RNA fusion in human retinal development
Abstract
Chimeric RNAs have been found in both cancerous and healthy human cells. They have regulatory effects on human stem/progenitor cell differentiation, stemness maintenance, and central nervous system development. However, whether they are present in human retinal cells and their physiological functions in the retinal development remain unknown. Based on the human embryonic stem cell-derived retinal organoids (ROs) spanning from days 0 to 120, we present the expression atlas of chimeric RNAs throughout the developing ROs. We confirmed the existence of some common chimeric RNAs and also discovered many novel chimeric RNAs during retinal development. We focused on CTNNBIP1-CLSTN1 (CTCL) whose downregulation caused precocious neuronal differentiation and a marked reduction of neural progenitors in human cerebral organoids. CTCL is universally present in human retinas, ROs, and retinal cell lines, and its loss-of-function biases the progenitor cells toward retinal pigment epithelial cell fate at the expense of retinal cells. Together, this work provides a landscape of chimeric RNAs and reveals evidence for their critical role in human retinal development.
Editor's evaluation
The primary goal of this paper is to profile expression of chimeric RNAs in human retinal organoids throughout development. The fundamental findings provide an important step forward in understanding retinogenesis specifically and neurogenesis, generally. The compelling evidence unveils a previously unrecognized significant role of chimeric RNA CTCL in human retinal development.
https://doi.org/10.7554/eLife.92523.sa0Introduction
The human retina is a laminar structure with a large number of different component cells that form morphologically and functionally distinct circuits. They work in parallel and in combination to produce complex visual output (Hoon et al., 2014). During retinogenesis, different subtypes of neurons are generated from the same group of retinal progenitor cells (RPCs) and precisely self-assemble into a functionally mature retina (Masland, 2012).
Dissecting the molecular mechanisms of human retinogenesis and functional maintenance has always been a focus and a challenging issue, which is particularly important for the treatment of human retinal diseases such as macular degeneration. A few groups have systematically elucidated the transcriptomics, chromatin accessibility, and proteomic dynamics during human and mouse retinogenesis (Huang et al., 2023), and comprehensively described the similarities and differences during this process. Lu et al. identified an unexpected role for ATOH7 expression in the regulation of photoreceptor specification during late retinogenesis as well as the enriched bivalent modification of H3K4me3 and H3K27me3 in human but not in murine retinogenesis (Lu et al., 2020), further underscoring the limitations of using mouse models to study the human retina and transcriptional regulation in human retinogenesis.
Difficulties in obtaining normal human retinal tissue have hampered studies of human retinal development. The emergence of retinal organoids (ROs) could circumvent this problem (Cheng et al., 2023; Jin et al., 2019). Many groups have used ROs to conduct studies related to human retinal development and have solved a basket of previously unsolvable problems, confirming the high similarity between ROs and human retina in terms of molecular features, cell types, and developmental rates (Cowan et al., 2020; Lu et al., 2020; Xie et al., 2020). Our group has extensive experience in RO culture and has conducted a series of studies using ROs (Deng et al., 2018; Ma et al., 2022; Pan et al., 2020; Zhang et al., 2021). For example, together with other groups, we have used ROs to confirm that retinoblastoma tumor cells are derived from cone precursor cells (Clevers, 2016; Jin et al., 2019; Li et al., 2022; Liu et al., 2021; Liu et al., 2020).
Chimeric RNAs are the ligation products of two or even more DNA or RNA sequences before or after transcription. They can be further translated into proteins in addition to their RNA form, increasing the richness of the transcriptome and proteome. Previous studies of chimeric RNAs have focused on tumors and larger tissues and organs, and found that chimeric RNAs are widely present in human normal and tumor tissues as part of the transcriptome and can regulate the activities of individual cells (Elfman and Li, 2018; Hu et al., 2018; Mertens et al., 2015; Singh et al., 2020). It has been shown that chimeric RNAs in normal human cortex increase with age and show differences in individual cells and tissues (Mehani et al., 2020), highlighting the critical role of chimeric RNAs in central nervous system (CNS) development and cell lineage maintenance. In 2021, Luo’s group found that downregulation of CTNNBIP1-CLSTN1 (CTCL) affects the growth of cerebral organoids, causing premature neuronal differentiation, and a marked reduction of neural progenitors (Ou et al., 2021). As retina is a part of the CNS, we hypothesize that CTCL is also present in the retina and plays an important role in human retinal development.
This study aims to investigate the molecular mechanism of human retinal development and function maintenance from a new perspective of chimeric RNAs. We used human embryonic stem cell (hESC)-derived ROs and performed RNA sequencing. We found that chimeric RNAs were expressed at all stages of RO development. Chimeric RNA CTCL is widely expressed in the human retina but not in the murine retina, and is critical for the differentiation of RPCs into neural retina (NR) or retinal pigment epithelium (RPE). RPCs with a relatively high level of CTCL expression will differentiate into NR, while those with a relatively low level of CTCL will differentiate toward RPE. These results provide important evidence for the physiological function of chimeric RNAs in human retinogenesis.
Results
Chimeric RNAs are present throughout the retinogenesis
To explore the chimeric RNAs in the developing human retina, we cultured ROs according to the methods described previously (Kim et al., 2019; Lowe et al., 2016). On day 0 (D0) of differentiation, hESCs in a good growth state were dissociated into individual cells and seeded at a certain cell density in V-shaped low-adhesion culture dishes so that the cells would aggregate into clusters to induce the neuroepithelial formation. At D30 of differentiation, the RO outermost layer with translucent NR structures was visible. As the differentiation time prolonged, the NR gradually became denser. At D105 of differentiation, ROs could be seen with the formation of RPE. At D120 of differentiation, the outer segments of photoreceptor cells were seen on the surface of ROs. We collected organoids from 0 to 120 days for RNA sequencing (Figure 1, top and middle panels). Using FusionCatcher software, we examined chimeric RNAs in human ROs covering eight developmental stages from D0, 30, 45, 60, 75, 90, and 105–120, corresponding to in vivo developmental stages D0, 30, 45, 60, 75, 90, 105, and 120 (Cowan et al., 2020), respectively. Each stage included two to three biological replicates (a total of N = 22), and each replicate contained approximately 100 ROs. To reduce false positives, splicing events were considered as positive chimeric RNA candidates only if spanning unique reads were detected at least once (Figure 1, bottom panel).
