Metabolic modulation regulates cardiac wall morphogenesis in zebrafish
Abstract
During cardiac development, cardiomyocytes form complex inner wall structures called trabeculae. Despite significant investigation into this process, the potential role of metabolism has not been addressed. Using single cell resolution imaging in zebrafish, we find that cardiomyocytes seeding the trabecular layer actively change their shape while compact layer cardiomyocytes remain static. We show that Erbb2 signaling, which is required for trabeculation, activates glycolysis to support changes in cardiomyocyte shape and behavior. Pharmacological inhibition of glycolysis impairs cardiac trabeculation, and cardiomyocyte-specific loss- and gain-of-function manipulations of glycolysis decrease and increase trabeculation, respectively. In addition, loss of the glycolytic enzyme pyruvate kinase M2 impairs trabeculation. Experiments with rat neonatal cardiomyocytes in culture further support these observations. Our findings reveal new roles for glycolysis in regulating cardiomyocyte behavior during cardiac wall morphogenesis.
Introduction
During development, the heart undergoes a series of morphogenetic changes to form a functional cardiac wall structure (Moorman and Christoffels, 2003; Staudt and Stainier, 2012). The outer wall of the developing ventricle consists of compact layer cardiomyocytes (CMs), while the inner wall consists of complex muscular ridges, termed trabeculae, which facilitate efficient cardiac contraction and oxygenation of the cardiac wall prior to the formation of coronary vessels (Sedmera et al., 2000; Staudt and Stainier, 2012). Disruption of ventricular wall morphogenesis is associated with congenital cardiac malformations, the most common type of birth defects (Fahed et al., 2013), yet the cellular and molecular mechanisms regulating this complex process remain unclear.
Neuregulin (NRG)/Erb-b2 receptor tyrosine kinase (ERBB) 2/4 signaling has been shown to be essential for cardiac trabeculation. Nrg1, Erbb2 and Erbb4 knockout mice (Gassmann et al., 1995; Lee et al., 1995; Meyer and Birchmeier, 1995) and nrg2a (Rasouli and Stainier, 2017) and erbb2 (Liu et al., 2010) mutant fish fail to form trabeculae. ERBBs are members of the epidermal growth factor (EGF) receptor tyrosine kinase family. NRGs are expressed by the endocardium (Corfas et al., 1995; Meyer and Birchmeier, 1995; Grego-Bessa et al., 2007; Rasouli and Stainier, 2017) and bind to ERBBs on CMs, triggering homo- or heterodimerization of ERBB family members and leading to activation of downstream pathways (Sanchez-Soria and Camenisch, 2010). However, the targets of ERBB2 signaling that regulate CM behavior during trabeculation have not been identified.
Cardiac metabolism has been extensively studied in adult animals due to its central role in supplying energy for cardiac contraction (Doenst et al., 2013; Kolwicz et al., 2013). Adult CMs rely mostly on fatty acids as an energy substrate, and they are oxidized in mitochondria to generate ATP (Ellen Kreipke et al., 2016). Under conditions of hypertrophic or ischemic stress, CMs revert to glycolytic metabolism (Doenst et al., 2013), which is characteristic of embryonic cardiomyocytes and uses glucose as a fuel. Besides its role in energy generation, little is known about the role of metabolism during cardiac development.
Here, using high-resolution single cell imaging in zebrafish, we first show that developing CMs undergo extensive shape changes during the formation of the trabecular layer. By modulating glucose metabolism pharmacologically, we show that glycolysis regulates these processes. Using CM-specific loss- and gain-of-function models as well as mutant animals compromised in their glycolytic activity, we identify a role for glycolysis in cardiac wall morphogenesis. This study provides new insights into the role of cardiac metabolism in cardiac development.
Results
Cardiomyocytes that enter the trabecular layer exhibit distinct behaviors
During cardiac trabeculation in zebrafish and mouse, CMs delaminate from the compact layer to seed the trabecular layer (Liu et al., 2010; Zhang et al., 2013; Staudt et al., 2014; Jiménez-Amilburu et al., 2016; Del Monte-Nieto et al., 2018). Although CM behavior during trabeculation has been observed in zebrafish (Staudt et al., 2014; Cherian et al., 2016), the 3D morphology of single cardiomyocytes during the trabeculation process needs to be further explored. To this end, we performed 3D time-course imaging using chimeric hearts generated by cell transplantation. To label CM membranes and nuclei with EGFP and DsRed2 respectively, we used Tg(myl7:EGFP-HRAS); Tg(myl7:nDsRed2) cells as donors (Figure 1a and Figure 1—figure supplement 1a). We found that delaminating CMs exhibit morphological changes as well as rearrangements of contact sites (Figure 1b–c” and Figure 1—figure supplement 1b–d”; Figure 1—videos 1 and 2), while CMs remaining in the compact layer do not exhibit such changes (Figure 1d–e”). To examine cell-cell junctions during delamination, we analyzed N-cadherin (Cdh2), a major adherens junction component, at single cell resolution, and to this end used Tg(myl7:EGFP-HRAS); Tg(myl7:cdh2-tdTomato) cells as donors (Figure 1—figure supplement 1e). We observed that N-cadherin localizes to protruding membranes in delaminating CMs (Figure 1—figure supplement 1f–g”) and to the lateral membranes of compact layer CMs (Figure 1—figure supplement 1h–i”), in agreement with a previous report (Cherian et al., 2016). Next, we analyzed sarcomere structure during delamination using Tg(myl7:LIFEACT-GFP); Tg(myl7:nDsRed2) cells as donors. We found that CMs display partial sarcomere disassembly in their protrusions when entering the trabecular layer (Figure 1f–g”). These data indicate that delaminating CMs exhibit distinct behaviors including dynamic cell shape changes.
