). Violin plots represent % of T-SCE per chromosome ends (n = at least 1500 events were scored), where the median is underlined in red and quartiles in white. P value, two-tailed student t-test (**, p<0.01).
-
Figure 4—source data 1
KD of TRF1 in HT1080-ST cells.
Related to Figure 4A.
- https://cdn.elifesciences.org/articles/49817/elife-49817-fig4-data1-v4.xlsx
-
Figure 4—source data 2
Quantification of APBs in HT1080-ST TRF1 KD.
Related to Figure 4B.
- https://cdn.elifesciences.org/articles/49817/elife-49817-fig4-data2-v4.xlsx
-
Figure 4—source data 3
T-SCEs quantification in HT1080-ST TRF1 KD.
Related to Figure 4C.
- https://cdn.elifesciences.org/articles/49817/elife-49817-fig4-data3-v4.xlsx
In conclusion, some of TRF1 functions are conserved between mouse and human. For both species, TRF1 suppresses telomere fragility and therefore is essential to facilitate DNA replication through telomeric repeats (Lee et al., 2018; Sfeir et al., 2009). We now show that like for mouse TRF1, its human homolog is essential to suppress APBs formation and BIR, which is likely responsible for the rescue of stalled/collapsed telomeric forks.
TRF1 suppresses mitotic DNA synthesis at telomeres
Since the denaturing CO-FISH experiments in TRF1 deficient cells identified single chromatid exchanges that are proposed to be reminiscent of BIR events, an HR alternative pathway required in G2-M phase (Roumelioti et al., 2016), we tested whether TRF1 depleted telomeres trigger non S-phase DNA synthesis. We performed a pulse with 5-bromo-2-deoxyuridine (BrdU) for 2 hr (Figure 5A) before carrying out BrdU immunofluorescence at telomeres in interphase cells (Figure 5B–C). Only non S-phase cells were counted in this experiment, based on the formation of clear BrdU foci (Dilley et al., 2016; Nakamura et al., 1986) (Figure 5B). TRF1-/- MEFs display elevated BrdU incorporation at telomeres, showing two-times more telomere synthesis compared to control cells (Figure 5C). To investigate DNA synthesis happening exclusively in mitosis, so-called MiDAS (Minocherhomji et al., 2015), we performed a similar experiment in metaphases. After incubating wt and TRF1 deficient MEFs with 5-ethynyl-2-deoxyuridine (EdU) and colcemid for 1 hr, mitotic cells were collected to analyse EdU incorporation on metaphase chromosomes (Figure 5A). We scored for telomeric and non-telomeric EdU foci (mitotic DNA synthesis) and found that CRE induced cells had a significant increase in telomeric mitotic DNA synthesis compared to the GFP control cells (Figure 5D). This result confirms that TRF1 depleted telomeres present an increased level of non-S-phase DNA synthesis, similar to what is observed in ALT cells. In addition, analysis of EdU incorporation in metaphase spreads allowed us to distinguish between conservative BIR associated DNA synthesis and HR semi-conservative DNA synthesis (Min et al., 2017). In the first case, EdU would be labelled on a single chromatid (Figure 5E upper panel), while in the latter, EdU would localise to both chromatids (Figure 5E bottom panel). Thus, to assess the mechanism of DNA synthesis in TRF1 deleted cells, the pattern of EdU incorporation on metaphase chromosomes was further investigated (Figure 5F). Non-telomeric (upper panel) and telomeric (middle panel) EdU foci formed mainly on a single chromatid. In fact, 72% of the mitotic DNA synthesis at non-telomeric sites localised to a single chromatid, while the remaining 28% of the signal was present at both chromatids (Figure 5F, upper panel). This result is even more striking when EdU signal was restricted to telomeres, with almost all the co-localisation being present at single chromatids (95%). These observations suggest that TRF1 is crucial for the suppression of mitotic DNA synthesis mediated by BIR at telomeres.
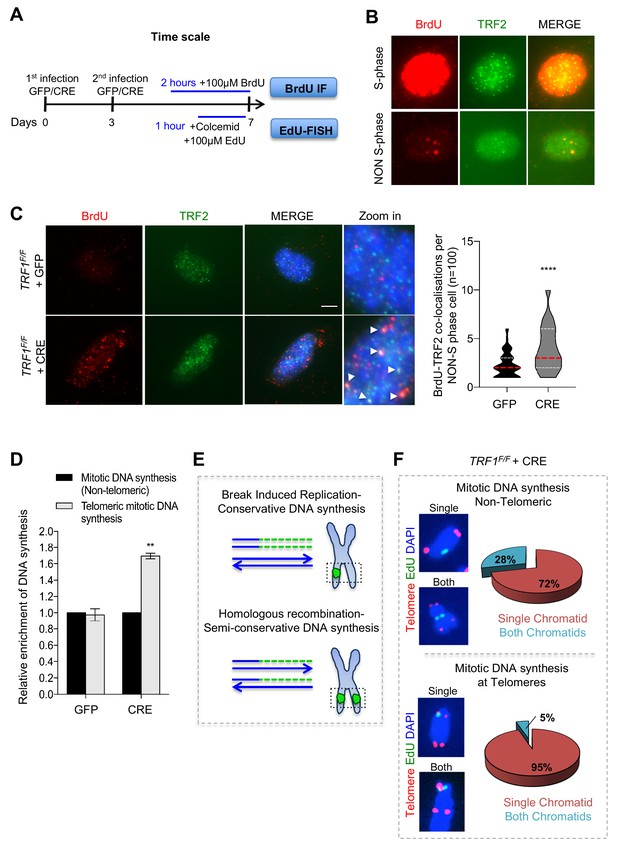
Deletion of TRF1 induces mitotic DNA synthesis at telomeres.
(A) Schematic overview of the experimental timeline. TRF1F/F MEFs cells were infected twice (day 0 and 3) with adenovirus containing either GFP control or CRE to mediate TRF1 deletion. Prior to collection at day 7, cells were treated with either BrdU (100 µM) for 2 hr or EdU (100 µM) + colcemid for 1 hr, to perform respectively BrdU-Immunofluorescence (IF) or EdU-FISH on metaphases. (B) Representative image of BrdU (red) - TRF2 (green) immunofluorescence showing example of cells in S-phase (upper panel) and non-S-phase (bottom panel). (C) Immunofluorescence showing co-localisation of BrdU (red) with TRF2 (green) in TRF1F/F MEFs nuclei (DAPI, blue) treated with GFP and CRE. Scale bar, 5 µm (denaturing conditions). Data are represented in a violin plot as % of cells in non-S-phase showing BrdU-TRF2 co-localising foci (n = 100 nuclei), where the median is underlined in red and quartiles in white, two-tailed student t-test (****, p<0.0001). (D) Quantification of DNA synthesis using the number of EdU-positive intra-chromosomes or telomeres in TRF1F/F cells infected with GFP and CRE relative to the GFP control (n = 50 metaphases). Data are represented as relative enrichment to the GFP control ± SEM of 3 independent biological replicates. P values, two-tailed student t-test (**, p<0.01). (E) Schematic representation of Break Induced Replication (top part) with single EdU foci at a single chromatid and Homologous recombination (bottom part) with EdU foci at both chromatids. (F) Analysis of DNA synthesis in TRF1 deleted cells. Upper panel: Non-telomeric mitotic DNA synthesis. Representative images showing EdU signal (green) in a single chromatid or in both chromatids. Pie chart representing % of chromosomes having EdU signal at a single chromatid or at both chromatids. Bottom panel: Telomeric mitotic DNA synthesis. Representative images showing EdU signal (green) at telomeres (red) at single or both chromatids. Pie chart representing % of chromosomes having EdU signal at telomeres at a single chromatid or both chromatids. Source data are provided as a Source Data File.