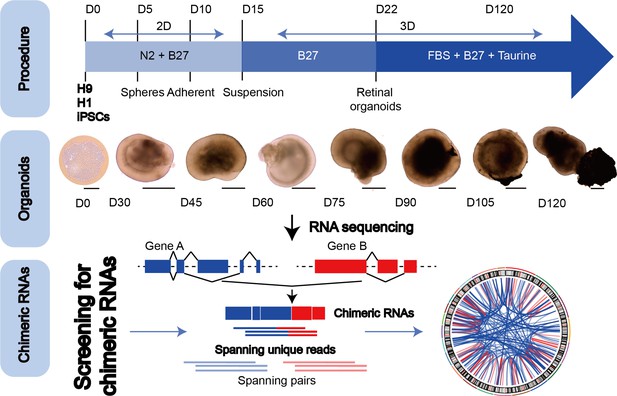
The general scheme of detection of chimeric RNAs.
(Top panel) Procedure for the generation of human retinal organoids (ROs) from pluripotent stem cells. (Middle panel) D0–120 human ROs were used for bulk RNA sequencing. Scale bars: 200 μm (D0) and 400 μm (D30–D120). (Bottom panel) Illustration of chimeric RNA screening process and criteria by FusionCatcher.
Chimeric RNAs were continuously expressed during RO development (Figure 2A). Chimeric events were categorized according to the locations of the parental genes on the chromosome, either inter- or intra-chromosomal. While the number of intra-chromosomal chimeric RNAs increased with the development of ROs (r2 = 0.93, p = 0.00076, Pearson’s correlation analysis), there was no such trend for inter-chromosomal chimeric RNAs (Figure 2B,C). Chimeric events can also be categorized according to the predicted effect (Figure 3—figure supplement 1). In the top three categories, the number of ‘In-frame’ chimeric RNAs was 11.2%, followed by ‘CDS(truncated)_UTR’ (10.7%) and ‘UTR_UTR’ (10.7%) (Figure 3A). We further classified chimeric events according to the type of their parental genes. The majority of chimeric RNAs were formed between two protein-coding genes (61.9%) (Figure 3B).
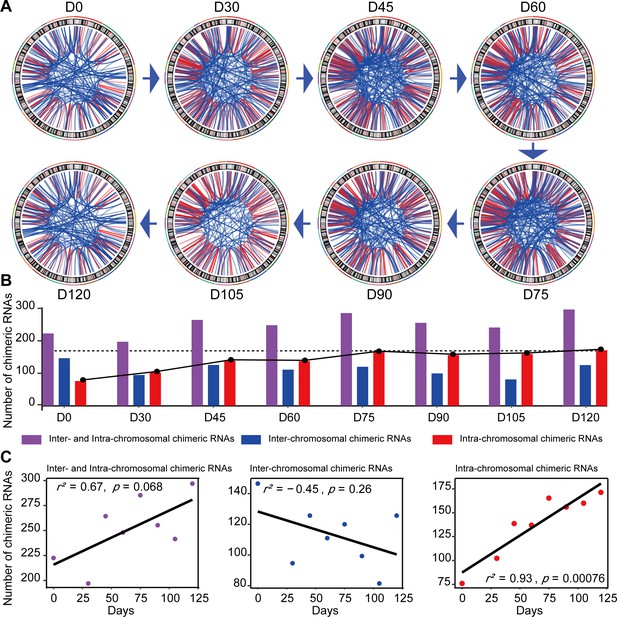
Expression of chimeric RNAs in the developing human retinal organoids.
(A) Circos plots of genomic distribution of chimeric RNA parental genes observed in this work. Red lines indicate parental genes located in the same chromosome. Blue lines indicate parental genes located in different chromosomes. The outermost colored lines of circos plots represent chromosomes. (B) Types of chimeric RNAs based on parental genes’ genomic distribution. (C) Pearson’s correlation analysis of the number of chimeric RNAs and developmental stages.
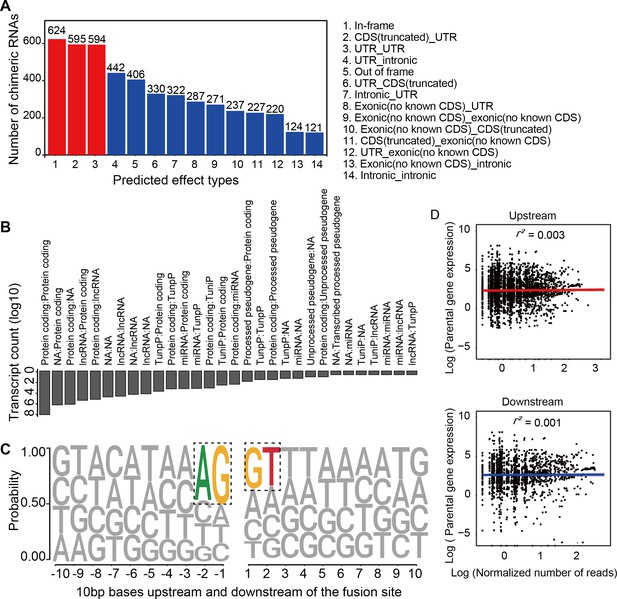
Characterization of chimeric RNAs in the developing human retinal organoids.
(A) Top 14 types and corresponding numbers of chimeric RNAs based on predicted effects. (B) Biotype quantification of parental gene combinations in all samples. TunpP: transcribed unprocessed pseudogene; TuniP: transcribed unitary pseudogene. (C) Motifs consisting of 20 bp DNA sequences around the fusion site. (D) Spearman correlation analysis of expression level of chimeric RNAs and their parental genes, p > 0.05.
Next, we examined the motifs covering 10 bp sequences immediately upstream or downstream of the fusion site of parental genes and found that the canonical AG/GT donor–acceptor motif had the highest position weight (Figure 3C). In addition, we also found that the expression of the chimeric RNAs did not correlate with the expression of the parental genes (Figure 3D). These results were consistent with previous reports (Ou et al., 2021; Singh et al., 2020), indicating that the procedure for screening chimeric RNAs was reliable and that these chimeric RNAs do not arise randomly, suggesting that they may play a role in the normal development and physiological activity of the retina.