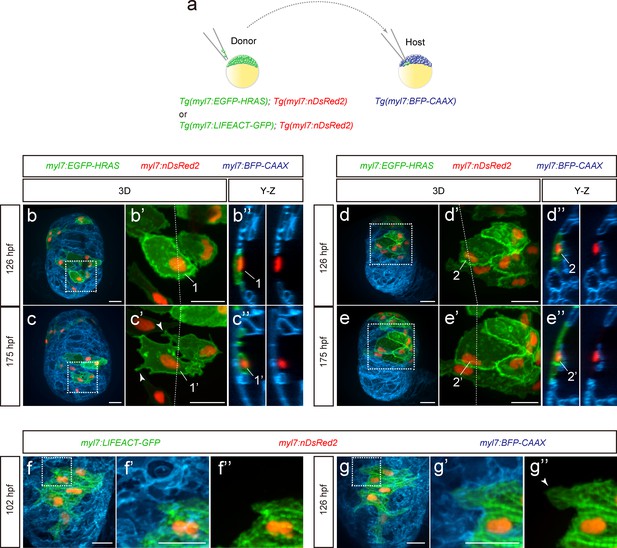
Cardiomyocyte behavior during cardiac trabeculation.
(a) Schematic of the transplantation experiment. (b–e) 3D time-course images of chimeric hearts; magnified view (b’, c’, d’, e’) of area in white boxes and Y-Z plane images (b”, c”, d”, e”) along white dashed lines (b’, c’, d’, e’). CMs initially in the compact layer (b’, b”) enter the trabecular layer (c’, c”) exhibiting morphological changes and membrane protrusions (c’; arrowheads; n = 5 CMs); CMs remaining in the compact layer (d’, d”, e’, e”) do not exhibit obvious morphological changes (n = 5 CMs). The same CMs are shown at 126 and 175 hpf as indicated in the images. (f, g) 3D time-course images of chimeric heart; magnified view (f’, f”, g’, g”) of area in white boxes. CMs entering the trabecular layer exhibit partial disassembly of their sarcomeres (g’; arrowhead). Scale bars, 20 μm.
ERBB2 signaling activates glycolysis in cardiomyocytes
To gain additional insight into the molecular mechanisms that regulate trabeculation, we focused on ERBB2 signaling which is essential for this process in both mouse (Gassmann et al., 1995; Lee et al., 1995; Meyer and Birchmeier, 1995) and zebrafish (Liu et al., 2010; Peshkovsky et al., 2011; Rasouli and Stainier, 2017). In order to identify targets of ERBB2 signaling, we analyzed protein expression in a CM-specific transgenic mouse model inducibly expressing a constitutively active form of ERBB2 (CAERBB2) (D’Uva et al., 2015). We found that upon CAERBB2 overexpression (OE), several glycolytic enzymes were upregulated, while mitochondrial proteins and oxidative phosphorylation (OXPHOS)-related enzymes were downregulated (Figure 2—source data 1). We also tested the effects of Erbb2 OE, which like CAErbb2 OE, activates downstream signaling (Pedersen et al., 2009), on the expression of glycolytic enzyme genes in rat neonatal CMs, and found that many of them were upregulated (Figure 2a). Notably, we found that Erbb2 OE in rat neonatal CMs greatly upregulated the levels of pyruvate kinase M2 (PKM2), a key glycolytic enzyme, (Figure 2b and c), and increased glycolytic activity as evidenced by measuring extracellular acidification rate (ECAR) (Figure 2d). NRG1 stimulation also activated glycolysis in rat neonatal CMs (Figure 2—figure supplement 1a). These findings are also supported by a study analyzing changes in mRNA levels in caErbb2 OE mouse hearts (Honkoop et al., 2019). Moreover, we treated zebrafish embryos with an Erbb2 inhibitor (Figure 2e), which has been shown to severely affect trabeculation (Figure 2—figure supplement 1b) (Liu et al., 2010; Peshkovsky et al., 2011), and found that the cardiac expression of glycolytic enzyme genes was downregulated (Figure 2b). Altogether, these data indicate that ERBB2 signaling activates glycolysis in CMs.
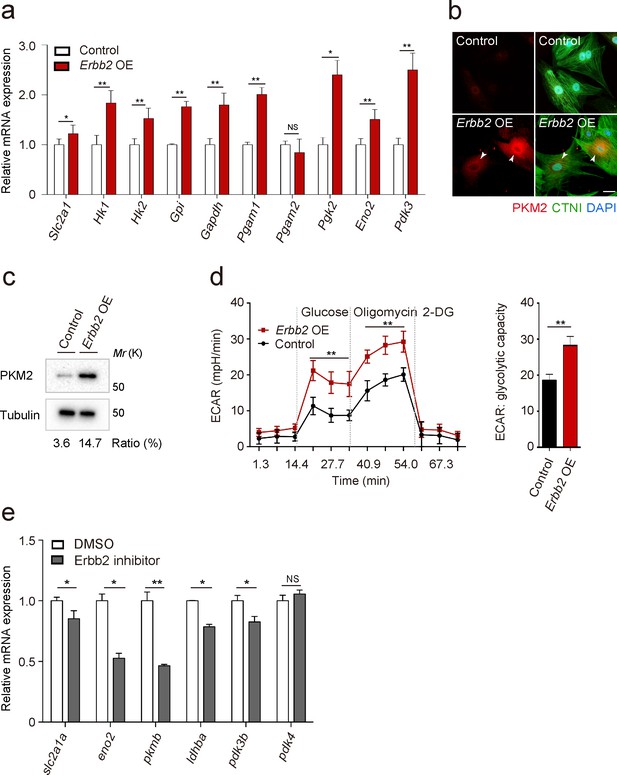
ERBB2 signaling activates glycolysis in cardiomyocytes.