-
Figure 5—source data 1
Quantification of BrdU-TRF2 co-localisation in S and non-S nuclei.
Related to Figure 5C.
- https://cdn.elifesciences.org/articles/49817/elife-49817-fig5-data1-v4.xlsx
-
Figure 5—source data 2
Quantification of Mitosis DNA synthesis in TRF1F/F MEFs and at telomeres.
Related to Figure 5D and F.
- https://cdn.elifesciences.org/articles/49817/elife-49817-fig5-data2-v4.xlsx
Mitotic DNA synthesis at replication stressed telomeres is POLD3 dependent
BIR is a recombination dependent process reinitiating DNA replication when one end of a chromosome shares homology with the template DNA, leading to conservative DNA synthesis, which is dependent on RAD52 and POLD3 (pol32 homolog in yeast) (Bhowmick et al., 2016; Sotiriou et al., 2016). ALT telomeres have recently been reported to be elongated by BIR, in a POLD3 and SMC5-dependent manner (Dilley et al., 2016; Min et al., 2017; Potts et al., 2006). Since the SMC5/6 complex was exclusively enriched in PICh purified TRF1 depleted telomeres (Figure 2A), we further investigated the role of POLD3 and SMC5 in BIR DNA synthesis observed in TRF1-/- MEFs. We generated TRF1 F/F cells deficient in SMC5 or POLD3 using specific shRNAs. Upon infection with GFP or CRE adenovirus, we produced respectively single or double deletion TRF1-SMC5 or TRF1-POLD3 cell lines. Loss of SMC5 and TRF1 expression were confirmed by immunoblotting (Figure 6A–B), while mRNA levels of POLD3 were analysed by RT-QPCR (Figure 6C). We only noticed a slight decrease in population doublings in the double mutants, while all cell lines were still able to properly divide and incorporate EdU (Figure 6—figure supplement 1, A–B). Thus, we carried out EdU-FISH in these cells to check for the presence of BIR (Figure 6D). We found that the enrichment of DNA synthesis at telomeres in TRF1 deleted cells was suppressed in the double mutant TRF1-POLD3, while the double mutant TRF1-SMC5 revealed similar telomeric DNA synthesis when compared to the single TRF1 mutant (Figure 6E). First, these results confirm that BIR is the molecular mechanism taking place at TRF1 depleted telomeres. Second, SMC5 appears to be dispensable for BIR dependent DNA synthesis at these replication-stressed chromosome ends.
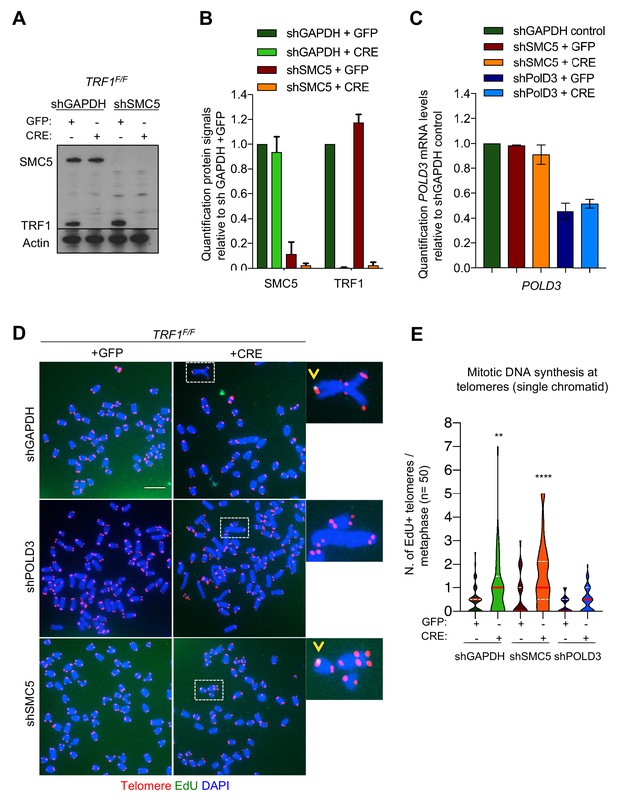
POLD3 but not SMC5 regulates mitotic DNA synthesis at TRF1 deleted telomeres.
(A) Western blotting showing expression of SMC5, TRF1 and Actin (loading control) proteins in TRF1F/F MEFs after infection with GFP or CRE-Adenovirus and deletion of SMC5 by shRNA. shGAPDH is used as negative control. (B) Quantification of the knock-out and knock-down shown in A. Graph shows protein signal quantification relative to shGAPDH in +GFP control cells, data are represented as mean ± SEM of 3 independent biological replicates. (C) Quantification of POLD3 mRNA levels relative to GAPDH control. Data are represented as mean ± SEM of 3 independent biological replicates. (D) Representative images of 6 different genotypes generated in the above description. Metaphases show EdU (green), telomeres labelled with TelPNA-C-rich-Cy3 (red) and chromosomes counterstained with DAPI (blue). Scale bar, 10 µm. (E) Quantification of mitotic DNA synthesis at telomeres (single chromatid) in TRF1F/F MEFs infected with shGAPDH control (GFP or CRE), shSMC5 (GFP or CRE) and shPOLD3 (GFP or CRE). Data are represented (n = 50 metaphases) as number of EdU positive telomeres per metaphase with a violin plot, where the median is underlined in red and quartiles in white. One-way ANOVA multiple comparisons (**, p<0.01; ****, p<0.0001) relative to shGAPDH+GFP sample. Source data are provided as a Source Data File.
-
Figure 6—source data 1
WB and Quantification of SMC5 knock-down efficiency in TRF1F/F MEFs.
Related to Figure 6A and B.
- https://cdn.elifesciences.org/articles/49817/elife-49817-fig6-data1-v4.xlsx
-
Figure 6—source data 2
POLD3 mRNA levels after KD in TRF1F/F MEFs.
Related to Figure 6C.
- https://cdn.elifesciences.org/articles/49817/elife-49817-fig6-data2-v4.xlsx
-
Figure 6—source data 3
Quantification of Mitosis DNA synthesis at telomeres in TRF1F/F MEFs with and without POLD3 and SMC5.
Related to Figure 6D and E.
- https://cdn.elifesciences.org/articles/49817/elife-49817-fig6-data3-v4.xlsx
SMC5 and POLD3 are required for APBs formation and recombination at TRF1 deficient telomeres
We further examined whether POLD3 and SMC5 could be responsible not only for the BIR dependent DNA synthesis but also for the other ALT-like phenotypes observed at TRF1 deficient telomeres.
Since TRF1 is well known to suppress telomere fragility or MTS (Sfeir et al., 2009) (Martínez et al., 2009), we first investigated the role of POLD3 and SMC5 in the induction or maintenance of this telomere replication stress in the double mutants (Figure 6—figure supplement 2, A–B). As previously reported, TRF1 depleted telomeres present approximately 20% of fragile telomeres per chromosomes (Figure 6—figure supplement 2, C). We could not detect any changes in the frequency of telomere fragility in TRF1-POLD3 nor TRF1-SMC5 mutants (Figure 6—figure supplement 2, C) suggesting that neither POLD3 nor SMC5 are involved in the mechanism that gives rise to telomere fragility. As APBs were increased in TRF1 deleted cells (Figure 2C), we investigated the roles of POLD3 and SMC5 in the formation of these specialised bodies. A significant reduction in number of cells having co-localising PML-telomere foci was detected in the double mutant cells TRF1-POLD3 and TRF1-SMC5 (Figure 7A) suggesting that POLD3 and SMC5 are necessary for the formation of these recombination machinery loci. We next explored the involvement of these two factors in HR by scoring for T-SCE (Figure 7B), discriminating also between the two categories of T-SCEs (single or double exchanges) in the analysis of the double mutants TRF1-SMC5 and TRF1-POLD3. We found that both types of exchanges are dependent on SMC5 and POLD3 (Figure 7—figure supplement 1). Finally, we assessed TERRA expression levels in the double mutants. Surprisingly, only the absence of POLD3 was able to rescue the increase in TERRA levels detected in TRF1 deficient cells, while the SMC5 single mutant increased TERRA expression (Figure 7C). Collectively, our data indicate that both POLD3 and SMC5 are essential for T-SCE and APBs formation, but only POLD3 is required to maintain increased TERRA levels and BIR observed in TRF1 deficient cells. This suggests that POLD3 and SMC5 have separate roles or act at different stages of the recombination events happening at TRF1 depleted telomeres, advocating also an intriguing connection between TERRA and BIR. We speculate that TERRA could trigger the homology search by stimulating the initial steps of BIR in which POLD3 is involved (Figure 8).