CTCL is present in the human retina and at all the stages of RO development
CTCL has been shown to have a regulatory role in human cerebral development. Similarly, four isoforms of CTCL, joined by alternative splicing of the parent pre-mRNAs, were detected in the ROs by RNA-seq. In-frame CTCL can be further translated into chimeric proteins, and we think that it plays a more critical role than the other three isoforms. It was dynamically expressed during RO development and there were two obvious higher expression timepoints at D60 and D120 (Figure 4A, B). Retinal cells like cones, rods, and horizonal cells had been generated around D120, so we chose D60 when most retinal cells were progenitors. To further validate the presence of CTCL in the developing human ROs, we extracted total RNAs from D60 human ROs, followed by reverse transcription and Sanger sequencing using specific primers to amplify the fragments containing fusion sites between CTNNBIP1 and CLSTN1 (Figure 4—source data 1; Figure 4—source data 2; Figure 4C,D and Key resources table). At D60, the In-frame, UTR_CDS(truncated)-1, and CDS(truncated)Intronic CTCL isoforms were confirmed but UTR_CDS(truncated)-2 CTCL was not detected.
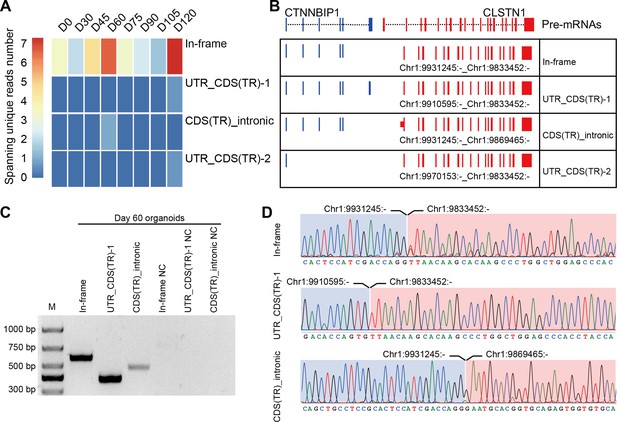
Four isoforms of CTCL are present in the retinal organoids (ROs).
(A) Heatmap of CTCL’s spanning unique reads of each isoform in the indicated stages. UTR_CDS(TR)-1: UTR_CDS(truncated)-1; CDS(TR)Intronic: CDS(truncated)Intronic; UTR_CDS(TR)-2: UTR_CDS(truncated)-2. (B) Schematic diagram of the structures of the four isoforms of CTCL. Blue represents upstream parental gene, red represents downstream parental gene. (C) Four isoforms of CTCL in D60 ROs were validated by quantitative reverse transcription polymerase chain reaction (qRT-PCR). (D) Sanger sequencing to verify three isoforms of CTCL.
-
Figure 4—source data 1
Source data for Figure 4C.
- https://cdn.elifesciences.org/articles/92523/elife-92523-fig4-data1-v3.pdf
-
Figure 4—source data 2
Source data for Figure 4C (labelled).
- https://cdn.elifesciences.org/articles/92523/elife-92523-fig4-data2-v3.pdf
To further validate the widespread presence of the In-frame CTCL in vivo and in vitro, we examined it in various samples including mouse retinas, three human induced pluripotent stem cell (iPSC)-cultured ROs, and human retinas. Indeed, the In-frame CTCL was consistently identified in human retinas, iPSCs1/iPSCs2/iPSCs3-derived ROs, and H9 cells, but not in the mouse retinas (Figure 5—source data 1; Figure 5—source data 2; Figure 5—source data 3; Figure 5—source data 4; Figure 5). Moreover, we also screened CTCL using the retinal transcriptome sequencing data available in the Gene Expression Omnibus (GEO) database, all of the nine human retinal samples yielded uniformly positive results (Table 1; Sun et al., 2019; Zauhar et al., 2022). These results suggest that CTCL is universally generated in human retinas in vivo, ROs, and retinal cell lines in vitro, but not a phenomenon of the H9 cell line exclusively. The dynamic presence of CTCL, especially the In-frame isoform, suggests that it may play a vital role in human retinal development.
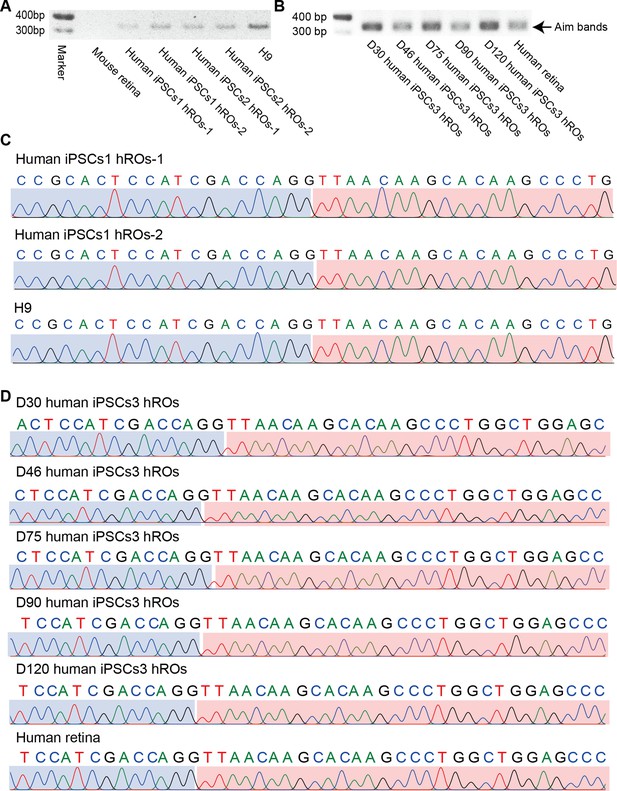
In-frame CTCL is common in human retinas and retinal organoids (ROs).
(A) The qRT-PCR validation of the In-frame CTCL in the mouse retina, iPSCs1/iPSCs2-derived ROs, and H9 cells. (B) The qRT-PCR validation of In-frame CTCL in the iPSCs3-derived ROs and human retina. (C) Verification of the In-frame CTCL in the mouse retina, iPSCs1/iPSCs2-derived ROs and H9 cells by Sanger sequencing. (D) Verification of the In-frame CTCL in the iPSCs3-derived ROs and human retinas by Sanger sequencing.
-
Figure 5—source data 1
Source data for Figure 5A.
- https://cdn.elifesciences.org/articles/92523/elife-92523-fig5-data1-v3.pdf
-
Figure 5—source data 2
Source data for Figure 5A (labelled).
- https://cdn.elifesciences.org/articles/92523/elife-92523-fig5-data2-v3.pdf
-
Figure 5—source data 3
Source data for Figure 5B.
- https://cdn.elifesciences.org/articles/92523/elife-92523-fig5-data3-v3.pdf
-
Figure 5—source data 4
Source data for Figure 5B (labelled).
- https://cdn.elifesciences.org/articles/92523/elife-92523-fig5-data4-v3.pdf
CTCL is detected in multiple healthy human retinas by RNA-seq.