(a) qPCR analysis of mRNA levels of glycolytic enzyme genes in control and Erbb2 overexpressing (OE) rat neonatal CMs (n = 3). Error bars, s.e.m. (b) Staining for PKM2, CTNI and DNA (DAPI) in control and Erbb2 OE rat neonatal CMs; arrowheads point to PKM2+ CMs. (c) Western blot analysis of PKM2 levels in control and Erbb2 OE rat neonatal CMs. (d) Extracellular acidification rate (ECAR) analysis in control and Erbb2 OE rat neonatal CMs; glycolytic capacity shown on the right (n = 7). Error bars, s.d. (e) qPCR analysis of mRNA levels of glycolytic enzyme genes in DMSO and Erbb2 inhibitor treated zebrafish hearts (n = 3). Error bars, s.e.m.; *p<0.05 and **p<0.001 by two-tailed unpaired t-test. NS, not significant. Scale bar, 20 μm.
-
Figure 2—source data 1
Mass spectrometry data.
P7 WT and CAERBB2 OE mouse hearts were isolated and protein expression levels analyzed by mass spectrometry. All presented proteins are statistically significant at p<0.05.
- https://cdn.elifesciences.org/articles/50161/elife-50161-fig2-data1-v2.docx
-
Figure 2—source data 2
Primer sequences for qPCR analysis.
Primer sequences used in Figure 2a and e.
- https://cdn.elifesciences.org/articles/50161/elife-50161-fig2-data2-v2.docx
-
Figure 2—source data 3
Mean Ct values of qPCR analysis in Figure 2a and e.
- https://cdn.elifesciences.org/articles/50161/elife-50161-fig2-data3-v2.docx
Glycolysis regulates cardiomyocyte delamination during development
In order to analyze the role of glycolysis during trabeculation, we first focused on pyruvate metabolism. The pyruvate dehydrogenase complex (PDC) catalyzes the conversion of pyruvate to acetyl-coenzyme A (acetyl-CoA), which enters the tricarboxylic acid cycle (Zhang et al., 2014). Pyruvate dehydrogenase kinases (PDKs) inhibit PDC activity and enhance glycolysis in CMs (Zhao et al., 2008) and cancer cells (Koukourakis et al., 2005; Lu et al., 2008; Leclerc et al., 2017; Peng et al., 2018), thereby regulating the switch between glycolysis and OXPHOS (Zhang et al., 2014). Our analyses show that ERBB2 signaling positively regulates Pdk3 gene as well as protein expression (Figure 2a and b and Figure 2—source data 1). Thus, we hypothesized that PDK3 was one of the key enzymes regulating glycolysis in delaminating CMs in response to Erbb2 signaling. Consistent with this model, we found that the PDK inhibitor dichloroacetate (DCA) led to a significant reduction in the number of CMs in the trabecular layer (Figure 3a). Of note, this phenotype is similar to the one caused by Erbb2 inhibition (Figure 3a).
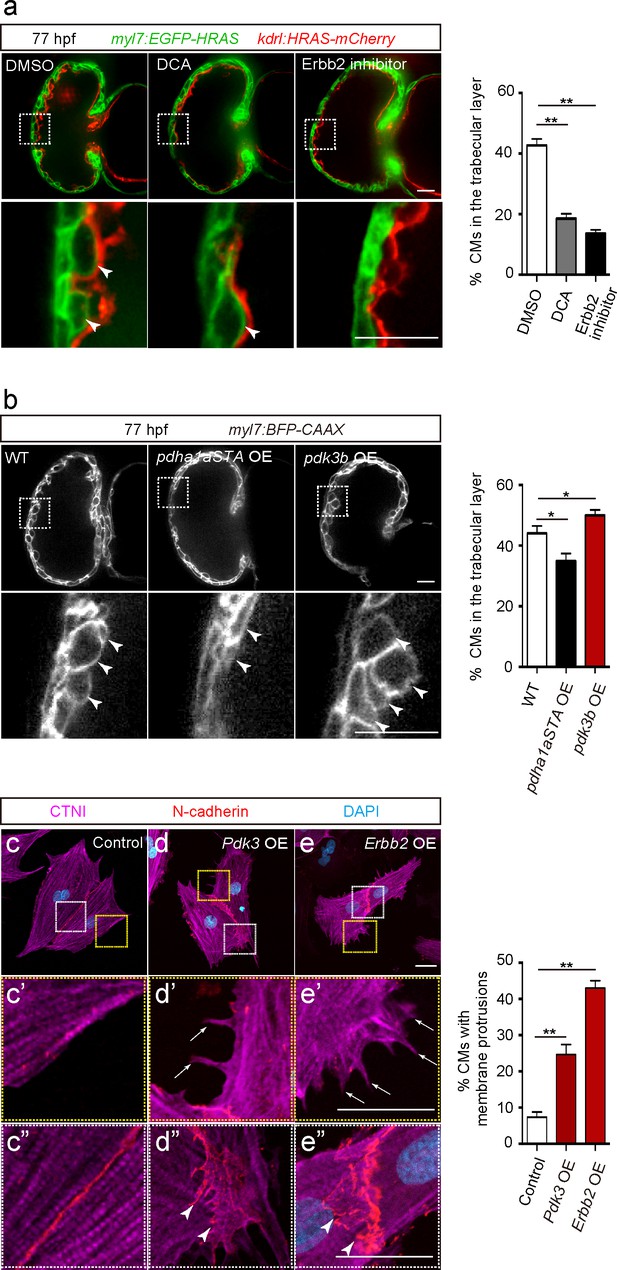
Glycolysis regulates cardiac trabeculation.