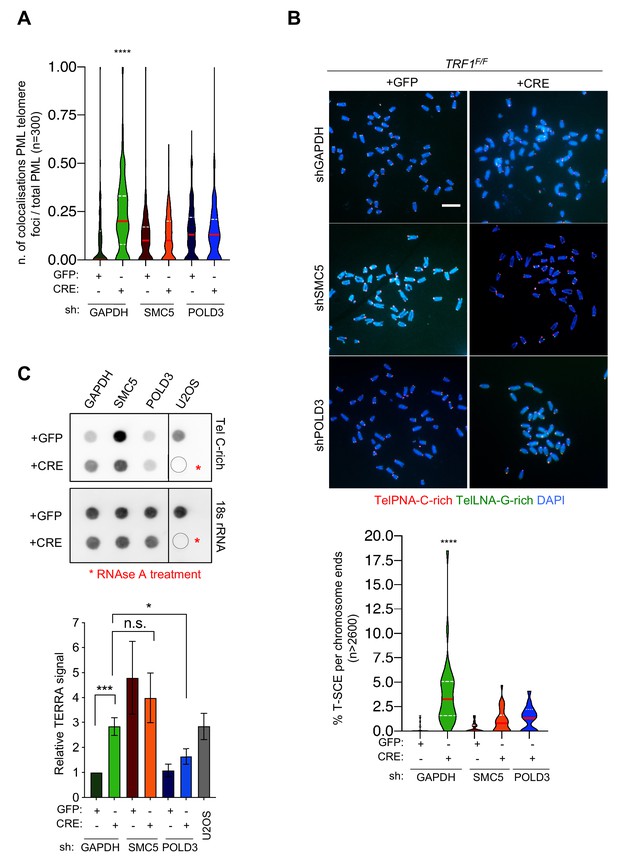
SMC5 and POLD3 are required for induction of recombination at TRF1 deficient telomeres.
(A) APBs formation in TRF1 deleted cells is rescued in double mutants TRF1-SMC5 and TRF1-POLD3. Quantification of APBs formation is represented as number of co-localising PML-telomere foci divided by the total number of PML present per nucleus (n = 300 nuclei analysed) from three independent biological replicates. Data are represented with a violin plot, where the median is underlined in red and quartiles in white. One-way ANOVA multiple comparisons (****, p<0.0001) relative to shGAPDH+GFP sample. (B) Representative images of the chromosome oriented (CO)-FISH assay with denaturation, used to score for telomeric T-SCEs in TRF1F/F MEFs infected with shGAPHH control (GFP or CRE), shSMC5 (GFP or CRE) and shPOLD3 (GFP or CRE). Telomeres are labelled with TelPNA-C-rich-Cy3 (red) and TelLNA-G-rich-FAM (green), while chromosomes are counterstained with DAPI (blue). Scale bar, 10 µm. For quantification T-SCE was considered positive when involved in a reciprocal exchange of telomere signal with its sister chromatid (both telomeres yellow) and for asymmetrical exchanges at single chromatid (one telomere yellow). Data are indicated as % of T-SCE per sister telomere (bottom panel). Data (n = > 2600 chromsome ends) from three independent biological replicates are indicated in a violin plot, where the median is underlined in red and quartiles in white. One-way ANOVA multiple comparisons (****, p<0.0001) relative to shGAPDH+GFP sample. (C) RNA dot blot analysis in TRF1, SMC5, POLD3 single and double mutants. The blot was revealed with a DIG-Tel-C-rich probe or 18 s rRNA as a control. TERRA signals were normalised to 18 s rRNA and GFP control (bottom panel). Data are represented as relative TERRA signal ± SEM of 4 independent biological replicates. P values, two-tailed student t-test (*, p<0.05; ***, p<0.001; n.s. = non significant). Source data are provided as a Source Data File.
-
Figure 7—source data 1
APBs co-localisations quantification in TRF1F/F MEFs with and without POLD3 and SMC5.
Related to Figure 7A.
- https://cdn.elifesciences.org/articles/49817/elife-49817-fig7-data1-v4.xlsx
-
Figure 7—source data 2
T-SCEs quantification in TRF1F/F MEFs with and without POLD3 and SMC5.
Related to Figure 7B.
- https://cdn.elifesciences.org/articles/49817/elife-49817-fig7-data2-v4.xlsx
-
Figure 7—source data 3
Quantification of Telomeric RNA molecules by dot-blot in TRF1F/F MEFs with and without POLD3 and SMC5.
Related to Figure 7C.
- https://cdn.elifesciences.org/articles/49817/elife-49817-fig7-data3-v4.xlsx
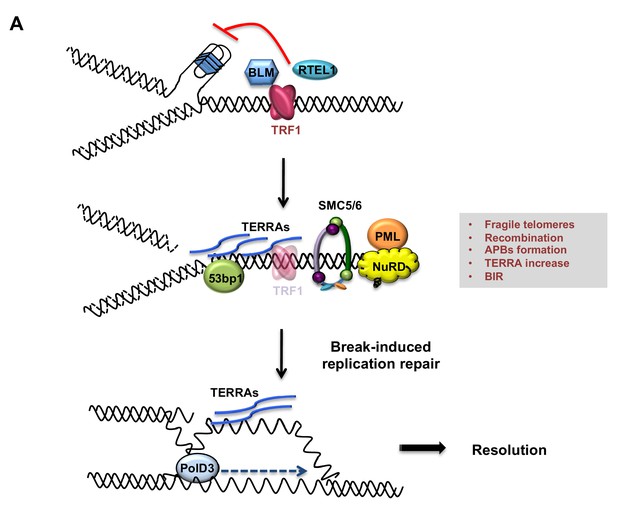
Model describing TRF1 as a negative regulator of telomeric transcription (TERRAs), APBs formation, telomeric recombination via PolD3-BIR dependent pathway.
Replicative stress induced by TRF1 deletion alters the chromatin status of these telomeres. Recruitment of chromatin remodellers/HR factors, TERRA accumulation and telomere fragility are observed. The SMC5/6 complex and polymerase POLD3 are among the factors recruited at replicative-stressed telomeres, representing the key players for APBs formation and telomere recombination, particularly BIR-mechanism. We propose that increased TERRAs molecules at telomeres could lead to increased R-loops, which are bypassed by POLD3 dependent BIR to resolve fork progression hindrance.