Data source | Samples | Gene 1 symbol | Gene 2 symbol | Fusion point for gene 1 | Fusion point for gene 2 |
---|---|---|---|---|---|
PMID: 30874468 (Sun et al., 2019) Data published by our lab | Human retina | CTNNBIP1 | CLSTN1 | 1:9871187:- | 1:9773394:- |
PMID: 30874468 (Sun et al., 2019) Data published by our lab | Human retina | CTNNBIP1 | CLSTN1 | 1:9871187:- | 1:9773394:- |
PMID:35784369 (Zauhar et al., 2022) SRR22315404 | Human retina | CTNNBIP1 | CLSTN1 | 1:9871187:- | 1:9773394:- |
PMID:35784369 (Zauhar et al., 2022) SRR22315407 | Human retina | CTNNBIP1 | CLSTN1 | 1:9871187:- | 1:9773394:- |
PMID:35784369 (Zauhar et al., 2022) SRR22315408 | Human retina | CTNNBIP1 | CLSTN1 | 1:9871187:- | 1:9773394:- |
PMID:35784369 (Zauhar et al., 2022) SRR22315412 | Human retina | CTNNBIP1 | CLSTN1 | 1:9871187:- | 1:9773394:- |
PMID:35784369 (Zauhar et al., 2022) SRR22315419 | Human retina | CTNNBIP1 | CLSTN1 | 1:9871187:- | 1:9773394:- |
PMID:35784369 (Zauhar et al., 2022) SRR22315420 | Human retina | CTNNBIP1 | CLSTN1 | 1:9871187:- | 1:9773394:- |
PMID:35784369 (Zauhar et al., 2022) SRR22315424 | Human retina | CTNNBIP1 | CLSTN1 | 1:9871187:- | 1:9773394:- |
CTCL knockdown obstructed NR differentiation but prompted the RPE differentiation
According to the chimeric CTCL RNA expression levels (Figure 4A), we focused on the D60 In-frame CTCL to explore its function. A CRX-tdTomato reporter hPSC line was used in the CTCL-knockdown experiment. This cell line is derived from H9 cells and it has been shown that tdTomato follows CRX expression spatiotemporally and the cells had the same organoid yield as H9 cells, suggesting that genetic modification of H9 did not affect its developmental process (Pan et al., 2020). ROs were differentiated according to the previous report (Lowe et al., 2016; Figure 6—figure supplement 1 and Video 1). Lentiviruses carrying shCTCL (short-hairpin CTCL) or scramble shRNA (see Key resources table) infected the retinal cells at D12. After 3 days, cells were harvested for analysis of knockdown efficiency (Figure 6A,B). Compared with the scramble shRNA group, the In-frame CTCL RNA level was reduced to approximately 70% (Figure 6B). The RNA levels of the parental genes, CTNNBIP1 and CLSTN1, showed no significant differences compared to the scramble shRNA group (Figure 6—figure supplement 2).
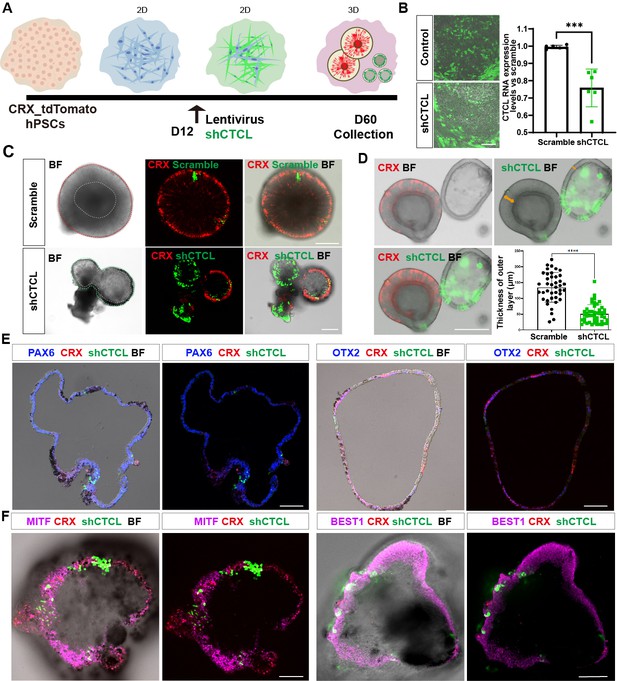
The CTCL knockdown obstructed neural retina’s (NR) cell fates but prompted the retinal pigment epithelial (RPE) differentiation.
(A) A schema illustrated the shRNA experiments. A CRX-tdTomato report line was used in this experiment. The shCTCL or scramble shRNA lentivirus transfected the retinal cells in three independent experiments on D12. All samples for shRNA experiments were collected on day 60, except those for analysis of knockdown efficiency. (B) Samples were obtained 48–72 hr after infection to examine the knockdown efficiency. There were two technical replicates for three independent experiments, t-test, ***, p < 0.001. Data were shown as mean ± standard deviation (SD). Scale bar = 200 μm. (C) D60 scramble shRNA-transfected retinal organoids (ROs) displayed a typical morphology of ROs with the expression of CRX. ShCTCL-transfected organoids showed thinner outer layers with much less CRX expression. BF, bright-field images, same as below.Scale bars = 200 μm. (D) The shCTCL group displayed much thinner outer layers (orange arrows in right panel). The outer layer thickness of 25 organoids from each group was measured for statistics analysis, t-test, ****, p < 0.0001. Data were shown as mean ± SD. Scale bar = 400 μm.(E) The section immunostaining of neural progenitor markers PAX6 and OTX2, and differentiated cell markers CRX, in shCTCL-treated organoids. Scale bars = 100 μm. (F) Whole-mount immunostaining of shCTCL-transfected organoids with CRX, shCTCL, and RPE-specific markers MITF and BEST1. Scale bars = 100 μm.
The 4 days live-cell imaging of retinal organoid differentiation from D3 to D7.
At D60, ROs with scramble shRNA displayed the typical RO morphology with the generation of photoreceptor precursors (CRX+) (Figure 6C, upper panel, live-cell imaging). In the shCTCL group, the organoid morphology changed with a thinner outer layer, and the CRX proteins diminished where the pigments appeared (Figure 6C, lower panel, live-cell imaging). The average thickness of the outer layers in those two groups was calculated (Figure 6D, outer layer indicated with orange arrows, live-cell imaging), and there is a dramatic decrease in the outer layer thickness in the shCTCL group.