(a) Confocal images (mid-sagittal sections) of 77 hpf hearts treated with DMSO, dichloroacetate (DCA) or Erbb2 inhibitor; magnified view of area in white boxes shown below; arrowheads point to CMs in the trabecular layer; percentage of CMs in the trabecular layer shown on the right (n = 5–7 ventricles). (b) Confocal images (mid-sagittal sections) of 77 hpf Tg(myl7:BFP-CAAX) alone or in combination with Tg(myl7:pdha1aSTA-P2A-tdTomato) or Tg(myl7:pdk3b-P2A-tdTomato) hearts; magnified view of area in white boxes shown below; arrowheads point to CMs in the trabecular layer; percentage of CMs in the trabecular layer shown on the right (n = 5–7 ventricles). (c–e”) Staining for CTNI, N-cadherin and DNA (DAPI) in control (c), Pdk3 (d) and Erbb2 (e) OE rat neonatal CMs; magnified view of area in yellow (c’, d’, e’) and white (c”, d”, e”) boxes; percentage of CMs exhibiting membrane protrusions shown on the right (n = 3 individual experiments; each value corresponds to an average of 30 CMs). Pdk3 and Erbb2 OE causes rat neonatal CMs to exhibit membrane protrusions (d’, e’; arrows) and cell-cell junction rearrangements (d’, e’; arrowheads). Error bars, s.e.m.; *p<0.05 and **p<0.001 by ANOVA followed by Tukey’s HSD test. Scale bars, 20 μm.
We next focused on pyruvate dehydrogenase E1 alpha 1 subunit a (pdha1a), which encodes a catalytic subunit of the PDC. Analysis of CM-specific loss of Pdha1 in mice has revealed the importance of PDC activity for OXPHOS (Sun et al., 2016). We generated a Tg(myl7:pdha1aSTA-P2A-tdTomato) line to overexpress an activated form of Pdha1a in CMs. This activated form of Pdha1a (Pdha1aSTA) contains mutations in its phosphorylation sites and thus is not inhibited by PDK. As a result, glycolysis is reduced and OXPHOS enhanced, as previously shown in cancer cells (Hitosugi et al., 2011; Fan et al., 2014). Notably, larvae expressing this activated form of Pdha1a exhibited a significant decrease in the number of CMs in the trabecular layer (Figure 3b and Figure 3—figure supplement 1a). We also generated a Tg(myl7:pdk3b-P2A-tdTomato) line to overexpress pdk3b in CMs and thereby promotes glycolysis (Lu et al., 2008), and found that these transgenic larvae exhibited a significant increase in the number of CMs in the trabecular layer (Figure 3b). Next, we tested whether the modulation of glycolysis affected CM proliferation and found that Tg(myl7:pdha1aSTA-P2A-tdTomato) or Tg(myl7:pdk3b-P2A-tdTomato) larvae did not exhibit a significant change compared to WT in the percentage of mVenus-gmnn+ CMs (Figure 3—figure supplement 1b). Furthermore, we examined whether the modulation of glycolysis affected CM morphology in rat neonatal CMs in culture and found that Pdk3 overexpression led to the induction of membrane protrusions (Figure 3d and d’), as well as cell-cell junction rearrangements (Figure 3d–d”). Similar effects were also observed following Erbb2 overexpression (Figure 3e–e”). Together, these data suggest that CM morphological changes regulated by glycolysis are important for delamination.
In order to further assess the role of glycolysis in trabeculation, we focused on zebrafish pkm2 to analyze a glycolytic enzyme mutant model. Loss of PKM2 has been shown to impair glycolysis in endothelial cells (Stone et al., 2018), and PKM2 expression has been associated with glycolysis and cell growth in cancer cells (Christofk et al., 2008). Moreover, in rat neonatal CMs, Erbb2 OE upregulated Pkm2 (Figure 2b and c). Mammalian Pkm encodes two splice variants (M1 and M2 isoforms); PKM2 plays an important role in glycolysis, while PKM1 promotes OXPHOS (Christofk et al., 2008; Lunt et al., 2015; Zheng et al., 2016). Zebrafish pkma2, a splice variant of pkma, and pkmb are the orthologues of mammalian Pkm2 (Stone et al., 2018). During early development, pkma is expressed in the heart, head, spinal cord and blood vessels, while pkmb is highly expressed in the somites (Figure 4—figure supplement 1a). At later stages, pkmb becomes clearly expressed in the heart (Figure 4—figure supplement 1b; Gunawan et al., 2019). We examined pkma2; pkmb double mutants in which Pkma1, which drives pyruvate metabolism via OXPHOS, remains intact, and found that loss of pkma2 and pkmb impaired trabeculation (Figure 4—figure supplement 1c). We did not find evidence for increased CM apoptosis in pkma2; pkmb double mutants compared to WT (Figure 4—figure supplement 1d). In order to examine the CM-specific role of pkma2 and pkmb in trabeculation, we performed cell transplantation experiments whereby pkma2; pkmb double heterozygous and double mutant cells were transplanted into WT embryos (Figure 4a). We found a significantly lower percentage of double mutant versus double heterozygous CMs in the trabecular layer of mosaic hearts (Figure 4b–c), indicating the importance of these genes in trabeculation. We also counted the number of trabecular CMs in these chimeric hearts and observed no significant deviation from WT (Figure 4—figure supplement 1e). Altogether, these results indicate that glycolysis plays important and CM-autonomous roles during trabeculation.