Discussion
Faithful DNA replication of genetic information is essential for the maintenance of genome stability and integrity. Specific genomic loci, including fragile sites and telomeres, represent major obstacles to DNA replication progression and/or completion. Fragile sites have the propensity to form visible gaps or breaks on chromosome in metaphase spreads of cell lines from patients having fragile X-syndrome or Huntington’s disease (reviewed in Minocherhomji and Hickson, 2014; Minocherhomji et al., 2015). It is well documented that CFS expression is exacerbated in cells grown under low to mild replication stress, for example upon inhibition of DNA polymerase with APH (Minocherhomji and Hickson, 2014; Minocherhomji et al., 2015). Fragile sites are hotspots for deletions, chromosome rearrangements and are associated with an increased frequency of homologous recombination (Glover and Stein, 1987). Over the last decade, telomeres have been identified as APH induced fragile sites displaying the standard phenotype of multiple spatially distinct telomere foci (MTS or telomere fragility) on metaphase spreads (Martínez et al., 2009; Sfeir et al., 2009). Various factors suppress MTS and thereby facilitate DNA replication at telomeres; including (Zaaijer et al., 2016) the shelterin protein TRF1 (Martínez et al., 2009; Sfeir et al., 2009), the DNA helicases RTEL1 (Vannier et al., 2012), BLM and WRN (Barefield and Karlseder, 2012), Topoisomerase TopoIIa (d'Alcontres et al., 2014) and Rif1 (Zaaijer et al., 2016). High levels of DNA damage and telomere fragility are characteristics of ALT cells (Cesare et al., 2009) (Min et al., 2017), presenting several DNA repair and damage factors in APBs (Draskovic et al., 2009; Wu et al., 2000; Yeager et al., 1999), indication of elevated telomeric stress in these cells. Therefore, it has been hypothesized that ALT mechanism arises from persistent replication stress, which can be resolved by the initial collapse of the replication fork, subsequently offering substrates for HR repair mechanisms dependent on homology search and telomere synthesis as reported with BIR pathway (Dilley et al., 2016).
In this study, we report that replication stress generated at TRF1 depleted telomeres in telomerase positive MEFs is associated with the recruitment of ALT signature factors including PML, subunits of the NuRD complex, BRCA1 and SMC5/6 complex. We suggest that the formation of permissive telomeric chromatin enables transcription of telomeric sequences into TERRAs and increases recombination as measured by T-SCEs, in a POLD3 and SMC5/POLD3 dependent manner, respectively. Moreover, we detect mitotic DNA synthesis at TRF1 depleted telomeres, which is dependent on POLD3 but not SMC5. Comparison analysis with APH-treated MEFs highlighted the unappreciated role of mouse TRF1 in suppressing telomere chromatin re-organisation, which we propose to contribute to HR-dependent telomere-sister chromatid exchanges. Herein, we showed that telomere replication stress induced by TRF1 deletion generates additional telomere responses (APBs, Chromatin remodeling, HR) compared to APH treated MEFs. We propose this is imputed to the function of TRF1 in stabilising telomere chromatin. Both mouse and human TRF1 suppresses telomere fragility and therefore is essential to facilitate DNA replication through telomeric repeats (Lee et al., 2018; Sfeir et al., 2009). We now show that like for mouse TRF1, its human homolog is essential to suppress APBs formation and BIR, that is POLD3 dependent in mouse and likely to contribute to the repair of stalled/collapsed telomeric forks.
Mouse TRF1 is essential for cell proliferation and to counteract TERRAs expression/stability and activation of HR, while it seems dispensable in HT1080-ST and HeLa cells. This could be explained by the difference in chromatin composition between the two species, indeed human telomeres display less abundant repressive marks compared to mouse chromosome ends (García-Cao et al., 2004; O'Sullivan et al., 2010; Rosenfeld et al., 2009). Moreover, mouse and human TERRAs have different origins. The main source of human TERRA molecules is from Human 20q subtelomere (Montero et al., 2016) however, other subtelomeres might contribute to local regulation and therefore control both cis and trans regulations (Tutton et al., 2016; Feretzaki et al., 2019). On the other hand, mouse TERRAs primary origin is more controversial with reports proposing Chr18 subtelomere as the principal source in MEFs (de Silanes et al., 2014), while recent literature hints to the subtelomeric regions of ChrX and ChrY (Chu et al., 2017). These PAR-TERRA would be more abundant and involved in trans regulation on the other chromosome ends.
Telomere transcription appears to be differently regulated in human and mouse cells and it is tempting to speculate that the difference in chromatin composition between the two species is essential for different transcription regulations. Therefore, it is plausible that human and mouse telomeres bear different accessibility to HR factors due to the distinct heterochromatin properties, that will undoubtedly be an interesting question to address in the future.
We suggest that chromatin remodelling factors such as NuRD-ZNF827 are recruited to mouse TRF1 deficient telomeres to counteract the shelterin instability. This may be explained by analogy with ALT telomeres where telomeric DNA sequence is interspersed with variant repeats (Conomos et al., 2012; Marzec et al., 2015), which are suggested to cause displacement of shelterin proteins (Conomos et al., 2013), thus increasing replication stress and DDR. In this scenario, nuclear receptors bind the interspersed variant repeats and recruit several chromatin remodelling factors including the NuRD complex, which can further alter the telomere architecture by increasing telomere compaction (Conomos et al., 2014); perhaps a transient state before stimulating telomere associations and generating more ‘open’ recombination permissive conditions at telomeres. In line with our findings, repressive chromatin at DSBs has been proposed to facilitate homology search and promote recruitment of HR proteins like BRCA1 (Khurana et al., 2014). In addition to BRCA1, we have identified through PICh analysis the SMC5/6 complex specifically recruited at TRF1 deficient telomeres. We demonstrate that this complex plays the same role at replication induced telomeres as in ALT cells, targeting telomeres to PML bodies (APBs) and facilitating telomeric HR at these sites (Potts et al., 2006), since double mutant SMC5-TRF1 disrupts formation of APBs and reduces T-SCEs events. However, we were unable to fully induce ALT in TRF1 deficient MEFs, as they display neither C-circles nor heterogeneity in telomere length and telomerase is still active. The latter could act as a stabiliser of telomeric DNA ends generated during fork restart (Tong et al., 2015), similarly to what happens in RTEL1-/- MEFs (Margalef et al., 2018) and in human RTEL1 deficient cells with long telomeres (Porreca et al., 2018).
Persistent DNA damage in ALT cells is suggested to originate from telomeric replication stress, which is proposed to be resolved by BIR in a POLD3 dependent manner (Dilley et al., 2016; Min et al., 2017; Roumelioti et al., 2016). Our results show that TRF1 is a major suppressor of telomeric replication stress and consequently of POLD3 dependent BIR. TRF1 deficient telomeres present slower movement of S-phase replication forks, measured by molecular combing (Sfeir et al., 2009). The slower replication rates at telomeres is proposed to be a consequence of the hindrance of the replication forks by DNA secondary structures, including formation of G-quadruplexes on the lagging strand template or RNA-DNA hybrids. In the absence of TRF1, BLM is unable to be recruited to replicated telomeres and to open DNA secondary structures (Lee et al., 2018; Zimmermann et al., 2014). Based on our results, we propose that in the absence of TRF1, POLD3 dependent BIR bypasses the stalled replication fork during G2/M phase.