To identify the aberrations in cell fate specification caused by CTCL knockdown, we examined the organoids with RPC-specific markers, PAX6 and OTX2. It was shown that PAX6 was highly expressed, but OTX2 was much less, and CRX appeared where GFP (shCTCL) was relatively lower (Figure 6E). CRX is known to be highly expressed in photoreceptors and precursors but low in mature RPE (Lidgerwood et al., 2021). Since the organoids acquired the features of RPE, we hypothesized that the outer layer of CTCL-knockdown organoids switched to the RPE fate. To further confirm this, the organoids were immunostained with RPE-specific markers, MITF, BEST1, and RPE65, which were present in the nucleus, cytoplasm, and intracellular regions, respectively (Figure 6F, Figure 6—figure supplement 3A, C). In contrast, no pigments or RPE-specific markers were observed in the scramble shRNA group, only the PAX6 was detected in the nucleus (Figure 6—figure supplements 3B, D–F). The shCTCL green fluorescence in Figure 6E–F was captured with live imaging and the signal was not amplified with anti-GFP antibodies; as a result, the weaker green signals can not be seen in the images. Taken together, these results indicate that CTCL is essential for the ROs’ differentiation and that CTCL knockdown would promote the RPE cell fate at the expense of neural retinal cells.
The underlying molecular changes in CTCL-knockdown ROs
The above results suggest that CTCL is essential for cell fate conversion in normal retinogenesis. To investigate the underlying molecular regulation, we compared the transcriptomes of shCTCL- and scramble shRNA-treated organoids by bulk RNA-seq. We obtained 3901 differentially expressed genes (DEGs) (shCTCL vs. scramble shRNA, |Log2(fold change)| > 1, p-value <0.05), among which 2720 were upregulated and 1181 downregulated (Figure 7A,B).
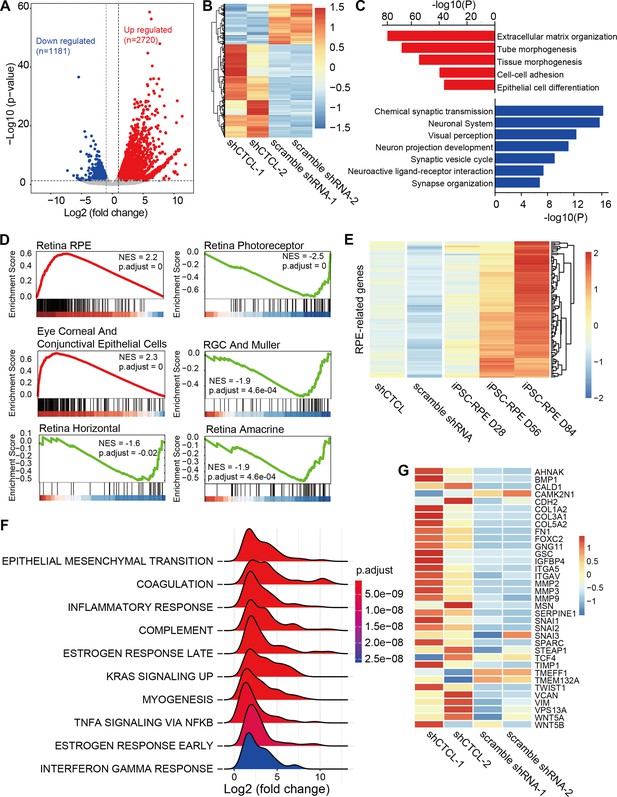
Transcriptomic alterations in CTCL-knockdown retinal organoids.
(A) Volcano plot shows differentially expressed genes (DEGs, p-values <0.05 and |Log2(shCTCL/scramble)| > 1) between shCTCL- and scramble shRNA-treated organoids at D60. (B) Heatmap of DEGs in shCTCL- and scramble shRNA-treated organoids. (C) Functional enrichment analysis of up- and downregulated DEGs. (D) Gene Set Enrichment Analysis (GSEA) results showed the enriched gene sets in shCTCL-treated organoids using the c8 reference gene set. (E) Expression of retinal pigment epithelial (RPE)-related genes in shCTCL- and scramble shRNA-treated organoids. (F) GSEA results showed the enriched gene sets in shCTCL-treated organoids using the hallmark reference gene set. (G) Expression of epithelial–mesenchymal transition (EMT)-related genes in shCTCL- and scramble shRNA-treated organoids.
To investigate the biological pathways affected by the loss-of-function of CTCL, we performed functional enrichment for up- and downregulated DEGs using Metascape. Upregulated DEGs were significantly enriched in pathways associated with extracellular matrix organization, morphogenesis, and epithelial cell differentiation. On the other hand, downregulated DEGs were significantly enriched in the neurogenesis-associated pathways (Figure 7C). To further understand the changes in cell composition between shCTCL- and scramble shRNA-treated organoids, we used the Gene Set Enrichment Analysis (GSEA) to analyze the whole-gene expression lists of the organoids. GSEA analysis with c8 as the reference gene set (Mootha et al., 2003; Subramanian et al., 2005) revealed that genes of RPE, ocular corneal, and conjunctival epithelial cells were significantly upregulated in shCTCL organoids; however, all genes related to neural retinal component cells (photoreceptor cells, Müller glial cells, horizontal cell, amacrine cell, and retinal ganglion cell) were downregulated (Figure 7D). Moreover, the expression pattern of RPE-related genes in shCTCL organoids was closer to that of RPE from induced pluripotent stem cells (Maruotti et al., 2015; Figure 7E and Figure 7—figure supplement 1A). At the same time, CTCL remains in a low expression state during RPE development (Figure 7—figure supplement 1B). These results suggest that CTCL has a positive role in the normal development of ROs and that CTCL deficiency would promote the differentiation of RPCs toward RPE.
To further explore the molecular mechanisms involved in this process, we performed the GSEA analysis using hallmark gene sets (Mootha et al., 2003; Subramanian et al., 2005). The most significantly enriched pathway was epithelial–mesenchymal transition (EMT) (Figure 7F). And most of the EMT-related genes were relatively highly expressed in shCTCL-treated organoids (Figure 7—figure supplement 2) (Figure 7G), which is particularly essential for neural crest delamination in vertebrates and the generation of different tissues during development (Kim et al., 2014b). Therefore, the downregulation of CTCL expression activated the EMT pathway which altered the directions of cell differentiation.