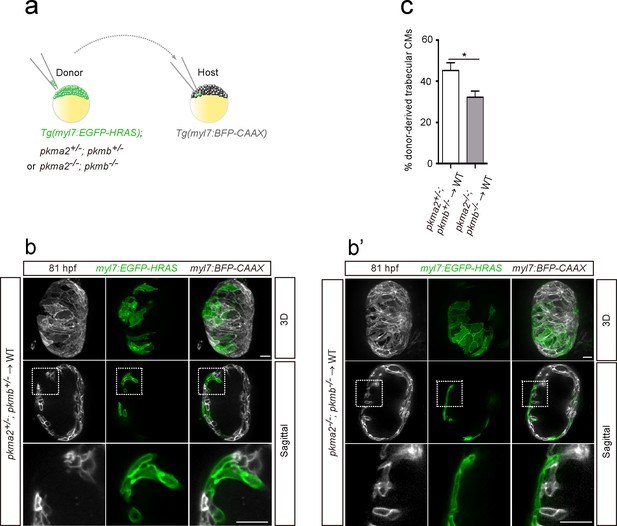
Loss of pkm2 impairs cardiac trabeculation.
(a) Schematic of the transplantation experiment. (b, b’) 3D and mid-sagittal section images of chimeric hearts using pkma2+/-; pkmb+/-; Tg(myl7:EGFP-HRAS) (b) and pkma2-/-; pkmb-/-; Tg(myl7:EGFP-HRAS) (b’) cells as donors; magnified view of area in white boxes shown below. (c) Percentage of donor-derived trabecular CMs (n = 10 ventricles). Error bars, s.e.m.; *p<0.05 by two-tailed unpaired t-test. Scale bars, 20 μm.
Discussion
During trabeculation, CMs exhibit membrane protrusions (Staudt et al., 2014) and rearrange their cell-cell junctions (Cherian et al., 2016; Miao et al., 2019). Our 3D single CM imaging clearly reveals that CMs that enter the trabecular layer change their shape, similar to migrating cells, and lose cell-cell adhesion, indicating that they undergo phenotypic changes. Epithelial cells exhibit cellular plasticity as they change shape, and lose cell-cell adhesion and apicobasal polarity - a phenotypic transformation called epithelial to mesenchymal transition (EMT) (Nieto, 2013; Ye and Weinberg, 2015; Varga and Greten, 2017). Recent studies suggest that endothelial cells can also undergo phenotypic changes towards mesenchymal-like cells (Markwald et al., 1977; Zeisberg et al., 2007; Pearson, 2015; Dejana et al., 2017; Kovacic et al., 2019). Before the onset of trabeculation, compact layer CMs exhibit apicobasal polarity, and then some of them depolarize and subsequently delaminate to seed the trabecular layer (Jiménez-Amilburu et al., 2016). Notably, ERBB2 signaling, which is essential for trabeculation (Lee et al., 1995; Liu et al., 2010), induces EMT in breast cancer cells (Carpenter et al., 2015; Ingthorsson et al., 2016). Altogether, these data indicate that during trabeculation CMs undergo an EMT-like process triggered by ERBB2 signaling.
Cardiac metabolism is essential for energy production to sustain continuous cardiac contractions (Doenst et al., 2013). However, the role of metabolism during cardiac development remains unclear. Our study reveals that glycolysis regulates CM behavior during cardiac wall morphogenesis. Glycolysis enables rapid production of ATP to meet the high-energy demands of cell proliferation and migration in developing tissues, cancer cells and endothelial cells (Lunt and Vander Heiden, 2011; Liberti and Locasale, 2016; Potente and Carmeliet, 2017). Moreover, glycolytic intermediates are utilized to produce biomass including proteins and nucleic acids, further supporting these processes (DeBerardinis et al., 2008; Potente and Carmeliet, 2017). In addition, excessive glycolysis is associated with EMT in cancer cells (Peppicelli et al., 2014; Huang and Zong, 2017; Morandi et al., 2017). These findings indicate that glycolysis could regulate CM behavior in several different ways, and it will be interesting to dissect these processes further.
Materials and methods
Reagent type (species) or resource | Designation | Source or reference | Identifiers | Additional information |
---|---|---|---|---|
Genetic reagent (Danio rerio) | pkma2s717 | Stone et al., 2018 | ||
Genetic reagent (Danio rerio) | pkmbs718 | Stone et al., 2018 | ||
Gene (Danio rerio) | pdk3b | NM_001080688 | ||
Gene (Danio rerio) | pdha1a | NM_213393 | ||
Gene (Rattus norvegicus) | Pdk3 | NM_001106581 | ||
Gene (Homo sapiens) | ERBB2 | NM_004448 | ||
Antibody | anti-Cardiac Troponin I (Goat polyclonal) | Abcam | AB_880622 Cat# ab56357 | IF(1:500) |
Antibody | anti-PKM2 (Rabbit monoclonal) | Cell Signaling | AB_1904096 Cat# D78A4 | IF(1:100) WB(1:1000) |
Chemical compound, drug | Sodium dichloroacetate | Sigma Aldrich | 347795 | |
Software, algorithm | Zen 2012 (Blue edition) | Carl Zeiss Microscopy | Version 1.1.2.0 | |
Software, algorithm | Imaris x64 | Bitplane | Version 9.3.0 | |
Other | DAPI stain | Sigma | D954 | (1 µg/mL) |
Zebrafish
All zebrafish husbandry was performed under standard conditions in accordance with institutional (MPG) and national ethical and animal welfare guidelines. The following transgenic lines and mutants were used: Tg(myl7:EGFP-Has.HRAS)s883 (D'Amico et al., 2007) abbreviated Tg(myl7:EGFP-HRAS), Tg(myl7:LIFEACT-GFP)s974 (Reischauer et al., 2014), Tg(−5.1myl7:DsRed2-NLS)f2Tg (Mably et al., 2003) abbreviated Tg(myl7:nDsRed2), Tg(kdrl:Has.HRAS-mCherry)s896 (Chi et al., 2008) abbreviated Tg(kdrl:HRAS-mCherry), Tg(myl7:cdh2-tdTomato)bns78 (Fukuda et al., 2017), Tg(myl7:BFP-CAAX)bns193 (Guerra et al., 2018), Tg(myl7:mVenus-Gemnn)ncv43Tg (Jiménez-Amilburu et al., 2016), pkma2s717 (Stone et al., 2018) and pkmbs718 (Stone et al., 2018). To generate Tg(myl7:pdk3b-P2A-tdTomato)bns365 and Tg(myl7:pdha1aSTA-P2A-tdTomato)bns366, pdk3b (NM_001080688) and pdha1a (NM_213393) were isolated by RT-PCR and cloned under the control of the myl7 promoter in a vector containing Tol2 elements and two I-SceI restriction enzyme sites. The following primers were used to amplify the cDNA: pdk3b (forward 5’- AAGCAGACAGTGAACAAGCTTCCACCATGAAACTGTTTATCTGCCTACTG-3’ and reverse 5’-TAGCTCCGCTTCCGTCGACTCTGTTGACTTTGTATGTGGAC-3’); pdha1a (forward 5’-AAGCAGACAGTGAACAAGCTTCCACCATGAGAAAGATGCTAACCATAATT-3’ and reverse 5’-TAGCTCCGCTTCCGTCGACGCTGATGGACTTGAGTTTG-3’).