Recent studies identified BIR as a mechanism to bypass RNA-DNA hybrids in a Rad52 and Pol32 dependent manner in yeast (Amon and Koshland, 2016; Neil et al., 2018). This mechanism is also conserved in human cells where POLD3 is necessary for the restart of stalled replication forks at RNA-DNA hybrids (Tumini et al., 2016). Altogether, we propose that increased TERRAs levels at TRF1 depleted telomeres could form RNA-DNA hybrids that are bypassed by POLD3 dependent BIR (Figure 8). This is in agreement with recent findings showing that TRF1 suppresses R-loop formation mediated by TRF2 (Lee et al., 2018). In contrast to Pold3, SMC5 acts as inhibitor of TERRA accumulation, as its absence is causing a significant increase in TERRA levels. This result is reminiscent of the role of yeast Smc5 in facilitating the resolution of toxic recombination intermediates at RNA-DNA hybrids generated by the helicase Mph1 (Chen et al., 2009; Lafuente-Barquero et al., 2017). We also describe a role of SMC5 in promoting T-SCEs, but not MiDAS formation (in contrast to PolD3), in the absence of TRF1. These results are indicating an exclusive function of SMC5 in HR at replicative-stressed telomeres, perhaps ensuring the right balance between accumulation and removal of HR-dependent intermediates formed during DNA repair (Aragón, 2018). On the other hand, the lack of Smc5 in promoting MiDAS seems in apparent contradiction with a recent observation in ALT cells (Min et al., 2017), where telomeric MiDAS is decreased in SMC5/6-depleted Saos2 cells. We speculate, this difference is due to an imbalance of factors used for ALT maintenance, compared to the early events observed in our conditional system after only few population doublings. Therefore, we cannot rule out a possible role of SMC5/6 in promoting MiDAS at a later stage, similar to the one observed in ALT maintenance.
Along with MUS81 structure specific nuclease, POLD3 and POLD4 subunits of the DNA polymerase delta are essential for CFS expression observed in human cells under replication stress (Minocherhomji et al., 2015; Tumini et al., 2016). To our surprise, TRF1-POLD3 double mutant did not show any suppression of telomere fragility, indicating key differences in the mechanism generating these phenotypes.
In conclusion, our analysis of TRF1 function provides an unappreciated molecular understanding of the level of protection that this shelterin protein offers at telomeres. Surprisingly, we establish that TRF1 ensures telomeric chromatin stability and suppresses the recruitment of chromatin remodellers including NuRD and the formation of APBs, which together promote a HR prone environment. At the same time, TRF1-dependent replication stress is associated with increased TERRAs expression/stability and activation of POLD3-dependent BIR to restart stalled/collapsed telomeric replication forks.
Materials and methods
Reagent type (species) or resource | Designation | Source or reference | Identifiers | Additional information |
---|---|---|---|---|
Cell line (Homo-sapiens) | HT1080-ST fibrosarcoma cell line | kind gift from J Lingner Cristofari and Lingner, 2006 | ||
Cell line (Homo-sapiens) | TRF1 CRISPR/Cas9 HeLa cell line | kind gift from Z Songyang Kim et al., 2017 | Doxycycline-inducible | |
Cell line (M. musculus) | TRF1F/F Mouse Embryonic Fibroblasts | Established in Boulton Lab (Crick Institute) Sfeir et al., 2009 | RRID:CVCL_UE12 Primary and SV40 immortalised | |
Transfected construct (human) - in HT1080-ST | TERF1 siRNA SMARTpool: ON-TARGETplus | Dharmacon/Thermo Fisher Scientific | #L-010542-02-0020 | 100 nM |
Transfected construct (human) - in HT1080-ST | ctl siRNA ON-TARGETplus Non-targeting Pool | Dharmacon | #D-001810-10-20 | 100 nM |
Other | Nucleofector II/2b Device | Lonza | #LO AAB-1001 | programme X-001. |
Commercial assay, kit | Nucleofector kit T | Lonza | #VVCA-1002 | |
Transfected construct (Mouse) | pLKO.1-puromycin lentiviral vectors shRNA for mouse SMC5 | Sigma | sequence: CCCATAATGCTCACGATTAAT | in MEFs |
Transfected construct (Mouse) | pLKO.1-puromycin lentiviral vectors shRNA for mouse POLD3 | Sigma | Sequence: GCATATACTCATGTGTGGTTT | in MEFs |
Transfected construct (Mouse) | pLKO.1-puromycin lentiviral vectors GAPDH | Open biosystems | Sequence: CTCATTTCCTGGTATGACA | in MEFs |
Software, algorithm | PRISM eight software, Statistical analysis | GraphPad | ||
Chemical compound, drug | DAPI stain | Invitrogen | D1306 | (1 µg/mL) |
Antibody | IgG, Rabbit polyclonal | Abcam | ab37415 | For ChIP 2 μg of antibody |
Antibody | TRF2, Rabbit polyclonal | Novus | NB110−57130/B2 | For ChIP 5 μg of antibody |
Antibody | BRCA1, Rabbit polyclonal | Novus | NBP1-45410 | For ChIP 5 μg of antibody |
Antibody | BAZ1b, Rabbit polyclonal | Cell Signaling | 2152S | For ChIP 5 μg of antibody |
Antibody | TR4, Mouse monoclonal | R and D biosystems | pp-H0107B-00 | For ChIP 5 μg of antibody |
Antibody | P66a, Rabbit polyclonal | Novus | NBP1-87359 | For ChIP 5 μg of antibody |
Antibody | MTA1, Rabbit polyclonal | Abcam | ab71153 | For ChIP 5 μg of antibody |
Antibody | CHD4, Rabbit polyclonal | Novus | NB100-57521 | For ChIP 5 μg of antibody |
Antibody | ZNF827, Mouse monoclonal | Santa Cruz | sc514943 | For ChIP 5 μg of antibody |
Antibody | IgG, Rabbit polyclonal | Abcam | ab37415 | For IF (1:1000) |
Antibody | TRF2, Rabbit polyclonal | Novus | NB110−57130/B2 | For WB (1:1000) and IF (1:250) |
Antibody | TRF2, Rabbit polyclonal | Gift from T de Lange | Ref: 1254 | For WB (1:1000) and IF (1:250) |
Antibody | TRF1, Rabbit polyclonal | Gift from T de Lange | Ref: 1449 | For WB (1:1000) and IF (1:250) |
Antibody | PML, Rabbit polyclonal | Gift from Paul Freemont | / | For IF (1:250) |
Antibody | SMC5, Rabbit polyclonal | Gift from Jo Murray | / | For WB (1:1000) and IF (1:250) |
Antibody | Beta-actin, Mouse monoclonal | Abcam | ab8226 | For WB (1:5000) |
Antibody | BrdU, Mouse monoclonal | MBL | MI-11–3 | For IF (1:500) |
Antibody | Anti-rabbit Alexa 488 antibody, Donkey polyclonal | Thermo, | A21206 | For IF (1:2000) |
Antibody | Anti-mouse Ig-HRP, Goat polyclonal | DAKO | P0447 | For WB (1:5000) |
Antibody | Anti-Rabbit Ig-HRP, Pig polyclonal | DAKO | P0217 | For WB (1:10000) |
For all quantification of telomeric phenotypes, masking was used during data analysis.
Cell culture, viral transductions and transfections with siRNAs
Request a detailed protocolTRF1 conditional knock-out MEFs (SV40-immortalised) were described previously (Martínez et al., 2009; Sfeir et al., 2009). Cells were cultured at 37°C in 5%CO2, using DMEM medium supplemented with 10% FCS (Sigma F2442). To achieve TRF1 deletion, cells were infected twice at 72 hr interval with Ad5-CMV-CRE (m.o.i. of 50) and harvested 3 or 4 days after the second infection.
pLKO.1-puromycin lentiviral vectors containing shRNAs for SMC5 (sequence CCCATAATGCTCACGATTAAT, Sigma), POLD3 (GCATATACTCATGTGTGGTTT, Dharmacon) or GAPDH (CTCATTTCCTGGTATGACA, Open biosystems) were introduced by infection of lentivirus-containing supernatant from 293FT cells. Puromycin selection was performed for 3 weeks at 2 µg/ml and several clones were expanded and cultured before screening them for knock-down efficiency.