Discussion
The term ‘chimeric RNA’ refers to any transcript composed of transcripts from different parental genes, including gene fusion transcripts (Wu et al., 2019). Chimeric RNAs with two parental gene sequences will further generate new dysregulated wild-type proteins, new fusion proteins, and new non-coding RNAs, expanding the abundance of the transcriptome and proteome. The first chimeric RNA that lacks DNA alterations is AML1-ETO, which is associated with acute myeloid leukemia (Langabeer et al., 1997). Subsequently, cis-splicing between neighboring genes was also found to generate chimeric RNAs, such as the CTCF-sensitive cis-spliced fusion RNAs ADCK4-NUMBL in prostate cancer (Qin et al., 2015). In addition, chimeric RNAs can be generated by long-range inter- and intra-chromosomal trans-splicing (Li et al., 2008). Early phase of studies has focused on the role of chimeric RNAs in cancer, where they contribute to cancer development, and serve as biomarkers and therapeutic targets.
Advances in sequencing technology have facilitated the identification of chimeric RNAs and have stimulated the research in the field of cancer. However, researchers have also found that there are some misunderstandings about chimeric RNAs. For example, PAX3-FOXO1 was initially identified as a biomarker for alveolar rhabdomyosarcoma (Singh et al., 2022), but was later found to be present during normal muscle differentiation. PAX3-FOXO1 is transiently required for muscle lineage commitment (Xie et al., 2019), and can escape miR-495 regulation during muscle differentiation. If PAX3-FOXO1 is not withdrawn at the right timing, it can lead to tumorigenesis. DUS4L-BCAP29 was originally identified in gastric cancer, where it promotes cell proliferation and is therefore considered to have tumorigenic potential (Kim et al., 2014a). DUS4L-BCAP29 was subsequently identified in human non-cancerous tissues (Tang et al., 2017), and its role in promoting cell proliferation is not unique to cancer cells. It is a chimeric RNA present in normal physiology with a function in promoting neural differentiation (Tang et al., 2019). Therefore, studying chimeric RNAs in normal tissues has two implications: first, we can learn more about the normal physiological functions of chimeric RNAs; second, we can establish a control baseline for the discovery of tumor markers.
It is now well established that chimeric RNAs are abundant in normal human tissues and play a regulatory role in cellular activities. In this work, we investigated chimeric RNAs in retina for the first time. We present the expression atlas of chimeric RNAs throughout the developing ROs mimicking human retinas at various development stages. As a recently identified chimeric RNA, and the In-frame CTCL was reported to play a key role in cerebral development (Ou et al., 2021). Using the ROs as a model for loss-of-function experiments, we found that the In-frame CTCL also has a key role in the development of the retina and that CTCL deficiency inhibited NR differentiation but induced the RPE differentiation.
In-frame CTCL is generated by cis-splicing fusion of the first 5 exons of CTNNBIP1 and the last 17 exons of CLSTN1. The 5′ parental gene CTNNBIP1 encodes a receptor for β-catenin, and their binding promotes the catabolism of β-catenin, which in turn puts the Wnt pathway in an inactive state (Fu et al., 2018). Its mutation has been shown to be associated with anterior segment dysgenesis 5 in the human disease database MalaCards (https://www.malacards.org/). CLSTN1 is a member of the calsyntenin family, a subset of the cadherin superfamily. It can mediate the axonal anterograde transport of certain types of vesicles (Nagase et al., 1998). The interesting question is that whether CTCL retains the biparental functions and/or acquires novel functions? Previous report demonstrated that CTCL could fine-tune Wnt signaling to regulate cerebral development (Ou et al., 2021). Therefore, in this study, we had expected CTCL to function by modulating the Wnt signaling pathway or by impacting vesicular trafficking. However, surprisingly, the GSEA and DEG functional enrichment results did not show significant changes in these two biological pathways (Figure 6C, D, F). The differences between the shCTCL- or scramble shRNA-treated organoids were mainly in EMT, extracellular matrix organization, morphogenesis, epithelial cell differentiation, and neurogenesis-associated pathways, suggesting that even the same chimeric RNAs may function differently in close tissues.
In summary, we have identified the chimeric RNAs in the developing human ROs and found that loss-of-function of CTCL inhibited NR differentiation while prompted the RPE differentiation. Although this chimeric RNA has been previously reported in the cerebral cortex and the retina is also part of the CNS, the two tissues differ in morphology, cellular composition, development, and function. Moreover, we have made new discoveries regarding the function and mechanism of CTCL. This study revealed for the first time a key role of chimeric RNAs in human retinal development, providing new insights into the role of chimeric RNAs in regulating tissue development. In the future, we will investigate more unreported chimeric RNAs in retinal development to further illustrate the critical role of chimeric RNAs in human retinal development.
Materials and methods
Reagent type (species) or resource | Designation | Source or reference | Identifiers | Additional information |
---|---|---|---|---|
Cell line (Homo sapiens) | CRX-tdTomato human ES reporter line-H9 | PMID:32831148 | Available upon reasonable request. | |
Cell line (Homo sapiens) | iPSCs1 | PMID:36714839 | Available upon reasonable request. | |
Cell line (Homo sapiens) | iPSCs2 | PMID:35451725 | Available upon reasonable request. | |
Cell line (Homo sapiens) | iPSCs3 | PMID:33970142 | Available upon reasonable request. | |
Antibody | anti-Pax6 (rabbit polyclonal) | Biolegend | Cat.# 862002 RRID: AB_3076431 | Dilution: 1:200 |
Antibody | anti-Ki67 (rabbit polyclonal) | Abcam | Cat.# ab15580 RRID:AB_443209 | Dilution: 1:200 |
Antibody | anti-OTX2 (rabbit monoclonal) | Abcam | Cat.# ab183951 RRID: AB_3076432 | Dilution: 1:200 |
Antibody | anti-HuC/D (mouse monoclonal) | Invitrogen | Cat.# A21271 RRID:AB_221448 | Dilution: 1:100 |