To generate the plasmid encoding an activated form of pdha1a (pdha1aSTA), the equivalent residues for human PDHA1 Ser293 and Tyr301 were replaced by alanine using the following primers: pdha1aSTA (forward 5’- CTATCGTTATCATGGACACGCTATGAGCGACCCAGGAGTCAGCGCCCGCACACGTGAGGAGA-3’ and reverse 5’- TTCCCTCACGTGTGCGGGCGCTGACTCCTGGGTCGCTCATAGCGTGTCCATGATAACGATAG-3’). Plasmids were then injected into one-cell stage embryos with I-SceI (NEB) or Tol2 mRNA.
Quantification of CMs in the trabecular layer
Request a detailed protocolQuantification of trabecular CMs in 77 and 81 hpf heats was performed as previously described (Jiménez-Amilburu et al., 2016) using the ZEN software (ZEISS). Starting from the mid-sagittal plane, we quantified trabecular versus compact layer CMs in the ventricular outer curvature, 12 planes up and 12 planes down at an increment of 1 μm per plane. To quantify donor-derived trabecular CMs in chimeric hearts generated by cell transplantation, we counted the number of donor-derived trabecular and compact layer CMs. Then, the percentage of donor-derived trabecular CMs was calculated by dividing the number of donor-derived trabecular CMs by the total number of donor-derived CMs. Quantification of trabecular CMs in 131 hpf hearts was performed using the ZEN software (ZEISS). Starting from the mid-sagittal plane, we measured the whole myocardial area as well as the trabecular area, 10 μm up and 10 μm down. Three different sagittal planes per heart were measured. The percentage of the trabecular area for each sagittal plane was calculated by dividing the trabecular area by the myocardial area, and the average value was used for the graph.
In situ hybridization
Request a detailed protocolIn situ hybridization was performed as previously described (Thisse and Thisse, 2008). To synthesize pkma (NM_199333) and pkmb (NM_001003488) RNA probes, the following primers were used to amplify the corresponding DNA fragments: pkma (forward 5’- TTGGATCCACCATGTCTCAAACTAAAGCTC-3’ and reverse 5’- TTTGAATTCTTACGGCACTGGGACGACAC-3’); pkmb (forward 5’- TTGGATCCACCATGTCTCAGACAAAGACTA-3’ and reverse 5’- TTTGAATTCTCAAGGCACCACAACGATG’). The DNA fragments were cloned into the pCS2 vector. DIG-labeled RNA probes were synthesized using a DIG RNA labeling kit (Sigma-Aldrich) and MegaScript T7 Transcription Kit (Thermo Fisher Scientific).
Pharmacological treatments
Request a detailed protocolZebrafish embryos were treated with DMSO (control), 30 mM DCA (Sigma-Aldrich) or 5 μM Erbb2 inhibitor (AG1478; Sigma-Aldrich) from 50 hpf to 77 hpf and then analyzed.
TUNEL assay
Request a detailed protocolTo examine apoptosis, an in situ cell death detection kit (Roche) was used.
Immunostaining
Request a detailed protocolRat neonatal CMs were fixed in 4% paraformaldehyde. Anti-cardiac troponin I (CTNI) 1:500 (ab56357, Abcam) and anti-PKM2 1:100 (D78A4, Cell Signaling) were used. After washing with PBS, samples were stained with Alexa-568, Alexa-488 or Alexa-647 secondary antibodies 1:500 (Life Technologies), followed by 4′,6-Diamidine-2′-phenylindole dihydrochloride (DAPI) 1:2000 (Merck) staining to visualize DNA.