HT1080-ST human fibrosarcoma cell line was previously described in Cristofari and Lingner (2006) and was a kind gift from J. Lingner. siRNAs transfections were performed in HT1080-ST cells using Cell Line Nucleofector kit T (Lonza #VVCA-1002) according to the manufacturer’s instructions. Briefly, 2 million HT1080-ST cells were transfected using 100 nM of SMARTpool: ON-TARGETplus TERF1 siRNA (Dharmacon #L-010542-02-0020) or ON-TARGETplus Non-targeting Pool (Dharmacon #D-001810-10-20). Transfection experiments were carried out using Nucleofector II/2b Device (Lonza #LO AAB-1001), programme X-001. Cells were harvested, counted and transfected again every 48 hr until final collection at day 6.
Doxycycline-inducible TRF1 CRISPR/Cas9 HeLa cell line was a kind gift from Z. Songyang and were previously described in Kim et al. (2017). Cells were incubated in 1 µg/ml of doxycycline for 15 days and the media containing fresh doxycycline was replaced every 2 days.
HeLa (Dox inducible TRF1 KO-CRISPR/Cas9) from Zhou Songyang (Kim et al., 2017); mycoplasma contamination testing status: negative. MEFs (TRF1F/F) established by Vannier at London Research Institute (now Crick Institute-Boulton Lab) and with cell services, mycoplasma contamination testing status: negative. HT1080 (originally Mao et al., 1993) is a kind gift from Joachim Lingner, mycoplasma contamination testing status: negative.
Aphidicolin treatment
Request a detailed protocolMEFs were treated with 0.4 µM of Aphidicolin (Sigma A0781) for 72 hr before collection and RNA dot blot analysis. For CO-FISH experiments, cells were treated with Aphidicolin for 56 hr before addition of fresh media containing BrdU/C and metaphase collection was performed after 16 hr. In all the experiments performed using Aphidicolin, media containing fresh drug was changed every 24 hr.
Western blot
Request a detailed protocolCells were scraped in cold PBS, spun down and incubated in lysis buffer (NaCl 40 mM; Tris 25 mM, pH 8; MgCl 2 mM; SDS 0.05%; Benzonase 1 µl/2 ml; Complete protease inhibitor cocktail, EDTA-free, Roche) for 10 min on ice. The lysates were sheared 10 times by forcing it through a 25G needle and left on ice for another 10 min. 35 μg of protein lysates were denatured for 10 min at 95°C after addition of Laemmli buffer 4X (50 mM Tris pH7; 100 mM DTT; 2%SDS; 0.1%bromophenol blue; 10% glycerol), separated on 4–12% Bis-Tris gels (Invitrogen) and transferred onto a nitrocellulose membrane (Amersham Protran 0.2 µm NC). Rabbit anti-TRF1 (gift from Titia de Lange) and rabbit anti-SMC5 (gift from Jo Murray) antibodies were diluted in PBST (PBS1x; 0.1% Tween-20, Sigma-Aldrich) with 5% non-fat milk. For detection of human TRF1, rabbit anti-TRF1 (SantaCruz #sc-6165-R) antibody was used at a final concentration of 0.2 µg/ml diluted in 5% non-fat milk (w/v) in TBS-T. Following incubations with HRP-coupled secondary antibodies signals were visualised using ECL II kit (Pierce) and x-ray film exposure (Amersham Hyperfilm ECL). Beta-actin antibody was used for normalisation (Abcam, ab8226).
Quantitative RT-PCR
Request a detailed protocolRNA extraction was carried out using RNeasy Mini Kit (Qiagen). 500 ng of RNA were subjected to reverse transcription using random hexamer primers and cDNA Synthesis Kit (Roche) according to the manufacturer’s protocol. Quantitative PCR was performed using QuantiTect SYBR Green PCR Master Mix and the following primers: mouse POLD3 with antisense 5’-ACACCAAGTAGGTAACATGCAG-3’ and sense 5’-AAGATCGTGACTTACAAGTGGC-3’ sequences; Mouse Actin with antisense 5’-CCAGTTGGTAACAATGCCATGT-3’ and sense 5’-GGCTGTATTCCCCTCCATCG-3’ sequences; The PCR cycles were as follows: 95°C for 15 min, 95°C for 15 s, 55°C for 30 s, 72°C for 30 s for 44 cycles.
Telomeric chromatin isolation by PICh
Request a detailed protocolPICh was carried out as previously described (Déjardin and Kingston, 2009) using the following 2’Fluoro-RNA probes for hybridisation: Destiobiotin-108 atom tether-UUAGGGUUAGGGUUAGGGUUAGGGt (Telo probe); Destiobiotin-108 atom tether-GAUGUGGAUGUGGAUGUGGAUGUGg (Scramble probe).
Gel and post digestion processing
Request a detailed protocolGels were processed using a variant of the in-gel digestion procedure as described in Shevchenko et al. (2006). Briefly, gel sections were excised and chopped into uniform cubes, followed by de-staining with 50/50, 50 mM ammonium bicarbonate (AmBic)/acetonitrile(ACN). Gel sections were then dehydrated with 100% ACN followed by the subsequent sequential steps: reduction with 10 mM dithiothreitol (DTT) at 56°C for 30 min in the dark, dehydration, alkylation with 55 mM iodoacetamide (IAM) at RT for 20 min in the dark and dehydration. Gel sections were finally re-hydrated with a 40 mM AmBic, 10% ACN solution containing 500 ng of Trypsin Gold (Promega, V5280) and incubated overnight at 37°C.Recovered gel digest extracts were dried on a speed-vac, reconstituted with 99/1, H2O/ACN + 0.1% FA and de-salted using a standard stage tip procedure using C18 spin tips (Glygen Corp, TT2C18). Dried gel digest peptide extracts solubilised in 25 µl of 0.1% trifluoroacetic acid (TFA) and clarified solution transferred to auto sampler vials for LC-MS analysis.
Mass spectrometry analysis
Request a detailed protocolPeptides were separated using an Ultimate 3000 RSLC nano liquid chromatography system (Thermo Scientific) coupled to a LTQ Velos Orbitrap mass spectrometer (Thermo Scientific) via an EASY-Spray source. 6 μL of sample was loaded in technical duplicates onto a trap column (Acclaim PepMap 100 C18, 100 μm × 2 cm) at 8 μL/min in 2% acetonitrile, 0.1% TFA. Peptides were then eluted on-line to an analytical column (EASY-Spray PepMap C18, 75 μm × 25 cm). Peptides were separated using a linear 120 min gradient, 4–45% of buffer B (composition of buffer B– 80% acetonitrile, 0.1% formic acid). Eluted peptides were analysed by the LTQ Velos operating in positive polarity using a data-dependent acquisition mode. Ions for fragmentation were determined from an initial MS1 survey scan at 15000 resolution (at m/z 200), followed by Ion Trap CID (collisional induced dissociation) of the top 10 most abundant ions. MS1 and MS2 scan AGC targets set to 1e6 and 1e4 for a maximum injection time of 500 ms and 100 ms respectively. A survey scan m/z range of 350–1500 was used, with a normalised collision energy set to 35%, charge state rejection enabled for +one ions and a minimum threshold for triggering fragmentation of 500 counts.
Data analysis
Request a detailed protocolAll data files acquired were loaded into MaxQuant (Cox et al., 2014) version 1.6.0.13 analysis software. Raw files were combined into an appropriate experimental design to reflect technical and biological replicates. The LFQ algorithm and match between runs settings were selected. Data were searched against the UniProt Reference Proteome Mus musculus protein database (UP000000589), downloaded on 16th January 2019 from the UniProt website. The database contains 17,002 reviewed (Swiss-Prot) and 37,186 un-reviewed (TrEMBL) protein sequences. MaxQuant also searched the same database with reversed sequences so as to enable a 1% false discovery rate at peptide and protein levels. A built-in database of common protein contaminants was also searched.