Antibody | anti-SOX2 (mouse monoclonal) | Santa Cruz | Cat.# sc-365823 RRID:AB_10842165 | Dilution: 1:200 |
Antibody | anti-MITF (mouse monoclonal) | Abcam | Cat.# ab3201 RRID:AB_303601 | Dilution: 1:100 |
Antibody | anti-GFAP (mouse monoclonal) | Santa Cruz | Cat.# sc-33673 RRID:AB_627673 | Dilution: 1:200 |
Antibody | anti-Sox9 (rabbit mono/oligo-colonal ) | Invitrogen | Cat.# 711048 RRID:AB_2633109 | Dilution: 1:200 |
Antibody | anti- RxRγ (mouse monoclonal) | Santa Cruz | Cat.# sc-365252 RRID:AB_10850062 | Dilution: 1:100 |
Recombinant DNA reagent | pLenti-U6-shRNA-EF1a-EGFP-T2A-Puro-WPRE | This paper | Available upon reasonable request. | |
Sequence-based reagent | UTR_CDS(truncated)-1 CTCL-forward | Tsingke Biotechnology Co., Ltd | TGCAAAGCCCTTGGAACA | |
Sequence-based reagent | UTR_CDS(truncated)-1 CTCL-reverse | Tsingke Biotechnology Co., Ltd | TCCACTACCACTGCATCAAAG | |
Sequence-based reagent | in-frame CTCL-forward | Tsingke Biotechnology Co., Ltd | TTCCTACTTCTGCCCAGCC | |
Sequence-based reagent | in-frame CTCL-reverse | Tsingke Biotechnology Co., Ltd | AGGCCTGGATGGTGAATGAAT | |
Sequence-based reagent | CDS(truncated)_intronic CTCL-forward | Tsingke Biotechnology Co., Ltd | GAAGAGTCCGGAGGAGATGTA | |
Sequence-based reagent | CDS(truncated)_intronic CTCL-reverse | Tsingke Biotechnology Co., Ltd | ATTCTCTGCCAAGACTTACACC | |
Sequence-based reagent | UTR_CDS(truncated)-2 CTCL-forward | Tsingke Biotechnology Co., Ltd | CTCCTGCTGCTGCTACTG | |
Sequence-based reagent | UTR_CDS(truncated)-2 CTCL-reverse | Tsingke Biotechnology Co., Ltd | GGTCCCTTCCCACAATCATAG | |
Sequence-based reagent | GAPDH forward | Tsingke Biotechnology Co., Ltd | CTCTGACTTCAACAGCGACA | |
Sequence-based reagent | GAPDH reverse | Tsingke Biotechnology Co., Ltd | GTAGCCAAATTCGTTGTCATACC | |
Sequence-based reagent | ShCTCL | Tsingke Biotechnology Co., Ltd | TGCTTGTTAACCTGGTCGA | |
Sequence-based reagent | Scramble | Tsingke Biotechnology Co, Ltd | GCCTAAGGTTAAGTCGCCCTCG | |
Software, algorithm | R (v4.0.3) | R Development Core Team, 2020 | RRID:SCR_001905 | https://www.r-project.org/ |
Software, algorithm | FusionCatcher | Nicorici, 2023 | https://github.com/ndaniel/fusioncatcher | |
Software, algorithm | Hisat2 | Kim and Park, 2022 | https://github.com/DaehwanKimLab/hisat2 | |
Software, algorithm | samtools | Danecek et al., 2023 | https://github.com/samtools/samtools | |
Software, algorithm | featureCounts | Liao et al., 2021 | http://subread.sourceforge.net/featureCounts.html | |
Software, algorithm | ggplot2 | Pedersen, 2022 | https://rdocumentation.org/packages/ggplot2/versions/3.3.6 | |
Software, algorithm | ggseqlogo | Wagih, 2017 | https://github.com/omarwagih/ggseqlogo | |
Software, algorithm | chimeraviz | Lågstad, 2023 | https://www.bioconductor.org/packages/release/bioc/html/chimeraviz.html | |
Software, algorithm | pheatmap | Kolde, 2019 | https://rdocumentation.org/search?q=pheatmap | |
Software, algorithm | clusterProfiler | Yu, 2012 | https://rdocumentation.org/search?q=clusterProfiler |
RNA sequencing and data analysis
Request a detailed protocolA total amount of 1–3 μg RNA per sample was used as input material for the RNA sample preparations. Sequencing libraries were generated using VAHTS Universal V6 RNA-seq Library Prep Kit for Illumina (NR604-01/02) following the manufacturer’s recommendations and index codes were added to attribute sequences to each sample. Briefly, mRNA was purified from total RNA using poly-T oligo-attached magnetic beads. Then we added fragmentation buffer to break the mRNA into short fragments. First-strand cDNA was synthesized using random hexamer primer and RNase H. Second-strand cDNA synthesis was subsequently performed using buffer, dNTPs, DNA polymerase I, and RNase H. And then, the double stranded cDNA was purified by AMPure P beads or QiaQuick PCR kit. The purified double-stranded cDNA was repaired at the end, added a tail and connected to the sequencing connector, then the fragment size was selected, and finally the final cDNA library was obtained by PCR enrichment.
We used FusionCatcher (Nicorici et al., 2014) software (https://github.com/ndaniel/fusioncatcher) to identify chimeric RNAs in human ROs. Positive chimeric RNAs identified using FusionCatcher were selected with alignment of spanning unique reads. The expression level of chimeric RNAs was reflected from log(Spanning_unique_reads/Total_number_of_reads*10,000,000) value. To analyze the expression of parental genes in human ROs, raw reads were first mapped to the hg38 human genome reference sequence by Hisat2 software, then transcripts were assembled with featureCounts. Metascape (https://metascape.org/gp/index.html) is used for functional annotation. The position weight matrix of the 20 bp DNA sequence motif around the fusion site was calculated by the seqLogo R package. GSEA analysis is done with the R package clusterProfiler.
Generation of ROs from hESCs
Request a detailed protocolThe CRX-tdTomato human ES reporter line and iPSCs were used for the ROs differentiation. The hESCs were cultured to 80% confluence. Cell colonies were dissociated using dispase buffer (Stem cell, 07923) for 5 min at 37°C and were then cut into smaller pieces/aggregates. The aggregates were collected and mixed with Matrigel (RD). The solidified gel with ES cells were dispersed into small pieces with medium containing 1:1 mixture of Dulbecco’s modified Eagle medium (DMEM)/F12, neurobasal medium, and 0.5× N2 supplement (Gibco), 0.5× B27 supplement (Gibco), 1× MEM Non-Essential Amino Acids Solution (Gibco), 2 mM Glutamax (Gibco), and 0.1 mM 2-mercaptoenthanol. At day 1 (D1), hollow cysts could be observed in the dish and cysts started to attach to the culture dish and spread in 3 days. At D5, the cysts were dispersed into two culture dishes with same medium. Medium was changed every 5 days. On D15, detaching the cells with dispase and change to medium with DMEM/F12 (3:1), 1× B27 supplement (Gibco), and 1× MEM-NEAA (Gibco) for a week. Optic vesicles formed in this period. Finally, optic vesicles were transfered to serum medium with DMEM/F12 (3:1), 1× B27 supplement (Gibco), 1× MEM-NEAA (Gibco), 8% fetal bovine serum (Gibco), 100 mM Taurine (Sigma-Aldrich), and 2 mM Glutamax (Gibco). ROs would be collected at D60.