Cell culture
Request a detailed protocolRat neonatal (P2-P4) CMs were isolated and cultured as previously described (Fukuda et al., 2017). Cells were plated onto 0.1% gelatin-coated (Sigma) plates and cultured in DMEM/F12 (Gibco) supplemented with 5% horse serum, L-glutamine, Na-pyruvate, penicillin and streptomycin at 37°C and 5% CO2. Adenovirus vectors for transfection into CMs were generated using the AdEasy system (Agilent Technologies). To generate adenovirus vectors encoding PDK3 (NM_001106581) or ERBB2 (NM_004448), the following primers were used to amplify Pdk3 from rat neonatal CM or ERBB2 from Addgene clone # 39321: Pdk3 (forward 5’-TAGAGATCTGGTACCGTCGACCACCATGCGGCTCTTCTACCG-3’ and reverse 5’-GGATATCTTATCTAGAAGCTTCTAGAAAGTTTTATTACTCTTGATCTTGTCC-3’); ERBB2 (forward 5’-TAGAGATCTGGTACCGTCGACGCGGCCGCACCACCATGTATCCATATGATGTTCCAGATTATGCTATGGAGCTGGCGGCCTTG-3’ and reverse 5’-GGATATCTTATCTAGAAGCTTTCACACTGGCACGTCCAG).
Imaging
Request a detailed protocolZebrafish embryos and larvae were anesthetized with 0.2% tricaine and mounted in 1% low-melting agarose. A Zeiss spinning disk confocal microscope system (CSU-X1, Yokogawa) and ORCA-flash4.0 sCMOS camera (Hamamatsu) was used to acquire images. 3D images were processed using Imaris (Bitplane). Circularity was measured using ImageJ (NIH).
Western blotting
Request a detailed protocolProtein expression levels were analyzed by western blotting as previously described (Fukuda et al., 2017). In brief, proteins were extracted with lysis buffer (150 mM Tris-HCl pH 7.5, 150 mM NaCl, 1% Triton X-100, 0.2% SDS, 1 mM EDTA, 5 mM NaF, 0.1 mM orthovanadate, 1 mM phenylmethylsulfonyl fluoride and 1 μg/ml aprotinin). Proteins were separated by SDS-PAGE. The following primary antibodies were used: anti-PKM2 1:1000 (D78A4, Cell Signaling) and anti-alpha-Tubulin 1:1000 (T6199, Sigma).
qPCR
Request a detailed protocolRat neonatal CMs were transfected with adenovirus vectors encoding genes of interest. 24 hr after transfection, total RNA was extracted. Zebrafish embryos and larvae were treated with DMSO or Erbb2 inhibitor from 55 to 106 hpf, and then the hearts were isolated to extract total RNA. A miRNeasy Mini kit (Qiagen) was used for total RNA extraction and cDNA was synthesized using a SuperScript Second Strand kit (Life Technologies). A CFX Connect Real-Time system (Bio-Rad) and DyNAmo colorFlash SYBR green qPCR kit (ThermoFisher Scientific) were used. Primer sequences are shown in Figure 2—source data 2 and Ct values in Figure 2—source data 3.
Metabolic assays
Request a detailed protocolThe extracellular acidification rate (ECAR) was measured using a Seahorse XFe96 analyzer (Seahorse Bioscience) following manufacturer’s protocol. Rat neonatal CMs were seeded at 30,000 cells/well on to 0.1% gelatin-coated XFe96 microplates (Agilent Technologies) in DMEM/F12 (Gibco) containing 10% fetal bovine serum (FBS), L-glutamine, Na-pyruvate, penicillin and streptomycin at 37°C and 5% CO2. After 24 hr of culture, the medium was replaced with serum-free medium. Then, cells were transfected with adenovirus vectors encoding genes of interest or a mock adenovirus vector, or treated with NRG1 (100 ng/ml; Abcam). 24 hr after transfection or NRG1 treatment, cells were maintained in non-buffered assay medium (Agilent Technologies) in a non-CO2 incubator 1 hr prior to the assay. A glycolysis stress test kit (Seahorse Bioscience) was used to monitor ECAR where baseline measurements were made followed by sequential injection of glucose (10 mM), oligomycin (2 μM), and 2-DG (100 mM).
Data availability
All data in this study are included in the manuscript and supporting files.
References
-
Foxn4 directly regulates tbx2b expression and atrioventricular canal formationGenes & Development 22:734–739.https://doi.org/10.1101/gad.1629408
-
The molecular basis of endothelial cell plasticityNature Communications 8:14361.https://doi.org/10.1038/ncomms14361
-
Cardiac metabolism in heart failure: implications beyond ATP productionCirculation Research 113:709–724.https://doi.org/10.1161/CIRCRESAHA.113.300376
-
Metabolic remodeling in early development and cardiomyocyte maturationSeminars in Cell & Developmental Biology 52:84–92.https://doi.org/10.1016/j.semcdb.2016.02.004
-
Genetics of congenital heart disease: the glass half emptyCirculation Research 112:707–720.https://doi.org/10.1161/CIRCRESAHA.112.300853
-
Tyr-301 phosphorylation inhibits pyruvate dehydrogenase by blocking substrate binding and promotes the warburg effectJournal of Biological Chemistry 289:26533–26541.https://doi.org/10.1074/jbc.M114.593970
-
Proteolysis regulates cardiomyocyte maturation and tissue integrationNature Communications 8:14495.https://doi.org/10.1038/ncomms14495
-
Notch signaling is essential for ventricular chamber developmentDevelopmental Cell 12:415–429.https://doi.org/10.1016/j.devcel.2006.12.011
-
Focal adhesions are essential to drive zebrafish heart valve morphogenesisThe Journal of Cell Biology 218:1039–1054.https://doi.org/10.1083/jcb.201807175
-
Aberrant Cancer metabolism in epithelial-mesenchymal transition and Cancer metastasis: mechanisms in Cancer progressionCritical Reviews in Oncology/Hematology 115:13–22.https://doi.org/10.1016/j.critrevonc.2017.04.005
-