Upon completion of the search, the ‘proteingroups.txt’ output file was loaded in Perseus version 1.4.0.2. Contaminant and reverse protein hits were removed. LFQ intensities were log2 transformed. Data were group categorised to ‘Scramble’, ‘Telomere’ or ‘Deletion’. Data were filtered for a minimum of 3 valid LFQ intensity values in at least one group. Missing values (NaN) were imputed from a normal distribution with default values.
FISH and CO-FISH on metaphase spreads
Request a detailed protocolFor metaphase spread preparation, cells were incubated for 60 min with 10 ng/ml colcemid (Roche). Cells were harvested, swollen in 75 mM KCl solution for 15 min at 37°C, fixed in ethanol/acetic acid solution (3:1, v/v) and washed three times with the same fixing solution. Suspensions of fixed cells were dropped onto glass slides and dried overnight before performing FISH experiments.
Q-FISH and CO-FISH procedures were performed as previously described (Ourliac-Garnier and Londoño-Vallejo, 2011). Briefly, metaphase spreads were fixed in 4% formaldehyde for 2 min, washed 3 × 5 min in PBS 1x, treated with pepsin (1 mg/ml in 0.05 M citric acid pH 2) for 10 min at 37°C, post-fixed for 2 min, washed and incubated with ethanol series (70%, 80%, 90%, 100%). Hybridising solution containing Cy3-O-O-(CCCTAA)3 probe (PNA bio) in 70% formamide, 10 mM Tris pH 7.4% and 1% blocking reagent (Roche, 11096176001) was applied to each slide, followed by denaturation for 3 min at 80°C on heating block. After 2 hr hybridisation at RT, slides were washed twice 15 min in 70% formamide, 20 mM Tris pH 7.4, followed by three washes of 5 min in 50 mM Tris pH 7.4, 150 mM NaCl, 0.05% Tween-20, dehydrated in successive ethanol baths and air-dried. Slides were mounted in antifade reagent (ProLong Gold, Invitrogen) containing DAPI and images were captured with Zeiss microscope using Carl Zeiss software. Telomeric signals were quantified using the ImageJ software (Fiji).
For CO-FISH, the cells were treated with 10 µM BrdU:BrdC (3:1) for 16 hr, followed by colcemid treatment as above. Prior to hybridisation slides were treated with RNAse A (0.5 µg/ml in PBS) for 10 min at 37°C, incubated with Hoechst (1 µg/ml in 2XSSC) for 10 min at RT, exposed to UV light for 1 hr and treated with ExoIII to degrade the neosynthesised DNA strand containing BrdU/C. Slides were next dehydrated through ethanol series, hybridising solution containing TelG-FAM probe (Exiqon) in 50% formamide, 2XSSC, 1% blocking reagent was applied to each slide, followed by denaturation for 3 min at 80°C on heating block and hybridisation for 2 hr in the dark. Slides were washed 2 × 15 min in 50% formamide, 2XSSC and 3 × 5 min in 50 mM Tris pH 7.4, 150 mM NaCl, 0.05% Tween-20. Finally, slides were dehydrated, incubated with TelC-cy3 probe for 2 hr, followed by the steps described above in the FISH protocol.
Immunofluorescence-FISH
Request a detailed protocolCells seeded on slides were permeabilised with Triton X-100 buffer (0.5% Triton X-100; 20 mM Tris pH8; 50 mM NaCl; 3 mM MgCl2; 300 mM sucrose) at RT for 5 min and then fixed in 3% formaldehyde/2%sucrose in PBS1X for 15 min at RT and washed three times in PBS1X. After a 10 min permeabilisation step and a wash in PBS1X, nuclei were incubated with blocking solution (10% serum in PBS1X) for 30 min at 37°C and stained with specific primary antibodies: rabbit anti-PML (1/200, a gift from Paul Freemont); rabbit anti-53bp1 dilution (1/400, Bethyl A300-272A). After three washes in PBS1X, nuclei were incubated with secondary donkey anti-rabbit Alexa 488 antibody (1/400, Life Technologies) for 40 min at 37°C, washed three times in PBS1X, post fixed 10 min and hybridised with TelC-cy3 PNA probe as described in FISH protocol.
EdU labeling and staining were performed as previously reported (Minocherhomji et al., 2015). Briefly, cells were incubated 1 hr with EdU (100 µM) and colcemid (10 ng/ml), followed by metaphase spread preparation. For EdU staining, the steps of fixation, pepsin treatment and dehydration in ethanol serial dilutions were carried out as in FISH protocol, followed by Click IT assay using EdU-Alexa Fluor 488 imaging kit according to the manufacturer’s instructions (Thermo Fisher). Metaphases were post-fixed and hybridised with TelC-cy3 PNA probe.
Terra-fish
Request a detailed protocolTERRA-FISH experiment was carried out as previously described (Azzalin et al., 2007) with minor modifications. Briefly cells were permeabilised 5 min with cold CSK buffer (10 mM Pipes pH7;100 mM NaCl; 300 mM sucrose; 3 mM MgCl2; 0.5% Triton X-100 and 10 mM of inhibitor Ribonucleoside Vanadyl Complex). After a wash in PBS1X, cells were fixed for 10 min in 3%formaldeyde solution and washed three times with PBS, followed by Immunofluorescence with primary anti-TRF2 (dilution 1/10.000, 1254 ab gift from T. de Lange). Nuclei were then incubated with secondary donkey anti-rabbit Alexa 488 antibody (1/400, Life Technologies) for 40 min at 37°C, washed three times in PBS1X and post fixed for 10 min. After incubation with ethanol series (70%, 80%, 90%, 100%) slides were dried O/N in the dark. TelC-cy3 PNA probe was used for TERRA detection and after incubation for 2 hr at RT, slides were washed 3 × 5 min in 50% formamide, 2XSSC at 39°C, 3 × 5 min in 2XSSC at 39°C and a final wash in 2XSSC at RT. Slides were dehydrated in successive ethanol baths, air-dried and mounted in antifade reagent (ProLong Gold, Invitrogen) containing DAPI and images were captured with Zeiss microscope using Carl Zeiss software. Quantification was performed using CellProfiler 3.1.8 software.
Chromatin immunoprecipitation (ChIP)
Request a detailed protocolChromatin preparation and ChIP experiments were performed as previously described (Porreca et al., 2018) with the following modifications: sonication of chromatin was performed for 20 min (30 s on/30 s off) in a Diagenode water bath-sonicator at high speed. 20–50 μg of chromatin was diluted 10 times in ChIP dilution buffer (20 mM Tris-HCl pH 8, 150 mM KCl, 2 mM EDTA pH 8, 1% Triton X-100, 0.1% SDS), pre-cleared with Dynabeads (Invitrogen) and incubated overnight with 2–5 μg of antibody (listed in the key resources table).
RNA dot blot
Request a detailed protocolRNA extraction was carried out using RNeasy Mini Kit (Qiagen), according to the manufacturer instructions. 2 µg of RNA were denatured in 0.2 M NaOH by heating at 65°C for 10 min, incubated 5 min on ice and spotted onto a positively charged Biodyne B nylon membrane (Amersham Hybond, GE Healthcare). Membranes were UV-crosslinked (Stratalinker, 2000 kJ) and baked for 45 min at 80°C, followed by hybridisation at 42°C with digoxigenin (DIG)-labeled telomeric C-rich oligonucleotide TAA(CCCTAA)4, prepared using 3’ end labelled kit (Roche). Signal was revealed using the anti-DIG-alkaline phosphatase antibodies (Roche) and CDP-Star (Roche) following the manufacturer’s instructions. Images were captured using the Amersham Imager 680 (GE Healthcare) and analysed using the Image Studio Lite software.
18 s rRNA probe with sequence: 5’-CCATCCAATCGGTAGTAGCG was used for normalisation.