CTCL knockdown in the ROs
Request a detailed protocolRNA interference oligo (5′-TGCTTGTTAACCTGGTCGA-3′) against CTCL was cloned into lentivirus vector (pLenti-U6-shRNA-EF1a-EGFP-T2A-Puro-WPRE). D12 retinal cells were infected with lentiviruses ( Multiplicity of infection [MOI] = 10, the ratio of the number of transducing lentiviral particles to the number of cells) for 6 hr, and replaced with fresh medium. The fluorescence was detected after 48 hr. The expression of CTCL, CTNNBIP1, and CLSTN1 in retinal cells was analyzed at D15 by quantitative PCR.
Immunostaining of cryosections
Request a detailed protocolROs were fixed in 4% paraformaldehyde for 30 min and imbedded in O.C.T. compound and sectioned into 10 μm slices. The cryosections were blocked with 0.5% Triton X-100 in 4% bovine serum albumin (BSA) for 1 hr. After that, sections were incubated in primary antibodies (diluted in 4% BSA supplied with 0.5% Triton X-100) at 4°C overnight. The following primary antibodies were used: anti-OTX2 (1:200, Cat.# ab183951; Abcam), anti-PAX6 (1:200, Cat.# 901301; Biolegend), anti-SOX2 (1:200, Cat.# sc-365823; Santa Cruz), anti-HuC/D (1:100, Cat.# A21271; Invitrogen), anti-MITF (1:100, Cat.# ab3201; Abcam), anti-GFAP (1:200, Cat.# sc-33673; Santa Cruz), anti-Sox9 (1:200, Cat.# 711048; Invitrogen), anti-RxRγ (1:100, Cat.# sc-365252; Santa Cruz), and anti-Ki67 (1:200, Cat.# ab15580; Abcam). After washed with phosphate-buffered saline, cryosections were stained with Alexa Fluor-conjugated secondary antibodies (diluted 1:500, Invitrogen) for 1 hr at room temperature in the dark.
Quantification and statistical analysis
Request a detailed protocolHeatmap was plotted with the R package Pheatmap. All plots were drawn with R package ggplot2. Detailed statistical analysis of experiments can be found in the figure legends, including the statistical tests used, exact values, and biological replicates.
Resource availability
Request a detailed protocolFurther information and requests for resources and reagents should be directed to and will be fulfilled by the lead contact, Professor Zi-Bing Jin (jinzb502@ccmu.edu.cn).
Materials availability
Request a detailed protocolAll unique/stable reagents generated in this study are available from the lead contact with a completed materials transfer agreement.
Data availability
Sequencing data has been deposited in GSA under accession code HRA005688 and may be accessed by application to a data access committee. The Gene Expression Omnibus (GEO) number for the bulk RNA-seq of organoids in this paper is GSE136929. All data generated or analyzed during this study is included in the manuscript and supporting files. Source data files have been provided for Figures 4C and 5A,B.
-
National Genomics Data CenterID HRA005688. CTCL in RO.
-
NCBI Gene Expression OmnibusID GSE136929. Human embryonic stem cell-derived organoidal retinoblastoma reveals cancerous origin and therapeutic target.
-
NCBI Gene Expression OmnibusID GSE155154. Human AMD.
References
-
Chimeric rna in cancer and stem cell differentiationStem Cells International 2018:3178789.https://doi.org/10.1155/2018/3178789
-
Functional architecture of the retina: development and diseaseProgress in Retinal and Eye Research 42:44–84.https://doi.org/10.1016/j.preteyeres.2014.06.003
-
TumorFusions: an integrative resource for cancer-associated transcript fusionsNucleic Acids Research 46:D1144–D1149.https://doi.org/10.1093/nar/gkx1018
-
Deep Sc-RNA sequencing decoding the molecular dynamic architecture of the human retinaScience China Life Sciences 66:496–515.https://doi.org/10.1007/s11427-021-2163-1
-
Stemming retinal regeneration with pluripotent stem cellsProgress in Retinal and Eye Research 69:38–56.https://doi.org/10.1016/j.preteyeres.2018.11.003
-
Role of the epithelial-mesenchymal transition and its effects on embryonic stem cellsExperimental & Molecular Medicine 46:e108.https://doi.org/10.1038/emm.2014.44
-
Transcriptomic profiling of human pluripotent stem cell-derived retinal pigment epithelium over timeGenomics, Proteomics & Bioinformatics 19:223–242.https://doi.org/10.1016/j.gpb.2020.08.002
-
Generation of human patient iPSC-derived retinal organoids to model retinitis pigmentosaJournal of Visualized Experiments 01:64045.https://doi.org/10.3791/64045
-
The emerging complexity of gene fusions in cancerNature Reviews Cancer 15:371–381.https://doi.org/10.1038/nrc3947
-
SoftwareR: A language and environment for statistical computing, version 4.0.3R Foundation for Statistical Computing, Vienna, Austria.
-
The landscape of chimeric RNAs in non-diseased tissues and cellsNucleic Acids Research 48:1764–1778.https://doi.org/10.1093/nar/gkz1223
-
Targeting KDM4 for treating PAX3-FOXO1-driven alveolar rhabdomyosarcomaScience Translational Medicine 14:eabq2096.https://doi.org/10.1126/scitranslmed.abq2096
-
Identification of chimeric RNAs in human infant brains and their implications in neural differentiationThe International Journal of Biochemistry & Cell Biology 111:19–26.https://doi.org/10.1016/j.biocel.2019.03.012
-
ggseqlogo: a versatile R package for drawing sequence logosBioinformatics 33:3645–3647.https://doi.org/10.1093/bioinformatics/btx469
Article and author information
Author details
Funding
National Natural Science Foundation of China (82125007)
- Zi-Bing Jin
The funders had no role in study design, data collection, and interpretation, or the decision to submit the work for publication.
Acknowledgements
This study was partially supported by grants from National Natural Science Foundation of China (82125007).
Ethics
One human donor retinal sample was included in this study. The study was approved by the Ethics Committee of Beijing Tongren Hospital (No. TRECKY2021-089) and conducted in accordance with the Declaration of Helsinki.
Copyright
© 2024, Wang, Zhang et al.
This article is distributed under the terms of the Creative Commons Attribution License, which permits unrestricted use and redistribution provided that the original author and source are credited.
Metrics
-
- 931
- views
-
- 253
- downloads
-
- 0
- citations
Views, downloads and citations are aggregated across all versions of this paper published by eLife.