Endothelial to mesenchymal transition in Cardiovascular Disease: JACC State-of-the-Art ReviewJournal of the American College of Cardiology 73:190–209.https://doi.org/10.1016/j.jacc.2018.09.089
-
Oncogenic role of PDK4 in human Colon cancer cellsBritish Journal of Cancer 116:930–936.https://doi.org/10.1038/bjc.2017.38
-
The warburg effect: how does it benefit Cancer cells?Trends in Biochemical Sciences 41:211–218.https://doi.org/10.1016/j.tibs.2015.12.001
-
A dual role for ErbB2 signaling in cardiac trabeculationDevelopment 137:3867–3875.https://doi.org/10.1242/dev.053736
-
Induction of pyruvate dehydrogenase Kinase-3 by Hypoxia-inducible Factor-1 promotes metabolic switch and drug resistanceThe Journal of Biological Chemistry 283:28106–28114.https://doi.org/10.1074/jbc.M803508200
-
Aerobic glycolysis: meeting the metabolic requirements of cell proliferationAnnual Review of Cell and Developmental Biology 27:441–464.https://doi.org/10.1146/annurev-cellbio-092910-154237
-
Structural development of endocardial cushionsAmerican Journal of Anatomy 148:85–119.https://doi.org/10.1002/aja.1001480108
-
Cardiac chamber formation: development, genes, and evolutionPhysiological Reviews 83:1223–1267.https://doi.org/10.1152/physrev.00006.2003
-
Plasticity of adult endothelium: how frequent and to what extent?Cardiovascular Research 108:319–320.https://doi.org/10.1093/cvr/cvv240
-
A naturally occurring HER2 carboxy-terminal fragment promotes mammary tumor growth and metastasisMolecular and Cellular Biology 29:3319–3331.https://doi.org/10.1128/MCB.01803-08
-
Contribution of acidic melanoma cells undergoing epithelial-to-mesenchymal transition to aggressiveness of non-acidic melanoma cellsClinical & Experimental Metastasis 31:423–433.https://doi.org/10.1007/s10585-014-9637-6
-
Dependence of cardiac trabeculation on neuregulin signaling and blood flow in zebrafishDevelopmental Dynamics 240:446–456.https://doi.org/10.1002/dvdy.22526
-
The link between angiogenesis and endothelial metabolismAnnual Review of Physiology 79:43–66.https://doi.org/10.1146/annurev-physiol-021115-105134
-
ErbB signaling in cardiac development and diseaseSeminars in Cell & Developmental Biology 21:929–935.https://doi.org/10.1016/j.semcdb.2010.09.011
-
Developmental patterning of the myocardiumThe Anatomical Record 258:319–337.https://doi.org/10.1002/(SICI)1097-0185(20000401)258:4<319::AID-AR1>3.0.CO;2-O
-
Uncovering the molecular and cellular mechanisms of heart development using the zebrafishAnnual Review of Genetics 46:397–418.https://doi.org/10.1146/annurev-genet-110711-155646
-
Cell plasticity in epithelial homeostasis and tumorigenesisNature Cell Biology 19:1133–1141.https://doi.org/10.1038/ncb3611
-
Epithelial-Mesenchymal plasticity: a central regulator of Cancer progressionTrends in Cell Biology 25:675–686.https://doi.org/10.1016/j.tcb.2015.07.012
-
Endothelial-to-mesenchymal transition contributes to cardiac fibrosisNature Medicine 13:952–961.https://doi.org/10.1038/nm1613
-
Molecular mechanism of ventricular trabeculation/compaction and the pathogenesis of the left ventricular noncompaction cardiomyopathy (LVNC)American Journal of Medical Genetics Part C: Seminars in Medical Genetics 163:144–156.https://doi.org/10.1002/ajmg.c.31369
-
Overexpression of pyruvate dehydrogenase kinase 4 in heart perturbs metabolism and exacerbates calcineurin-induced cardiomyopathyAmerican Journal of Physiology-Heart and Circulatory Physiology 294:H936–H943.https://doi.org/10.1152/ajpheart.00870.2007
Article and author information
Author details
Funding
Max-Planck-Gesellschaft (Open-access funding)
- Didier YR Stainier
The funders had no role in study design, data collection and interpretation, or the decision to submit the work for publication.
Acknowledgements
We thank Beate Grohmann, Radhan Ramadass, Srinath Ramkumar, Simon Perathoner, Carmen Büttner, Nana Fukuda and Sharon Meaney-Gardian for help and support, Arica Beisaw, Ruben Marin-Juez, Josephine Gollin and Rashmi Priya for comments on the manuscript, and Jeroen Bakkers for communication. This work was supported in part by funds from the Max Planck Society to DYRS.
Ethics
Animal experimentation: All zebrafish husbandry was performed under standard conditions in accordance with institutional (MPG) and national ethical and animal welfare guidelines approved by the ethics committee for animal experiments at the Regional Board of Darmstadt, Germany (permit numbers B2/1017, B2/1041 and B2/1159).
Copyright
© 2019, Fukuda et al.
This article is distributed under the terms of the Creative Commons Attribution License, which permits unrestricted use and redistribution provided that the original author and source are credited.
Metrics
-
- 3,456
- views
-
- 585
- downloads
-
- 25
- citations
Views, downloads and citations are aggregated across all versions of this paper published by eLife.
Citations by DOI
-
- 25
- citations for umbrella DOI https://doi.org/10.7554/eLife.50161
Download links
Downloads (link to download the article as PDF)
Open citations (links to open the citations from this article in various online reference manager services)
Cite this article (links to download the citations from this article in formats compatible with various reference manager tools)
Further reading
-
- Developmental Biology
Experiments in zebrafish have shed new light on the relationship between development and regeneration in the heart.