Northern blot
Request a detailed protocol10 µg of RNA was denatured for 10 min at 65°C in sample buffer (50% formamide, 2.2M formaldehyde, 1X MOPS) followed by ice incubation for 5 min. 10X Dye buffer (50% Glycerol, 0.3% Bromophenol Blue, 4 mg/ml Ethidium Bromide) was added to each sample and all of them were run on a formaldehyde agarose gel (0.8% agarose, 1X MOPS, 6.5% formaldehyde) at 5V per cm in 1X MOPS buffer (0.2M MOPS, 50 mM NaOAc, 10 mM EDTA, RNAse free water). The gel was rinsed twice in water, washed twice with denaturation solution (1.5M NaCL, 0.05M NaOH), followed by additional three washes with 20XSSC before transferring the RNA on a positively charged Biodyne B nylon membrane (Amersham Hybond, GE Healthcare) using a neutral transfer in 20XSSC. The membrane was fixed and detected as described for the RNA dot blot.
Data availability
All data generated or analysed during this study are included in the manuscript and supporting files. Source data files have been provided for all figures. Proteomic data have been made available at ProteomeXchange, under the accession code PXD017022.
-
ProteomeXchangeID PXD017022. Data from: TRF1 averts chromatin remodelling, recombination and replication dependent-Break Induced Replication at mouse telomeres.
References
-
The Smc5/6 complex: new and old functions of the enigmatic Long-Distance relativeAnnual Review of Genetics 52:89–107.https://doi.org/10.1146/annurev-genet-120417-031353
-
Spontaneous occurrence of telomeric DNA damage response in the absence of chromosome fusionsNature Structural & Molecular Biology 16:1244–1251.https://doi.org/10.1038/nsmb.1725
-
Alternative lengthening of telomeres: models, mechanisms and implicationsNature Reviews Genetics 11:319–330.https://doi.org/10.1038/nrg2763
-
PAR-TERRA directs homologous sex chromosome pairingNature Structural & Molecular Biology 24:620–631.https://doi.org/10.1038/nsmb.3432
-
Variant repeats are interspersed throughout the telomeres and recruit nuclear receptors in ALT cellsThe Journal of Cell Biology 199:893–906.https://doi.org/10.1083/jcb.201207189
-
NuRD-ZNF827 recruitment to telomeres creates a molecular scaffold for homologous recombinationNature Structural & Molecular Biology 21:760–770.https://doi.org/10.1038/nsmb.2877
-
Accurate proteome-wide label-free quantification by delayed normalization and maximal peptide ratio extraction, termed MaxLFQMolecular & Cellular Proteomics 13:2513–2526.https://doi.org/10.1074/mcp.M113.031591
-
SMARCAL1 resolves replication stress at ALT telomeresCell Reports 14:1032–1040.https://doi.org/10.1016/j.celrep.2016.01.011
-
Shelterin: the protein complex that shapes and safeguards human telomeresGenes & Development 19:2100–2110.https://doi.org/10.1101/gad.1346005
-
Induction of sister chromatid exchanges at common fragile sitesAmerican Journal of Human Genetics 41:882–890.
-
Characterization of RAD51-independent break-induced replication that acts preferentially with short homologous sequencesMolecular and Cellular Biology 22:6384–6392.https://doi.org/10.1128/MCB.22.18.6384-6392.2002
-
Targeted deletion reveals an essential function for the telomere length regulator Trf1Molecular and Cellular Biology 23:6533–6541.https://doi.org/10.1128/MCB.23.18.6533-6541.2003
-
TRF1 participates in chromosome end protection by averting TRF2-dependent telomeric R loopsNature Structural & Molecular Biology 25:147–153.https://doi.org/10.1038/s41594-017-0021-5
-
ATR suppresses telomere fragility and recombination but is dispensable for elongation of short telomeres by telomeraseThe Journal of Cell Biology 188:639–652.https://doi.org/10.1083/jcb.200908136
-
Alternative lengthening of telomeres mediated by mitotic DNA synthesis engages Break-Induced replication processesMolecular and Cellular Biology 37:e00226.https://doi.org/10.1128/MCB.00226-17
-
Structure-specific endonucleases: guardians of fragile site stabilityTrends in Cell Biology 24:321–327.https://doi.org/10.1016/j.tcb.2013.11.007
-
Telomeric RNAs are essential to maintain telomeresNature Communications 7:12534.https://doi.org/10.1038/ncomms12534
-
Structural organizations of replicon domains during DNA synthetic phase in the mammalian nucleusExperimental Cell Research 165:291–297.https://doi.org/10.1016/0014-4827(86)90583-5
-
RNA-DNA hybrids promote the expansion of friedreich's ataxia (GAA)n repeats via break-induced replicationNucleic Acids Research 46:3487–3497.https://doi.org/10.1093/nar/gky099
-
Reduced histone biosynthesis and chromatin changes arising from a damage signal at telomeresNature Structural & Molecular Biology 17:1218–1225.https://doi.org/10.1038/nsmb.1897
-
Telomere length analysis by quantitative fluorescent in situ hybridization (Q-FISH)Methods in Molecular Biology 735:21–31.https://doi.org/10.1007/978-1-61779-092-8_3
-
How shelterin protects mammalian telomeresAnnual Review of Genetics 42:301–334.https://doi.org/10.1146/annurev.genet.41.110306.130350
-
Human RTEL1 stabilizes long G-overhangs allowing telomerase-dependent over-extensionNucleic Acids Research 46:4533–4545.https://doi.org/10.1093/nar/gky173
-
The SMC5/6 complex maintains telomere length in ALT Cancer cells through SUMOylation of telomere-binding proteinsNature Structural & Molecular Biology 14:581–590.https://doi.org/10.1038/nsmb1259
-
Roles of human POLD1 and POLD3 in genome stabilityScientific Reports 6:38873.https://doi.org/10.1038/srep38873
-
Telomerase-negative immortalized human cells contain a novel type of promyelocytic leukemia (PML) bodyCancer Research 59:4175–4179.
Article and author information
Author details
Funding
European Commission (637798 MetDNASecStr)
- Rosa Maria Porreca
- Emilia Herrera-Moyano
- Eleni Skourti
- Pui Pik Law
- Jean-Baptiste Vannier
Medical Research Council (Telomere Replication and Stability)
- Roser Gonzalez Franco
- Alex Montoya
- Peter Faull
- Holger Kramer
- Jean-Baptiste Vannier
The funders had no role in study design, data collection and interpretation, or the decision to submit the work for publication.
Acknowledgements
We thank Titia de Lange for providing TRF1 antibody, Paul Freemont for providing PML antibody, Jo Murray for SMC5 antibody, Joachim Lingner for HT1080 cells and Zhou Songyang for TRF1 inducible CRISPR/Cas9 cells. Special thanks to Paulina Marzec for teaching RMP the PICh techniques in Boulton Lab and Jerome Dejardin for the constructive feedback. We thank Arturo Londono, Julia P Cooper, Julian Sale, Titia de Lange, Valerie Borel, Simon Boulton for their helpful comments. TRF1 MEFs were generated in Simon Boulton’s group from Titia de Lange’s mouse line.
Vannier lab’s work is supported by the London Institute of Medical Sciences (LMS), which receives its core funding from UKRI (MRC) and by an ERC Starter Grant (637798; MetDNASecStr). Rosa Maria Porreca is funded by ERC Starter Grant (637798; MetDNASecStr).
Copyright
© 2020, Porreca et al.
This article is distributed under the terms of the Creative Commons Attribution License, which permits unrestricted use and redistribution provided that the original author and source are credited.
Metrics
-
- 3,576
- views
-
- 582
- downloads
-
- 30
- citations
Views, downloads and citations are aggregated across all versions of this paper published by eLife.
Citations by DOI
-
- 30
- citations for umbrella DOI https://doi.org/10.7554/eLife.49817